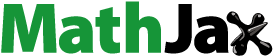
ABSTRACT
The following manuscript describes 10 newly synthesised pentafluorostilbene derivatives that are diverse in the character of the substituent attached to the second phenyl ring, as well as in the substitution site. The compounds were investigated for their applicability as iodonium salt photosensitisers for initiating cationic, radical and hybrid photopolymerisation. For this purpose, various spectroscopic studies were performed, such as absorbance and fluorescence quenching experiments. For kinetic research, Fourier transform infrared spectroscopy (real-time FT-IR) was utilised, which enabled analysis of the performance of the investigated two-component photoinitiating systems in real time. According to a variety of kinetic studies, the most effective initiator system was selected and applied for application studies in the subsequent stage, namely the preparation of photocurable polymer nanocomposites using light-initiated 3D printing technology (DLP – Digital Light Processing). The most effective two-component initiating system was tested in seven different photo-curable resins by thermogravimetry (TGA) and dynamic mechanical analysis (DMA) to demonstrate its applicability in 3D printing of nanocomposites.
Introduction
Photoinduced polymerisation is a photochemically initiated process utilising a suitable light source emitting electromagnetic radiation in the ultraviolet (UV) to visible (VIS) range [Citation1–3.] Photopolymerisation processes are now widespread and rapidly developing with technologies related to the moulding of three-dimensional models employing light-initiated 3D printing [Citation4–9] and the printing industry, where they are commonly used for photo-curing UV varnishes and inks [Citation10–13].
The chemical compounds applied as photoinitiators should comply with a number of requirements [Citation14, Citation15]. Absorption characteristics are the most important parameter with a direct impact primarily on the photopolymerisation efficiency. A photoinitiator should absorb all or most of the light radiation emitted by industrial light sources. Currently, much attention is being paid to the design of new compounds that will absorb radiation in the UV-A range or even visible light, making it possible to use these compounds for the role of photosensitisers in polymerisation processes. An innovative approach is the use of pentaflurostilbene derivatives for the role of photosensitisers in two-component initiator systems.
Stilbene derivatives have been the focus of many research groups for several years [Citation16–20]. Stilbene compounds are characterised by good spectroscopic properties, hence they play an important role in photochemistry [Citation21–26] and medicine [Citation27–32]. Owing to their spectroscopic properties, they have found applications as optical brighteners, which absorb radiation in the near ultraviolet range while simultaneously emitting radiation in the blue range of the visible spectrum, making colours appear more distinct [Citation33, Citation34]. Thanks to this feature, substances based on stilbene derivatives are widely applied as optical brighteners in the textile and paper industries. Stilbene derivatives, however, are used primarily in medicine and the cosmetic industry [Citation35–37]. This is because stilbenoids have antioxidant [Citation38, Citation39], anticancerogenic [Citation40–43] and anti-allergic properties [Citation44–46]. However, their potential utilisation is not limited to biomedical applications. Due to their interesting spectroscopic properties, stilbene derivatives have been used over the past few years in the construction of efficient organic light-emitting diodes [Citation47–49], as well as solar cells [Citation50–52].
An interesting application of stilbene derivatives containing fluorine atoms in their structure was described by Neckers, et al. [Citation53]. The compounds presented in their work were investigated as potential molecular fluorescent probes for monitoring photopolymerisation processes, emphasising their suitability for cationic photopolymerisation. The compounds trans-2-(2’,5′-dimethoxyphenyl)ethyl-2,3,4,5,6-pentafluoro-benzene and trans-2-(3′,4′,5′-trimethoxyphenyl)ethyl-2,3,4,5,6-pentafluorobenzene absorb mainly in the UV range. Moreover, they show little sensitivity to changes in solution polarity, thus, in a limited way, they can be used as fluorescent molecular sensors for monitoring the polymerisation kinetics. Nevertheless, other stilbene derivatives with a more expanded structure such as trans,trans-1,4-bis[2-(2’,5'-dimethoxy)phenylethyl)-2,3,5,6-tetrafluorobenzene and trans,trans-1,4-bis[2-(3’,4’,5'-trimethoxy)phenyl)-2,3,5,6-tetrafluorobenzene provided higher fluorescence quantum yields. Based on the study, it was shown that among all four compounds, trans,trans-1,4-bis[2-(2’,5'-dimethoxy)phenylethyl)-2,3,5,6-tetrafluorobenzene exhibits the highest sensitivity to environmental changes, so it is best suited for monitoring the changes occurring during cationic and free radical photopolymerisation.
In 2010, the spectroscopic properties of eight new stilbene derivatives with various electron-donor and electron-acceptor substituents were described. The stilbene derivatives presented in this paper were investigated as potential fluorescent sensors for monitoring cationic photopolymerisation processes. Fluorescence Probe Technology (FPT) was used to monitor the photopolymerisation process, the idea of which is to measure changes in fluorescence characteristics under the influence of changes in environmental properties [Citation54–59]. The study showed that stilbene derivatives with electron-acceptor groupings exhibit greater sensitivity to changes in the surrounding environment than derivatives containing electron-donor substituents. It was concluded that all stilbene derivatives could find application as fluorescent probes for monitoring the course of cationic photopolymerisation. It was also found that 3,4-(methylenedioxy)-4'-cyanostilbene showed the highest sensitivity to environmental changes among the tested compounds.
One really interesting application of stilbenes is their utilisation for the role of photosensitisers in a two-component initiator system. There are some reports in the literature concerning this issue [Citation60]. The present work concerns the study of pentafluorostilbene derivatives equipped with amine functional groups. Such design ensures desired properties that improving their photosensitising activity. The pentaflurobenzene (electron-acceptor) in combination with amine groups (electron-donor) strongly red-shifting absorption of stilbene core by push–pull effect (D-π-A structure). The amine groups ensure proper reducing properties allowing efficient generation of radicals and superacid in combination with iodonium salts. Both effects were obtained in a relatively small structure which should additionally have a favourable effect on the solubility of these compounds and thus their overall performance.
In this paper, we investigated the suitability of 10 1,2,3,4,5-pentafluoro-6-[(E)-styryl]benzene derivatives to behave as potential photosensitisers of the diphenyliodonium salt IOD to initiate radical, cationic and hybrid photopolymerisation processes applying light sources in the ultraviolet and visible range. The next step was to verify the possibility of obtaining photo-curable polymer nanocomposites using 3D printing technology, where a two-component initiator system (pentafluorostilbene derivative + IOD iodonium salt) was utilised as an initiator.
Experimental
Nine derivatives of 1,2,3,4,5-pentafluoro-6-[(E)-styryl]benzene, differentiated by the type of amine substituent located in the second phenyl ring and the substitution position of the amine moiety in the phenyl ring were investigated for the role of diphenyliodonium salt photosensitisers for efficient initiation of different types of photopolymerisation process. This group included the following compounds: 2-[(E)-2-(2,3,4,5,6-pentafluorophenyl)vinyl]-N-phenylaniline (M2), 3-[(E)-2-(2,3,4,5,6-pentafluorophenyl)vinyl]-N-phenylaniline (M3), 4-[(E)-2-(2,3,4,5,6-pentafluorophenyl)vinyl]-N-phenylaniline (M4), N-methyl-2-[(E)-2-(2,3,4,5,6-pentafluorophenyl)vinyl]-N-phenylaniline (M5), N-metylo-3-[(E)-2-(2,3,4,5,6-pentafluorophenyl)vinyl]-N-phenylaniline (M6), N-metylo-4-[(E)-2-(2,3,4,5,6-pentafluorophenyl)vinyl]-N-phenylaniline (M7), 3-[(E)-2-(2,3,4,5,6-pentafluorophenyl)vinyl]-N,N-diphenylaniline (M8), 2-[(E)-2-(2,3,4,5,6-pentafluorophenyl)vinyl]-N,N-diphenylaniline (M9), 4-[(E)-2-(2,3,4,5,6-pentafluorophenyl)vinyl]-N,N-diphenylaniline (M10). 1,2,3,4,5-pentafluoro-6-[(E)-styryl]benzene (M1) was used as a reference compound. The structures of the analysed compounds are shown in . Stilbene derivative structures were confirmed by nuclear magnetic resonance spectroscopy – 1H NMR (Figures S.1-S.10).
The following monomers were employed to develop the photo-curable formulations: 3,4-epoxycyclohexylmethyl-3,4-epoxycyclohexane-carboxylate (CADE, Lambson Ltd), trimethylolpropane triacrylate (TMPTA, Sigma Aldrich), Ebecryl 4858 (Aliphatic urethane diacrylate, Allnex), Ebecryl 130 (aliphatic difunctional acrylate, Allnex), Ebecryl 4740 (aliphatic allophanate urethane acrylate, Allnex), Ebecryl 45 (tetrafunctional polyether acrylate, Allnex), Ebecryl 1291 (aliphatic urethane hexaacrylate, Allnex), Isobornyl acrylate (Photomer 4012, IGM Resins). Bis-(4-t-butylphenyl)iodonium hexafluorophosphate (IOD, Lambson Ltd.) was applied as a reference commercial photoinitiator. Zinc oxide (ZnO, Sigma Aldrich, 20 nm nanoparticles size) was used as nano-additives to obtain photo-curable polymer nanocomposites. The structures of the applied monomers and initiator are shown in Supplementary Material (Figure S.11).
Spectroscopic measurements
⇒ Absorbance measurements
The SilverNova® TEC miniature spectrometer (StellarNet Inc., USA) equipped with a CCD array for the spectral range of 190 nm–1100 nm was employed to examine absorbance. Appropriately prepared solutions of stilbene derivatives with a concentration of 7.4·10−5 mol/dm3 in acetonitrile were placed in a quartz cuvette with an optical path length of 1 cm. A SL5 deuterium-halogen lamp manufactured by StellarNet Inc. was employed as the light source for absorbance measurements.
⇒ Measurements of emission and excitation spectra and fluorescence quenching
Emission and excitation spectra and fluorescence quenching measurements were carried out on a Quanta MasterTM 40 spectrofluorimeter (Photon Technology International, Canada) using a xenon lamp manufactured by the Photon Institute of Krakow. The test solutions were placed in a quartz cuvette with an optical path length of 1 cm. The amount of added iodonium salt serving as a fluorescence quencher ranged from 2 to 60 mg.
Electrochemical investigation of oxidation and reduction potentials
Voltammetric measurements were carried out to determine the values of oxidation and reduction potentials of the investigated pentafluorostilbene derivatives. The electrochemical analyser M161 and electrode stand M164 (from MTM-ANKO, Poland) with a standard three-electrode cell, were used. During the measurement, tetrabutylammonium hexafluorophosphate (1 M) (Sigma Aldrich) was applied as the basic electrolyte, a platinum plate was utilised as the working electrode, and a silver electrode (Ag/AgCl) was used as the reference electrode. Voltammetry was recorded at a scan rate of 100 mV/s, using ferrocene as a standard, and potentials were determined from the mid-peak potentials.
Photopolymerisation kinetics measurements
Fourier transform infrared spectroscopy was employed to determine kinetic profiles. The apparatus consists of a NICOLETTM iSTM 10 spectrometer made by Thermo Fisher Scientific and a special horizontal attachment dedicated to kinetic measurements. Various UV-LED: λmax = 365 nm (M365L2 Thorlabs, the light intensity on the photopolymerised sample was 5.29 mW/cm2) and Vis-LEDs were applied as light sources: λmax = 405 nm (M405L3 Thorlabs, the light intensity on the photopolymerising sample was 6.53 mW/cm2), λmax = 420 nm (M420L3 Thorlabs, the light intensity on the photopolymerising sample was 7.29 mW/cm2). Photopolymerisation measurement spectra were recorded in OMNIC software. The measurements were performed in a dimmed room, the temperature during the experiments was 25°C. The distance of the fibre end from the sample surface was 2.1 cm. The conversion rate of acrylate and epoxy groups was determined based on the following formula [Citation61]:
Where:
C – conversion of functional groups.
A – absorbance determined as an area of the specific functional group’s absorption band with a maximum at 790 cm−1 for epoxies (the oscillation of oxirane ring) [Citation62] or 1635 cm−1 (acrylic C = C bond oscillation) and 6165 cm−1 for acrylates ( = C–H bond oscillation) [Citation63,Citation64].
Photocurable resins for kinetics measurements and 3D printing experiments were prepared by dissolving the initiator system (IOD/stilbene derivative 1.0/0.1% w/w) in an epoxy monomer for cationic photopolymerisation, in an acrylate monomer for radical photopolymerisation.
3D-VAT printing experiments
For 3D printing experiments, three different models were designed in Fusion 360 software (AutoDesk) in stereographic (.stl) format. The first model ((a)) was utilised to determine the optical resolution of the 3D printed objects, as well as the effect of nano-additives on the print quality. Subsequently, (3 × 5 × 50) mm3 beams ((b)) and (10 × 10 × 1) mm3 slices ((c)) were printed as testing specimens for DMA and TGA, respectively. The three-dimensional objects were printed by a Lumen X + TM printer (CellInk Inc.) equipped with a projector emitting at 405 nm with a light irradiance on the printed surface of 1.99–9.95 mW/cm2.
Figure 2. 3D printing models employed for DLP technology: (a) study of the resolution of the prepared resins, (b) DMA measurements, (c) TGA measurements.
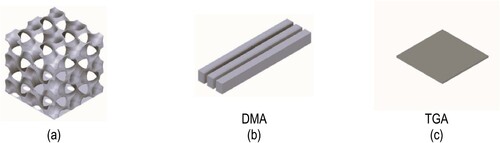
Determination of 3D printing parameters
Jacob’s working curves test, which involved printing slices (1 × 1 cm) at different times without applying a printing platform, allowed the determination of basic 3D printing parameters such as the critical energy Ec, among others. The following formula [Citation65] was employed to determine the parameters:
Where:
Cd – thickness of the cured layer [µm]; Dp – depth penetration [µm]; E0 – light energy at the surface [mJ/cm2].
The printed slices were first cleaned of the uncured resin, then their thickness was measured at five different locations with the use of a micrometer screw and the averaged thickness was determined. Based on the quotient of the curing time of each slice and the intensity of light incident on the printed slice (measured with a light intensity metre from ThorLabs PM 160 Optical Power Meter), E0 (light energy at the surface [mJ/cm2]) was calculated. The averaged Cd value was plotted on a logarithmic graph as Cd = f(E0) to define Dp as the slope of the working curve and Ec as its intersection with the x-axis.
Optical microscopy
OLYMPUS DSX1000 optical microscope was employed for optical imaging of 3D prints aimed at qualitative evaluation of the obtained nanocomposites. The optical visualisation allowed verification of the real size of the resulting printouts (compared to the designed 3D model).
SEM analysis
A high-resolution scanning electron microscope Apreo 2 S LoVac (Thermo Fisher Scientific) equipped with X-ray energy dispersive spectrometer (EDS) detectors UltraDry (Thermo Fisher Scientific) and Octane Elect (EDAX Ametek GmbH) was used to analyse the surface quality of the resulting prints. The samples were coated with a thin layer of gold and imaging was performed at an accelerating voltage of 2.0 kV and an electron beam current of 0.4 nA.
Dynamic mechanical analysis (DMA) measurements
Thermomechanical properties of the printed specimens were evaluated by RSA-G2 (TA Instruments, USA) using a hybrid dynamic mechanical analysis (DMA) combined with a heat deflection temperature measurement (HDT) [Citation8]. The testing specimens printed according to (b) were measured in a 3-point bending setup. The oscillatory frequency was 1 Hz, the oscillatory strain of 0.002% was superimposed with a small static stress of 0.455 MPa. A temperature ramp test was performed from –30°C to 160°C at a controlled heating rate of 2°C/min. The glass transition temperature (Tg) was determined at the maximum loss tangent (tan δ = E′′/E′), where E’ and E” represent storage and loss moduli, respectively. Heat deflection temperature (HDT) was determined at flexural strain ϵf of 0.195%, corresponding to deflection s of about 0.25 mm according to equation:
(6)
(6) where h is the specimen’s thickness, and L is the geometry span (40 mm). The network density (υe) was determined by measuring the modulus in the rubbery region at temperatures above Tg according to equation:
(7)
(7) where
is the Poisson’s ratio (
= 0.5 for incompressible material),
is the rubber-plateau modulus, T is the thermodynamic temperature, and R is the gas constant.
Thermogravimetric analysis measurements
Thermogravimetric analysis was performed by Discovery TGA (TA Instruments, USA) as a controlled increase of temperature to 700°C at a heating rate of 10°C/min in a non-oxidative nitrogen atmosphere. The samples with a typical size of around 10 mg were collected from the slices printed according to (c). A differential thermogravimetric signal was obtained as the first derivative of the residual weight signal in the TRIOS OEM software.
Results and discussion
Spectroscopic properties
The investigated 1,2,3,4,5-pentafluoro-6-[(E)-styryl]benzene derivatives could be divided into two groups according to the type of their substituent. Compounds containing phenylamine substituent (M2, M3, M4) represent secondary amines while compounds with phenylmethylamine (M5, M6, M7) or diphenylamine (M8, M9, M10) moieties can be classified as tertiary amines. Their spectroscopic properties were analysed by measuring the absorbance of their acetonitrile solutions. The M1 reference showed maximum absorbance at 293 nm and negligible absorption above ∼340 nm (). On the contrary, the tested derivatives absorbed up to ∼420–450 nm. While the amine substituents at the ortho- (M2, M5, M8) and meta- (M3, M6, M9) positions of the second phenyl ring gave rise to a second weaker absorption band at ∼350–370 nm, para-substituted derivatives (M4, M7, M10) shifted the main peak towards higher wavelengths ().
The highest molar extinction coefficients in the UV-A and visible range among the tested compounds scaled in the order M10 (ϵ@λmax-AB = 28569 dm3mol−1cm−1 at 375 nm) > M4 (ϵ@λmax-AB = 27920 dm3mol−1cm−1 at 362 nm) > M7 (ϵ@λmax-AB = 24023 dm3mol−1cm−1 at 366 nm), rendering the para-substituted derivatives as the most promising candidates for use in VIS-range photoinitiating systems. The compound 4-[(E)−2-(2,3,4,5,6-pentafluorophenyl)vinyl]-N,N-diphenylaniline (M10) featured the best spectroscopic properties because its maximum absorbance was relatively high and located at rather long wavelength, allowing it to absorb light up to 450 nm (). The strong electron-donor character of its diphenylamine substituent had the highest absorbance also in the meta- (M9 > M3 > M6) position, but the smaller phenylamine moiety was more beneficial in the ortho-position (M2 > M8 > M5) (). Moreover, tertiary amines show a slight shift in the absorption spectrum towards longer wavelengths relative to secondary amines. The reference compound M1, in contrast to the other investigated pentafluorostilbene derivatives, has a negligible band above 325 nm. This is caused by electronic effects. All derivatives with amine groups have a more polarised structure – the pentafluorobenzene is electron-acceptor. In their case, the band in the visible range, corresponds to the intramolecular charge transfer (ICT) transition originates from push–pull effect of electron donating and accepting groups composition in prepared structures. The more polarised the structure, the less energy is needed to excite the molecule [Citation66]. In more, the substituent site affects the intensity of the absorption band in the visible range, for compounds with a substituent in the para position (M4, M7, M10) a hyperchromic effect can be observed with reference to compounds with a substituent in the ortho and meta positions. This effect is due to the preservation of the structure of conjugated bonds also in the excited state of the molecule when the substituents are in para positions relative to each other. In the case of ortho positions, the structure is also preserved. Unfortunately, the probability of excitation decreases with increasing steric hindrance due to the size of the substituent in this position.
shows a summary of the spectroscopic properties of the studied stilbene derivatives: molar extinction coefficients for wavelengths of λ = 365 nm, λ = 405 nm, λ = 420 nm (ϵ), wavelengths corresponding to the absorption maximum for the longest wavelength band (λmax-ab), molar extinction coefficients at λmax-ab (ϵ@λmax-ab).
Table 1. Spectroscopic characterisation of 1,2,3,4,5-pentafluoro-6-[(E)-styryl]benzene derivatives.
Activity mechanism of two-component photocatalytic systems
Photosensitising employs light quanta to initiate the process of electron transfer from a donor molecule to an acceptor molecule. The electron transfer process includes a two-component initiator system in which the radiation absorbing molecule (photosensitiser) is excited and becomes the electron donor which is oxidised, while the other molecule acting as the electron acceptor is reduced. The electron transfer between the two different components is characterised by the change in Gibbs free energy (ΔGet), which must be negative to thermodynamically permit the electron transfer. That sets up a second general requirement on effective photosensitisers next to their good spectroscopic properties. All studied pentafluorostilbene derivatives have a negative ΔGet value, which suggests that they can effectively initiate the photopolymerisation process. The calculated values of (ΔGet) for the studied photoinitiating systems oscillate in the range between −1.50 and −1.05 eV for the singlet excited state.
The oxidation potentials of the investigated 1,2,3,4,5-pentafluoro-6-[(E)-styryl]benzene derivatives were determined by cyclic voltammetry. The lowest values of oxidation potential, which is a desirable feature of photosensitisers in the two-component initiator systems, were obtained for molecules with substituents in the para position, namely M4, M7 and M10. The sensitisers’ singlet state energies (E00) were determined from excitation and emission spectra. Negative Gibbs free energies confirmed the ability of the spontaneous electron transfer between iodonium salt and stilbene derivatives. Thus, they can be used as a two-component photoinitiating system while the stilbene derivatives act as photosensitisers. They efficiently initiate cationic, radical, thiol-ene, as well as hybrid photopolymerisation processes as will be demonstrated later.
Fluorescence quenching measurements were carried out subsequently. Increasing the amount of the iodonium salt as a quencher caused a linear decrease in stilbene derivatives’ fluorescence intensity. On this basis, the Stern–Volmer relationship was determined and the Stern–Volmer coefficient (KSV) was determined. Fluorescence quenching also provided information on the electron transfer rate constant (ket) between the diphenyliodonium salt and the photosensitiser in the excited state. All calculated and measured values related to the electron transfer process are shown in . In turn, all the formulas that were employed in the calculations are included in the Supplement (Figures S.12-S.59).
Table 2. Electrochemical and thermodynamic properties of 1,2,3,4,5-pentafluoro-6-[(E)-styryl]benzene derivatives.
Photosensitising properties
⇒ Cationic photopolymerisation with epoxy ring opening
Cationic photopolymerisation processes are widespread in industrial applications because they have several key advantages determining their practical applicability. Cationic photopolymerisation is characterised by a living character [Citation67], which means that the reaction proceeds efficiently even after the radiation source is turned off (dark polymerisation), enabling a high degree of reactivity to be achieved. For this reason, nowadays photoinitiated cationic polymerisation is playing an increasingly important role in global economies as a method for obtaining photo-curable polymer coatings [Citation68]. The dynamic progress in the development of this type of photopolymerisation is also due to the wide range of choices of monomers that polymerise according to the cationic mechanism. For the cationic photopolymerisation process, cycloaliphatic epoxy resins or vinyl ethers are usually employed as monomers [Citation69–71].
The first step in investigating the suitability of 1,2,3,4,5-pentafluoro-6-[(E)-styryl]benzene derivatives to function as photosensitisers of iodonium salts for initiating the photopolymerisation process was to monitor the cationic photopolymerisation of the epoxy monomer. For this purpose, compositions consisting of the iodonium salt IOD, the corresponding stilbene derivative (1/0.1% w/w) and the epoxy monomer CADE were prepared. The photopolymerisation process took 800 s. The measurement was performed on a drop of photopolymerising epoxy resin on a BaF2 pastille (polymerisation in air). The FT-IR spectra before and after cationic photopolymerisation are shown in . During the process, the epoxy ring, which is present in the CADE epoxy monomer, breaks down, which can be seen on the FT-IR spectrum. The decreasing intensity of the peak located at a wavenumber of 790 cm−1 indicates the disappearance of epoxy groups during the process, which is associated with the formation of ethers bonds. That is evidenced by the increasing intensity of the band at 1080 cm−1.
Figure 4. FT-IR spectrum changes for CADE epoxy monomer-based composition during cationic photopolymerisation under 365 nm UV-LED irradiation, employing IOD + M10 (1.0/0.1% w/w) initiator system.
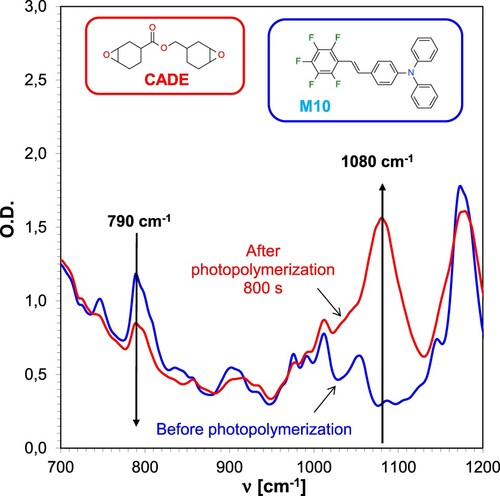
For the cationic photopolymerisation, conversions were calculated based on the disappearance of the band corresponding to the oxirane ring. Initially, measurements were carried out employing an ultraviolet light source emitting radiation with a wavelength of λ = 365 nm. The obtained conversions of the epoxy monomer ranged between 41 and 69%. The reference compound M1 does not show a sensitising effect due to its low molar extinction coefficient of 200 [dm3mol−1cm−1] at the wavelength of 365 nm, as well as a relatively high value of the oxidation potential. The highest conversions of oxirane groups were reached for resins that contained the photosensitisers M9 and M10, which is related to the presence of the electron-donor substituent in the para position. A formulation with IOD iodonium salt as the initiator of the photopolymerisation process was employed as the reference resin. The kinetic profiles obtained are shown in . Analogous measurements were conducted by applying light sources in the visible range of λ = 405 and λ = 420 nm. The kinetic profiles obtained are provided in the ESI (Figure S.60).
Figure 5. Kinetic profiles obtained during the cationic photopolymerisation of the epoxy monomer CADE for two-component systems consisted of the IOD iodonium salt and the appropriate 1,2,3,4,5-pentafluoro-6-[(E)-styryl]benzene derivative (1.0/0.1% w/w) during irradiation with a UV-LED emitting a wavelength of 365 nm.
![Figure 5. Kinetic profiles obtained during the cationic photopolymerisation of the epoxy monomer CADE for two-component systems consisted of the IOD iodonium salt and the appropriate 1,2,3,4,5-pentafluoro-6-[(E)-styryl]benzene derivative (1.0/0.1% w/w) during irradiation with a UV-LED emitting a wavelength of 365 nm.](/cms/asset/3d4b4192-7de7-42c5-be86-9e96443db186/nvpp_a_2301030_f0005_oc.jpg)
demonstrates the conversion rates of epoxy monomer during cationic photopolymerisation depending on the light source employed to initiate the process.
Table 3. The obtained conversion rates of CADE epoxy monomer during the cationic photopolymerisation process applying light sources in the UV-Vis range.
The effectiveness of potential photosensitisers in a two-component initiator system is not determined only by the degree of monomer over-reactivity achieved. An extremely important parameter that guarantees the effectiveness of the system is also the induction time, which tells about the time required to initiate the growth of the polymer chain. Another equally important factor is the slope of the kinetic curve, which tells about the speed of process initiation. presents the values of the induction times and the slopes of the kinetic curves for the profiles obtained during the cationic photopolymerisation of the epoxy monomer CADE applying UV- and Vis-LED light sources (LED@365 nm, LED@405 nm, LED@420 nm).
Table 4. The obtained values of induction time and kinetic curve slope during the process of cationic photopolymerisation initiated by UV- and Vis-LED light sources.
Analysis of the effect of the nature of the amino group current in the structure of the 1,2,3,4,5-pentafluoro-6-[(E)-styryl]benzene derivative on the photosensitisation of the photopolymerisation process showed that the diphenylamine substituent in M10 compound yielded the highest degrees of epoxy monomer conversion compared to the other tested substituents. However, the compound M8 with the diphenylamine group in ortho- position achieved only a low monomer conversion. That may be caused by the M8’s high oxidation potential which is not desirable for the photosensitisation process. On the other hand, secondary amine derivatives (M2, M3, M4), provided a very fast photopolymerisation process, which was confirmed by obtaining very short induction times and high values of the slope of the kinetic curves. Meanwhile, the induction time for initiator systems containing tertiary amines with the -NPh(CH3) substituent is slightly increased compared to secondary amines, while the kinetic curves reach smaller conversion values. Two effects may affect the profiles of photopolymerisation the -NPh(CH3) substituent ensures lower molar absorption coefficients. Such photosensitisers absorb less light allowing it to penetrate the sample more. The second effect may be caused by the lowest oxidation potential compared to secondary amines ensure by alkyl substituent. It increase electron density in amine substituent and its neighbourhood, increasing the reduction rate of iodonium salt. shows the effect of the amine substituent nature on the resulting epoxy monomer conversion rates.
Figure 6. Effect of the amine group character present in the photosensitiser structure on the degree of CADE monomer conversion (light source LED@365 nm).
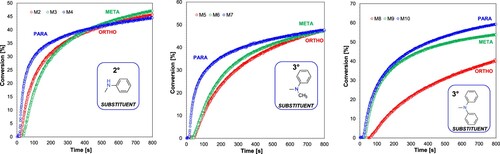
Analysis of the effect of the substitution location of the amino group in the structure of the sensitiser () on the photopolymerisation process showed that the process occurs most rapidly for binary systems containing a photosensitiser with an amino substituent located in para position to one of the phenyl rings found in the stilbene systems analysed. The photopolymerisation process initiated with these systems is characterised by a very short induction time. This is caused by the best molar absorption coefficient and lowest oxidation potentials for para derivatives. Both factors ensure that the necessary amount of light is absorbed as quickly as possible and this energy is transferred to the iodonium salts as efficiently as possible. This guarantees the fastest generation of the required amount of superacid necessary to initiate the polymerisation process and thus shortens the induction time of this process.
Figure 7. Influence of the substitution location of the amino group in the photosensitiser on the conversion rate of the CADE monomer (light source LED@365 nm).
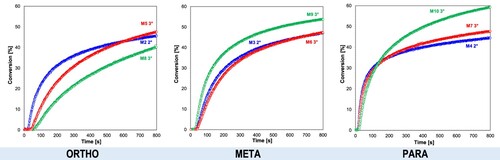
Subsequently, the cationic ring-opening photopolymerisation of the epoxy ring was conducted under visible light using Vis-LEDs emitting radiation at λ = 405 nm and λ = 420 nm. Similarly to ultraviolet light, compound M1 also presented no utility as a photosensitiser of iodonium salts under visible light. Measurements involving a 405 nm emitting diode demonstrated that the highest conversion rates were obtained in two-component systems with photosensitisers such as: M10 (4-[(E)−2-(2,3,4,5,6-pentafluorophenyl)vinyl]-N,N-diphenylaniline) and M4 (4-[(E)−2-(2,3,4,5,6-pentafluorophenyl)vinyl]-N-phenylaniline), due to the presence of an amine substituent at the para position and their highest molar extinction coefficients at these wavelengths (, ).
⇒ Radical photopolymerisation of acrylate monomer
Nowadays, radical photopolymerisation is the most widely applied way to produce polymeric materials by photochemical processes. The classical radical polymerisation process consists of 3 stages: initiation, propagation and termination. There are many means that can initiate this process, such as elevated temperature, ultraviolet or visible light, microwave radiation, ultrasound, chemical agent, or mechanical stress [Citation72–74].
Subsequent investigation of the applicability of 1,2,3,4,5-pentafluoro-6-[(E)-styryl]benzene derivatives for the role of sensitisers of commercially available iodonium salts was carried out by monitoring the progress of radical photopolymerisation of the acrylate monomer TMPTA. The studies were performed for two-component systems consisting of the iodonium salt IOD and stilbene photosensitisers (1.0/0.1% w/w). The measurement consisted of inserting a drop of photopolymerising acrylate resin between two pieces of polypropylene film to avoid oxygen inhibition during the measurement process. The progress of the photopolymerisation process was monitored based on the disappearance of the band, the maximum of which is located at a wave number of 1634 cm−1, corresponding to double bonds. shows the changes in the FT-IR spectrum during the radical photopolymerisation of the acrylate monomer.
Figure 9. Changes in the FT-IR spectrum for an acrylate monomer-based TMPTA composition during radical photopolymerisation under 365 nm UV-LED irradiation, employing the IOD + M10 (1/0.1% w/w) initiator system.
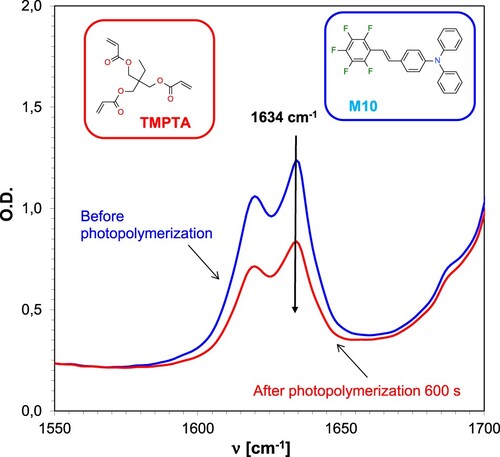
Ultraviolet light measurements demonstrated that all the investigated bimolecular systems could initiate the radical photopolymerisation of the acrylate monomer TMPTA. The lowest degree of reactivity was achieved by a two-component initiating system with M1 (1,2,3,4,5-pentafluoro-6-[(E)-styryl]benzene) as the photosensitiser. This system is the least efficient because compound M1 has a low molar extinction coefficient at λ = 365 nm and has a high oxidation potential value (1771mV) compared to the other stilbene derivatives. The highest conversion is characterised by the system based on compound M10, the conversion for this system is 43%. A formulation with IOD iodonium salt as the initiator of the photopolymerisation process was employed as the reference resin. The kinetic profiles obtained are shown in . Analogous measurements were performed using a diode emitting λ = 405 nm radiation. The obtained kinetic profiles are shown in the supplement (Figure S.61).
Figure 10. Kinetic profiles achieved during the progress of radical photopolymerisation of acrylate monomer TMPTA for two-component systems consisting of the IOD iodonium salt and the corresponding 1,2,3,4,5-pentafluoro-6-[(E)-styryl]benzene derivative (1.0/0.1% w/w) during irradiation with a UV-LED emitting a wavelength of 365 nm.
![Figure 10. Kinetic profiles achieved during the progress of radical photopolymerisation of acrylate monomer TMPTA for two-component systems consisting of the IOD iodonium salt and the corresponding 1,2,3,4,5-pentafluoro-6-[(E)-styryl]benzene derivative (1.0/0.1% w/w) during irradiation with a UV-LED emitting a wavelength of 365 nm.](/cms/asset/cf46a3a3-e8d1-41e3-a535-196ceae92945/nvpp_a_2301030_f0010_oc.jpg)
Sensitising measurements carried out under visible light using a Vis-LED emitting radiation at λ = 405 nm proved that the system based on compound M1 (1,2,3,4,5-pentafluoro-6-[(E)-styryl]benzene) does not exhibit a sensitising effect, which may be due to the poor spectroscopic properties of compound M1 in the visible light range. In contrast, the highest degree of monomer conversion under UV light was 44%, which was achieved with the M10 sensitiser. shows the degrees of monomer conversion during the progress of radical photopolymerisation initiated by UV- and Vis-LED light sources.
⇒ Hybrid photopolymerisation of epoxy and acrylate monomers
Table 5. The obtained conversion rates of acrylate monomer TMPTA, the values of the induction time and the slope of the kinetic curve during the progress of radical photopolymerisation initiated by UV- and Vis-LED light sources.
The most recent issue of light-induced polymerisation is hybrid polymerisation, which combines the advantages of different photopolymerisation processes because it integrates different polymerisation mechanisms or different types of materials [Citation75–77]. Among hybrid photopolymerisation systems, there are two main types. Hybrid system type I – composed of a mixture of monomers polymerising according to different mechanisms, i.e. radical and cationic, employing two different initiators dedicated to a specific polymerisation reaction. Such a composition makes it possible to obtain polymer networks, so-called interpenetrating polymer networks (IPN) [Citation78, Citation79], which are formed by simultaneous polymerisation of two multifunctional monomers. Hybrid system type II – composed of a mixture of different types of materials (e.g.: chain-polymerising vinyl monomer and cycloaliphatic ring-opening cationic epoxy monomer), the common polymerisation of which follows the same mechanism, so a single photoinitiator is involved. Due to the fact that hybrid photopolymerisation processes can be a combination of two different types of polymerisation, namely cationic and free radical polymerisation, thus this type of polymerisation is applied to obtain Interpenetrating Polymer Network (IPN). Generally, the obtaining of IPN-type polymers is aimed at providing key features of one polymerising component, while preserving the properties of the other component. In some instances, it is possible to obtain cross-linked polymeric materials with completely new and different properties compared to the initial monomers. The essential characteristics of photocrosslinked IPN systems (i.e. morphology, cross-linking density, degree of phase separation, size and shape of microdomains in the network) can be controlled by the intensity of UV light or the type and concentration of photoinitiator. Therefore, IPN-type systems are currently of great interest due to their enormous potential applications. Indeed, they can be used, for example, as sound and vibration-damping materials, in impact-resistant materials, as hardened plastics, membranes, ion-exchange resins. They can also find applications in electrical insulation, coatings, encapsulating substances, and drug carriers.
The final stage of the kinetic research was to monitor the progress of the hybrid photopolymerisation of the cationic monomer CADE and the radical TMPTA. For each composition, two measurements were carried out, one of which involved placing a drop of the test composition between two pieces of propylene film (laminate condition), while in the second the drop was placed on a pellet (air condition). With these two methods, it was possible to investigate the effect of the presence of atmospheric oxygen on the monomer conversion rates obtained.
During the measurements, bands originating from the two monomers could be observed. As in the case of cationic polymerisation, a decrease in the intensity of the band located at 790 cm−1 was observed during hybrid polymerisation, as well as an increase in the intensity of the band at a wavenumber of 1080 cm−1. In contrast, the band at a wavenumber of 1634 cm−1 originating from the double bond characteristic of the TMPTA monomer showed a rapid decrease during the progress of radical photopolymerisation, as shown in .
Figure 11. Changes in the FT-IR spectrum for resins based on CADE epoxy monomer and TMPTA acrylate monomer during hybrid photopolymerisation under 365 nm UV-LED irradiation using IOD + M9 (1.0/0.1% w/w) initiator system.
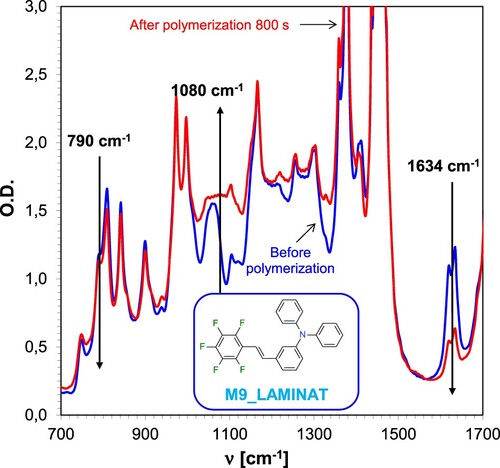
The resulting TMPTA acrylate monomer conversions are significantly higher for measurements made between two pieces of propylene film than for measurements on the pellet. The lower TMPTA radical monomer conversion rates for measurements on the pellet are due to the presence of oxygen, which causes the radical photopolymerisation process to stop or slow down. In contrast, in the case of the epoxy monomer, the situation is the contrary. High conversions of the epoxy monomer were obtained during measurements on the pellet, which confirmed the absence of oxygen inhibition during the cationic photopolymerisation process. During the measurements carried out in the laminate, higher conversions were obtained for the TMPTA monomer than for the epoxy monomer, because the acrylate monomer polymerises much faster than the epoxy monomer. The experiments showed that all investigated stilbene derivatives are effective initiating components of photoinitiating systems for the cationic photopolymerisation process, thus demonstrating their suitability for the role of photosensitisers of IOD iodonium salt. shows the kinetic profiles obtained during the course of hybrid photopolymerisation of acrylate monomer TMPTA and epoxy monomer CADE for a composition containing the photocatalytic system IOD + M10 (1.0/0.1% w/w). The kinetic profiles obtained during the hybrid photopolymerisation process for the other photocatalytic systems are presented in the Supplementary Materials (Figures S.62-S.70).
Figure 12. Kinetic profiles obtained during the photopolymerisation process of the hybrid acrylate monomer TMPTA and the epoxy monomer CADE for the two-component system consisting of the iodonium salt IOD/M10 (1.0/0.1% w/w) during irradiation with a UV-LED emitting a wavelength of 365 nm.
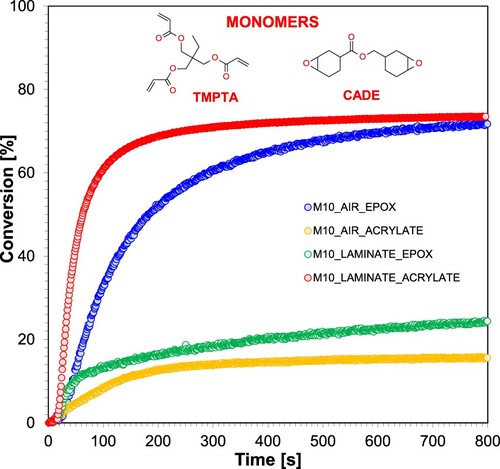
Comparison of the results of the degree of epoxy monomer conversion during the cationic photopolymerisation process with the epoxy monomer conversion obtained during the hybrid photopolymerisation carried out on the pastille shows that significantly more monomer was converted during the hybrid polymerisation. Despite applying the same concentration of initiator and photosensitiser, the hybrid photopolymerisation proceeds more efficiently. This phenomenon may be due to the presence of a radical monomer in the hybrid composition. During the hybrid photopolymerisation carried out on the pastille, the radical monomer is inhibited by oxygen, so it either does not undergo the reaction at all or only to a small extent, so that all of the incipient system found in this composition falls exclusively on the epoxy monomer. The twice higher conversion is a great demonstration of increased efficiency by applying double the initiator concentration reference to CADE. During the process carried out under anaerobic conditions, acrylate monomer conversions are also much higher for hybrid photopolymerisation than for radical photopolymerisation. The processes carried out in the laminate ensure that no oxygen is available to the photopolymerising composition, so the polymerisation of the TMPTA radical monomer is not slowed down. On the other hand, during the hybrid photopolymerisation carried out in the laminate, the radical monomer shows a much higher reaction rate than the cationic monomer. summarises the conversion of epoxy and acrylate functional groups.
Table 6. The obtained conversion rates of acrylate monomer TMPTA and epoxy monomer CADE during the progress of hybrid photopolymerisation initiated by UV-LED light source.
3D printing application
The research described in the previous chapters was a fundamental investigation to select the compound that showed the best photocatalytic properties. Based on the analysis of the performed spectroscopic and kinetic studies, compound M10, which demonstrated the highest performance in two-component photocatalytic systems, was selected for the role of the iodonium salt photosensitiser for application studies.
⇒ Optimisation of the monomer formulation of a photocurable resin
Table 7. Formulation of photo-curable polymerising resins by radical mechanism.
Accordingly, in order to select the formulation showing the best properties for further 3D printing experiments, a Jacob’s test was conducted. This experiment involved printing 8 1 × 1 cm slices from each resin (resins 1–6) at different times (30s, 40s, 50s, 60s, 80s, 100s, 120s and 150s), and then the thickness of each printed slice was measured using a micrometer screw. Based on the quotient of the light intensity of the DLP printing device and the exposure time of each print, the energy E0 (Light energy at the surface) was determined. Based on the graph Cd = f(E0) (), where Cd – thickness of cured slices, the critical energy Ec and depth of light penetration Dp were determined. For this purpose, the formula was employed: Cd = Dp ln[E0/Ec]. shows the obtained results and 3D printing conditions.
Table 8. 3D printing conditions and determined parameters for resins 1–6.
According to the experiment, resin 2 was selected for subsequent research because compared to the other resins (1, 3–6), it has the lowest critical energy value (118.55 mJ/cm2), which reports the minimum energy that must be supplied to the system in order to crosslink the resin. To obtain prints from resin 2, a lower intensity of light incident on the photopolymerising resin (7.96 mW/cm2) was used than for resins 5 and 6 (9.95 mW/cm2).
⇒ Optimisation of photosensitiser concentration
The subsequent research step to finalise the formulation of the radical resin for 3D printing was to optimise the concentration of the M10 photosensitiser. Fourier transform infrared spectroscopy was used for the experiments. Five compositions (Resin 2a–2e) were prepared, varying the amount of contained photosensitiser (0.1–1.0%). shows the exact contents of each component in the resin.
Table 9. Formulation of radical resins 2a–2e.
The photopolymerisation kinetics experiments were carried out using a Vis-LED@405 diode as a light source (the intensity of light incident on the photopolymerising sample was 7.96 mW/cm2, which corresponds to the value of light intensity incident on the print during Jacob’s basic working curves test for resin 2). Each measurement lasted 900s. The measurements were performed under anaerobic conditions, on 0.1 mm thick rings (the ring thickness corresponds to the thickness of the layer during the 3D printing process). shows the kinetic profiles obtained during the radical photopolymerisation of 2a–2e resins. The conversion of acrylate groups was determined based on the disappearance of the band, the maximum of which is located at a wavenumber of 6165 cm−1.
Figure 14. Kinetic profiles achieved during radical photopolymerisation of 2a-2e resins applying LED@405 nm light source.
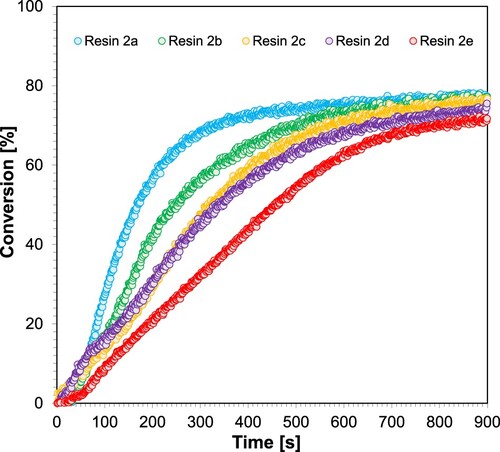
Based on the kinetic profiles received, the conversion of functional (acrylate) groups, the induction time for each of the investigated resins differentiated by the concentration of photosensitiser (2a–e) and the slope of the kinetic curve were determined. below shows the obtained results.
Table 10. The obtained conversion rates of acrylate monomers, the values of the induction time and the slope of the kinetic curve during the progress of hybrid photopolymerisation initiated by Vis-LED light source (LED@405 nm).
Estimating the efficiency of initiation with a two-component photocatalytic system: IOD + different concentrations of M10, three parameters were taken into account, namely: the degree of double-bond conversion, the slope of the kinetic curve, and the induction time. The conducted tests showed that the highest initiation abilities were obtained for the IOD + M10 system (1.0/0.1% w/w), meaning resin 2a, which was subsequently employed to obtain printed polymer nanocomposites.
⇒ Choice of kind and concentration of nano additive for preparation of photo-curable polymer nanocomposites
ZnO nanoparticles, which have recently gained increasing interest due to their photocatalytic, antibacterial and improved stability to ultraviolet radiation properties, were chosen to produce polymer nanocomposites [Citation80–83]. A series of properties make ZnO nanopowder suitable for a wide range of industrial applications including: piezoelectric devices, chemical sensors, optoelectronics, solar cells and spin electronics. It is also applied in the pharmaceutical and cosmetic industries, as well as in the medical and textile industries. The following chapter introduces another application of ZnO nanopowder, that is, utilising it to obtain photo-curable polymer nanocomposites during the DLP light-initiated 3D printing process.
The wide application spectrum of zinc oxide led to its choice to produce a photo-curable nanocomposite via DLP technology. For this purpose, resin 2 (initiator system IOD/M10 (1.0/0.1% w/w), resin formulation: Ebecryl 4740/Ebecryl 130/Photomer 4012 (70/15/15 w/w/w) was modified with 10% w/w addition of 20 nm zinc oxide nanoparticles ()). The resulting resin was marked as Resin 7. The 10% wt. addition of ZnO was chosen based on 3D printing experiments. With a higher content of ZnO nanoparticles, there was a problem with the curing of the photo-curable resin, due to the low light transmission through the resin.
Table 11. Formulation of radical resin 2a and resin 7.
Printing parameters were determined by the Jacob working curves method, as was done for resins 1–6. For this purpose, eight slices were printed from resin 7 applying different exposure times ranging from 30 to 150 s. Their thickness was measured to determine Ec and Dp (). shows the printing conditions and the obtained basic 3D printing parameters for resins 2 and 7 (resin 2 + 10% ZnO). It can be seen that adding 10% w/w of ZnO nanoparticles decreased the critical energy almost two times from 118.55 mJ/cm2 (resin 2) to 65.56 mJ/cm2 (resin 7), but also significantly reduced the penetration depth from 267.8 µm to 170.6 µm (). Similar results were previously reported by Korčušková, et al., who documented that the changes were related not only to the compromised optical clarity and light scattering on the particles but also to the specific ZnO properties causing a photothermal effect [Citation84].
Table 12. 3D printing conditions and determined parameters for resins 2 and 7 (resin 2 + 10% ZnO).
The following stage was 3D printed by DLP technology from resin 2 and resin 7 and optical visualisation of the resulting prints. Initially, a 3D model was prepared in Fusion 360 software and then printed from resin 2 and resin 7. Based on experiments on determining the basic printing parameters, the most optimal exposure times for each layer of resin during printing, as well as the intensity of light incident on the printed object, were selected. The two initial layers were exposed for 100s, while the subsequent layers were exposed for 30s for both resin 2 and resin 7. The printer’s light irradiance in the printing vat was 7.96 mW/cm2. The resulting prints were cleaned with isopropanol from the uncured resin, and subjected to optical visualisation by optical and scanning electron microscopes. The following graphics show images of prints obtained with DLP technology from resin 2 () and resin 7 ().
⇒ SEM analysis
Figure 16. Photographs of the print obtained with resin 2 using DLP technology; images taken on an optical microscope and camera; images taken under visible light and with a UV flashlight.
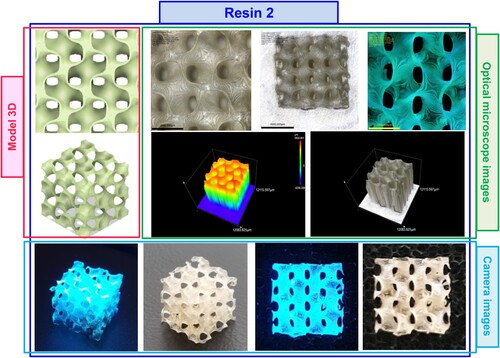
Figure 17. Photographs of the print obtained with resin 7 using DLP technology; images taken on an optical microscope and camera; images taken under visible light and with a UV flashlight.
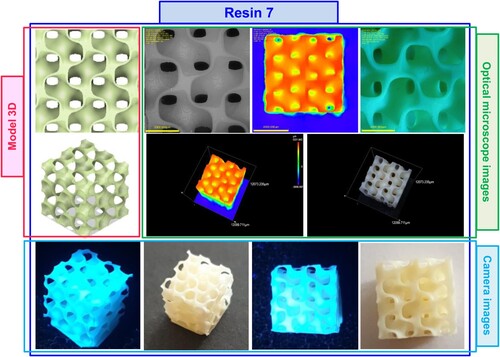
Figure 18. Photographs of a print obtained with resin 2 using DLP technology; images obtained on a scanning electron microscope.
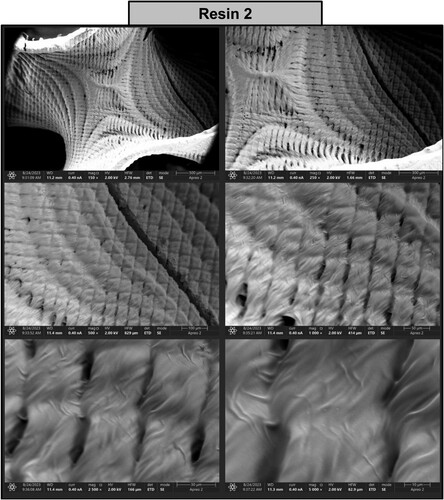
Figure 19. Photographs of a print obtained with resin 7 using DLP technology; images obtained on a scanning electron microscope.
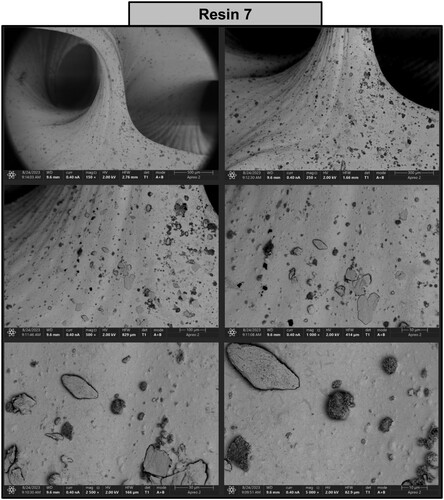
The structure printed from resin 2 reveals the pattern of the individual voxels with a dimension of approximately 50 µm separated by microdefects (). The addition of nanoparticles (resin 7) smoothened the surface and rendered the individual voxels almost indistinguishable (). Furthermore, agglomerated nanoparticles were visible on the surface of the printed structure. In addition, an elemental composition tested by SEM-EDS (energy dispersive spectroscopy) mapping revealed the presence of carbon (C), oxygen (O) and zinc (Zn), confirming that the inclusions are formed by aggregated ZnO nanoparticles. Analysis of the print obtained from resin 2 confirmed the presence of carbon (44.90%) and oxygen (55.10%) in the examined sample, as shown in (a). While analysis of the print obtained from resin 7 showed that the following chemical elements were present in the analysed printout: carbon (46.40%), oxygen (35.30%), and zinc (18.20%), as shown in (b).
⇒ Thermomechanical investigation
The DMA results () revealed that the ZnO nanoparticles shifted Tg from 50.3°C (resin 2) to 44.5°C (resin 7) and reduced the network density (υe) from 48.5 kmol·m−3–41.2 kmol·m−3, documenting the deteriorated curing of the nanoparticle resin () [Citation84]. At the same time, the maximum of the loss factor (tan δ) decreased from 0.44–0.36 while the glassy modulus (E’glassy) determined at –25°C increased from 2.84 GPa to 2.91 GPa, despite the resin’s 2 higher Tg. It suggests that nanoparticles slightly pronounce the contribution of elastic deformation, probably due to a weak nanoreinforcement effect [Citation85, Citation86]. A previous study utilising 2% v/v (≈9.4% w/w) of 20 nm ZnO nanoparticles in a free radical acrylic 3D printing resin with 6% of diphenyl (2,4,6-trimethylbenzoyl)-phosphine oxide (TPO) as a photoinitiator reported analogical drop in Tg by 10.2 °C from 54.2 to 44.0°C attributed to the photothermal effect and scattering caused by the nanoparticles [Citation84]. Despite using a different resin and printer, these results suggest that diaryl iodonium salt with the newly developed pentafluorostilbene derivative (M10) as a photosensitiser represent a more efficient photoinitiating system for printing nanocomposites than the commonly used phosphine oxides.
Figure 21. Dynamical mechanical analysis (DMA) analysis showing storage and loss moduli, and loss factor as a function of temperature for resin 2 (without nanoparticles) and resin 7 (with nanoparticles).
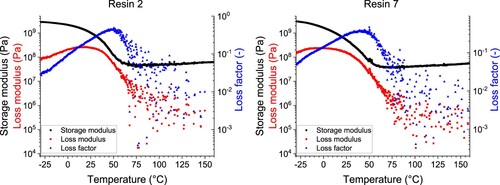
Table 13. 3D printing conditions and determined parameters for resins 1–7. Glassy at −25, rubbery at +120.
The thermal decomposition in non-oxidative atmosphere was studied by TGA (). The resin 2 degraded in two major steps with a maximum decomposition rate at 371 and 463°C, which were accompanied by several minor steps. The nanocomposite resin 7 started to decompose at lower temperatures (330°C) while the main decomposition peak split into two processes at 411 and 446°C. The lower thermal resistance correlates with the less developed network revealed by DMA measurement (). The residue at 700°C contributed 3.4 and 14.0% w/v for resins 2 and 7, respectively, corresponding to an additional 11.0% w/w thermally stable inclusions in the nanocomposite resin. That exceeds the designated nanoparticle loading of 10% w/w, which may be caused by trapping the matrix in the voids between the particles.
Conclusions
The presented pentafluorostilbene derivatives demonstrate favourable absorption properties, especially in visible light, absorbed up to ∼420–450 nm. Such absorption was obtained easily using polarised D-π-A structure design using electron-donating amine groups which exhibit high photosensitising activity. Such structures allow to obtain absorption compatible with the emission of light sources applied in most DLP printers (λ = 405 nm), what increase photosensitising activity of presented stilbenes as well as photoinitiating activity of two-component systems with commercial diaryliodonium salt. Another advantage of pentafluorostilbene is their very beneficial redox potential. They easily reduced iodonium salts, ensuring high efficiency of active species generation such as superacids or radicals. It allows to efficiently initiate radical, cationic and hybrid photopolymerisation utilising a light source from both ultraviolet and visible range. According to conducted measurements, the most effective photoinitiating system (M10 + IOD) was selected, which in a further stage of research was employed for application research related to the preparation of photo-curable polymer nanocomposites using vat 3D printing technology. The high photoactivity of the M10 + IOD system allowed its application as an efficient photoinitiating system for vat 3D printing of ZnO-nanocomposites using DLP technology. Obtained printed 3D objects have desire resolution (limited only by pixels displayed by printer) even with 10% w/w of ZnO filler. These results clearly indicate the high potential of using the presented pentafluorostilbene as photosensitisers in two-component photoinitiating systems for nanocomposite vat 3D printing. However, such highly beneficial properties of presented compounds should allow to use presented compounds in even more advanced applications. Therefore, in the next stage of research pentafluorostilbenes will be investigated in two-component photoinitiating systems for photoinduced atom transfer radical polymerisation (photo-ATRP) polymerisation.
Supplemental Material
Download MS Word (6.2 MB)Acknowledgements
The present work was funded by the OPUS LAP project contract number 2020/39/I/ST5/03556 ‘Advanced photopolymerized nanocomposite materials processed by additive manufacturing’.
Disclosure statement
No potential conflict of interest was reported by the authors.
Data availability statement
The data that support the findings of this study are openly available in Mendeley Data at https://data.mendeley.com/datasets/52m89r83df/1.
Additional information
Funding
References
- Kaya K, Koyuncu S, Yagci Y. Photoinduced synthesis of poly(N-ethylcarbazole) from phenacylium salt without conventional catalyst and/or monomer. Chem Commun. 2019;55:11531–11534. doi:10.1039/C9CC04968A
- Topa M, Ortyl J. Moving towards a finer way of light-cured resin-based restorative dental materials: recent advances in photoinitiating systems based on iodonium salts. Materials. 2020;13:4093, doi:10.3390/ma13184093
- Balcerak A, Kabatc J, Czech Z, et al. High-performance UV-vis light induces radical photopolymerization using novel 2-aminobenzothiazole-based photosensitizers. Materials. 2021;14:7814. doi:10.3390/ma14247814
- Voet VSD, Guit J, Loos K. .Sustainable photopolymers in 3D printing: a review on biobased, biodegradable, and recyclable alternatives Macromol Rapid Commun. 2021;42:2000475. doi:10.1002/marc.202000475
- Bao Y, Paunović N, Leroux JC. Challenges and opportunities in 3D printing of biodegradable medical devices by emerging photopolymerization techniques. Adv Funct Mater. 2022;32:2109864, doi:10.1002/adfm.202109864
- Bagheri A, Jin J. Photopolymerization in 3D printing. ACS Appl Polym Mater. 2019;1:593–611. doi:10.1021/acsapm.8b00165
- Jankowska M, Chachaj-Brekiesz A, Trembecka-Wójciga K, et al. Novel multi-material photo-curable resins containing high-performance photoinitiating systems and nano additives dedicated to 3D-VAT printing. Polym Chem. 2023;14:2088–2106. doi:10.1039/D2PY01583H
- Štaffová M, Ondreáš F, Svatík J, et al. 3D printing and post-curing optimization of photopolymerized structures: basic concepts and effective tools for improved thermomechanical properties. Polym Test. 2022;108:107499. doi:10.1016/j.polymertesting.2022.107499
- Hola E, Topa M, Chachaj-Brekiesz A, et al. New, highly versatile bimolecular photoinitiating systems for free-radical, cationic and thiol–ene photopolymerization processes under low light intensity UV and visible LEDs for 3D printing application. RSC Adv. 2020;10:7509–7522. doi:10.1039/C9RA10212D
- Lago MA, Rodríguez-Bernaldo de Quirós A, Sendón R, et al. Photoinitiators: a food safety review. Food Addit Contam Part A. 2015;32:779–798.
- Arman Kandirmaz E, Kayaman Apohan N, Gençoğlu EN. Preparation of novel thioxanthone based polymeric photoinitiator for flexographic varnish and determination of their migration behavior. Prog Org Coat. 2018;119:36–43. doi:10.1016/j.porgcoat.2018.02.012
- Hola E, Pilch M, Galek M, et al. New versatile bimolecular photoinitiating systems based on amino-m-terphenyl derivatives for cationic, free-radical and thiol–ene photopolymerization under low intensity UV-A and visible light sources. Polym Chem. 2020;11:480–495. doi:10.1039/C9PY01091B
- Tomal W, Pilch M, Chachaj-Brekiesz A, et al. Photoinitiator-catalyst systems based on meta-terphenyl derivatives as photosensitisers of iodonium and thianthrenium salts for visible photopolymerization in 3D printing processes. Polym Chem. 2020;11:4604–4621. doi:10.1039/D0PY00597E
- Lu H, Zhu X. Synthesis of D-π-A-π-D photoinitiator with high-photoinitiation efficiency by restricting photoinduced isomerization process. J Appl Polym Sci. 2022;139:5.
- Chi T, Somers P, Wilcox DA, et al. Tailored thioxanthone-based photoinitiators for two-photon-controllable polymerization and nanolithographic printing. J Polym Sci B Polym Phys. 2019;57:1462–1475. doi:10.1002/polb.24891
- Borys F, Tobiasz P, Poterała M, et al. Development of novel derivatives of stilbene and macrocyclic compounds as potent of anti-microtubule factors. Biomed. Pharmacother. 2021: 133:110973.
- De Filippis B, Ammazzalorso A, Amoroso R, et al. Stilbene derivatives as new perspective in antifungal medicinal chemistry. Drug Dev Res. 2019;80:285–293. doi:10.1002/ddr.21525
- Yang T, Fang L, Rimando AM, et al. A stilbenoid-specific prenyltransferase utilizes dimethylallyl pyrophosphate from the plastidic terpenoid pathway. Plant Physiol. 2016;171:2483–2498. doi:10.1104/pp.16.00610
- Pecyna P, Wargula J, Murias M, et al. More than resveratrol: new insights into stilbene-based compounds. Biomolecules. 2020;10:1–40. doi:10.3390/biom10081111
- Mikstacka R, Stefański T, Różański J. Tubulin-interactive stilbene derivatives as anticancer agents. Cell Mol Biol Lett. 2013;18:368–397. doi:10.2478/s11658-013-0094-z
- Krohn OA, Quick M, Ioffe IN, et al. Time-resolved photochemistry of stiffened stilbenes. J Phys Chem B. 2019;123:4291–4300. doi:10.1021/acs.jpcb.9b00784
- Raucci U, Weir H, Bannwarth C, et al. Chiral photochemistry of achiral molecules. Nat Commun. 2022;13:1, doi:10.1038/s41467-022-29662-1
- Weir H, Williams M, Parrish RM, et al. Nonadiabatic dynamics of photoexcited cis-stilbene using Ab initio multiple spawning. J Phys Chem B. 2020;124:5476–5487. doi:10.1021/acs.jpcb.0c03344
- Wang Y, Liu Y, Bersuker IB. Sudden polarization and zwitterion formation as a pseudo-Jahn–Teller effect: a new insight into the photochemistry of alkenes. Phys Chem Chem Phys. 2019;21:10677–10692. doi:10.1039/C9CP01023H
- Krishnan SB, Clark RJ, Lin X, et al. α-Methylstilbene Isomers: Relationship of Structure to Photophysics and Photochemistry. J Phys Chem A. 2022;126:8976–8987. doi:10.1021/acs.jpca.2c06319
- Hsu YF, Hong JW, Yang JS. A highly fluorescent cis-stiff-stilbene. ChemPhotoChem. 2023;7:1.
- Liu J, Li T, Li R, et al. Hepatic organoid-based high-content imaging boosts evaluation of stereoisomerism-dependent hepatotoxicity of stilbenes in herbal medicines. Front Pharmacol. 2022;13:862830, doi:10.3389/fphar.2022.862830
- Zhao Y, Yang Z, Zhang Z, et al. The first chromosome-level Fallopia multiflora genome assembly provides insights into stilbeny biosynthesis. Hortic Res. 2023;10:5.
- Wu JY, Ding HY, Wang TY, et al. A new stilbene glucoside from biotransformation-guided purification of Chinese herb Ha-Soo-Oh. Plants. 2022;11:17.
- Chen JY, Lian X, Fan YW, et al. Four new stilbenes and one new flavonoid with potential antibacterial and anti-SARS-CoV-2 activity from Cajanus cajan. J Nat Med. 2023;77:858–866. doi:10.1007/s11418-023-01727-5
- Al-Khayri JM, Mascarenhas R, Harish HM, et al. Stilbenes, a versatile class of natural metabolites for inflammation—an overview. Molecules. 2023;28:9.
- Li X, Cai X, Zeng Q, et al. Exploring the mechanism of stilbenes to quench singlet oxygen based on the key structures of resveratrol and its analogues. Food Chem. 2023;403:134350, doi:10.1016/j.foodchem.2022.134350
- Salas H, Gutiérrez-Bouzán C, López-Grimau V, et al. Respirometric study of optical brighteners in textile wastewater. Materials (Basel). 2019;12:5. doi:10.3390/ma12050785
- Dekanić T, Pušić T, Soljačić I, et al. The influence of iron ions on optical brighteners and their application to cotton fabrics. Materials (Basel). 2021;14:17, doi:10.3390/ma14174995
- Aneklaphakij C, Chamnanpuen P, Bunsupa S, et al. Recent green technologies in natural stilbenoids production and extraction: the next chapter in the cosmetic industry. Cosmetics. 2022;9:5. doi:10.3390/cosmetics9050091
- Krambeck K, Oliveira A, Santos D, et al. Identification and quantification of stilbenes (piceatannol and resveratrol) in Passiflora edulis by-products. Pharmaceuticals. 2020;13:4, doi:10.3390/ph13040073
- Dou J, Sui M, Malinen K, et al. Spruce bark stilbenes as a nature-inspired sun blocker for sunscreens. Green Chem. 2022;24:2962–2974. doi:10.1039/D2GC00287F
- Choiri S, Fitriastuti R, Faradiva FZ, et al. Antioxidant activity and nano delivery of the most frequently applied stilbene derivates: a brief and recent review. Pharm Sci. 2022;28:365–375.
- Digafie Z, Melaku Y, Belay Z, et al. Synthesis, molecular docking analysis, and evaluation of antibacterial and antioxidant properties of stilbenes and pinacol of quinolines. Adv Pharmacol Pharm Sci. 2021;2021:6635270.
- Piekuś-Słomka N, Mikstacka R, Ronowicz J, et al. Hybrid cis-stilbene molecules: novel anticancer agents. Int J Mol Sci. 2019;20:6, doi:10.3390/ijms20061300
- Yousuf M, Jinka S, Adhikari SS, et al. Methoxy-enriched cationic stilbenes as anticancer therapeutics. Bioorg Chem. 2020;98:103719, doi:10.1016/j.bioorg.2020.103719
- Levenson AS. Metastasis-associated protein 1-mediated antitumor and anticancer activity of dietary stilbenes for prostate cancer chemoprevention and therapy. Semin Cancer Biol. 2022;80:107–117. doi:10.1016/j.semcancer.2020.02.012
- Tan YJ, Ali A, Tee SY, et al. Galloyl esters of trans-stilbenes are inhibitors of FASN with anticancer activity on non-small cell lung cancer cells. Eur J Med Chem. 2019;182:111597, doi:10.1016/j.ejmech.2019.111597
- O’Croinin C, Guerra AG, Doschak MR, et al. Therapeutic potential and predictive pharmaceutical modeling of stilbenes in Cannabis sativa. Pharmaceutics. 2023;15:7.
- Jesus A, Sebastião AI, Brites G, et al. A hydrophilic sulfated resveratrol derivative for topical application: sensitization and anti-allergic potential. Molecules. 2023;28:7. doi:10.3390/molecules28073158
- Bakrim S, Machate H, Benali T, et al. Natural sources and pharmacological properties of pinosylvin. Plants. 2022;11:12. doi:10.3390/plants11121541
- Chen CT, Fang PH, Jou JH, et al. Spirally configured cis-Stilbene/fluorene (STIF), 10,11-benzo-, and imidazole-fused STIF hybrids as electron-transporting materials for organic light-emitting diode applications. Chem Asian J. 2023;18:e202300482, doi:10.1002/asia.202300482
- Wang H, Wu H, Bian G, et al. An anthracene-based bis-stilbene derivative as luminescent materials for organic light emitting diodes. Materials (Basel). 2023;16:10.
- Ruan S-B, Chan C-Y, Ye H, et al. A spirofluorene-end-capped bis-stilbene derivative with a low amplified spontaneous emission threshold and balanced hole and electron mobilities. Opt Mater (Amst). 2020;100:109636, doi:10.1016/j.optmat.2019.109636
- Ashassi-Sorkhabi H, Salehi-Abar P. Evaluation of the performance of stilbene-based hole transport materials with an emphasis on their configuration for use in perovskite solar cells. Sol Energy. 2019;188:951–957. doi:10.1016/j.solener.2019.06.076
- Sathiyan G, Dasi G, Ramasamy SK, et al. Stilbene-containing carbazole-based fullerene derivatives as alternative electron acceptor for efficient organic solar cells. Appl Nanosci. 2023;13:4101–4108. doi:10.1007/s13204-022-02707-z
- Park IH, Chu L, Leng K, et al. Highly stable two-dimensional Tin(II) iodide hybrid organic–inorganic perovskite based on stilbene derivative. Adv Funct Mater. 2019;29:39.
- Strehmel B, Malpert JH, Sarker AM, et al. New intramolecular fluorescence probes that monitor photoinduced radical and cationic cross-linking. Macromolecules. 1999;32:7476–7482. doi:10.1021/ma990773b
- Ortyl J, Sawicz K, Popielarz R. Performance of amidocoumarins as probes for monitoring of cationic photopolymerization of monomers by fluorescence probe technology. J Polym Sci A Polym Chem. 2010;48:4522–4528. doi:10.1002/pola.24243
- Ortyl J, Galica M, Popielarz R, et al. Application of a carbazole derivative as a spectroscopic fluorescent probe for real time monitoring of cationic photopolymerization. Pol. J. Chem. Technol. 2014;16:75–80. doi:10.2478/pjct-2014-0013
- Ortyl J, Fiedor P, Chachaj-Brekiesz A, et al. Review on zinc oxide nanoparticles: antibacterial activity and toxicity mechanism. The applicability of 2-amino-4,6-diphenyl-pyridine-3-carbonitrile sensors for monitoring different types of photopolymerization processes and acceleration of cationic and free-radical photopolymerization under near UV light. Sensors (Switzerland). 2019;19:7, doi:10.3390/s19071668
- Ortyl J, Galek M, Milart P, et al. Aminophthalimide probes for monitoring of cationic photopolymerization by fluorescence probe technology and their effect on the polymerization kinetics. Polym Test. 2012;31:466–473. doi:10.1016/j.polymertesting.2012.01.008
- Ortyl J, Popielarz R. The performance of 7-hydroxycoumarin-3-carbonitrile and 7-hydroxycoumarin-3-carboxylic acid as fluorescent probes for monitoring of cationic photopolymerization processes by FPT. J Appl Polym Sci. 2013;128:1974–1978, doi:10.1002/app.38378
- Ortyl J, Milart P, Popielarz R. Applicability of aminophthalimide probes for monitoring and acceleration of cationic photopolymerization of epoxides. Polym Test. 2013;32:708–715. doi:10.1016/j.polymertesting.2013.03.009
- Tomal W, Krok D, Chachaj-Brekiesz A, et al. Beneficial stilbene-based derivatives: from the synthesis of new catalytic photosensitizer to 3D printouts and fiber-reinforced composites. Eur Polym J. 2021;156:110603, doi:10.1016/j.eurpolymj.2021.110603
- Petko F, Hola E, Jankowska M, et al. 3D-VAT printing of nanocomposites by photopolymerisation processes using amino-meta-terphenyls as visible light-absorbing photoinitiators. Virtual Phys Prototyp. 2023;18:1, doi:10.1080/17452759.2023.2244936
- Al Mousawi A, Dietlin C, Graff B, et al. Meta-terphenyl derivative/iodonium salt/9H-carbazole-9-ethanol photoinitiating systems for free radical promoted cationic polymerization upon visible lights. Macromol Chem Phys. 2016;217:1955–1965. doi:10.1002/macp.201600224
- Fu J, Liu W, Hao Z, et al. Characterization of a low shrinkage dental composite containing bismethylene spiroorthocarbonate expanding monomer. Int J Mol Sci. 2014;15:2400–2412. doi:10.3390/ijms15022400
- Danso R, Hoedebecke B, Whang K, et al. Development of an oxirane/acrylate interpenetrating polymer network (IPN) resin system. Dent Mater. 2018;34:1459–1465. doi:10.1016/j.dental.2018.06.013
- Bennett J. Measuring UV curing parameters of commercial photopolymers used in additive manufacturing. Addit Manuf. 2017;18:203–212.
- Bureš F. Fundamental aspects of property tuning in push–pull molecules. RSC Adv. 2014;4:58826–58851. doi:10.1039/C4RA11264D
- Cho JD, Kim HK, Kim YS, et al. Dual curing of cationic UV-curable clear and pigmented coating systems photosensitized by thioxanthone and anthracene. Polym Test. 2003;22:633–645. doi:10.1016/S0142-9418(02)00169-1
- Guy N, Giani O, Blanquer S, et al. Photoinduced ring-opening polymerizations. Prog Org Coat. 2021;153:106159.
- Ortyl J, Wilamowski J, Milart P, et al. Relative sensitization efficiency of fluorescent probes/sensitizers for monitoring and acceleration of cationic photopolymerization of monomers. Polym Test. 2015;48:151–159. doi:10.1016/j.polymertesting.2015.10.006
- Klikovits N, Knaack P, Bomze D, et al. Novel photoacid generators for cationic photopolymerization. Polym Chem. 2017;8:4414–4421. doi:10.1039/C7PY00855D
- Li Y, Shaukat U, Schlögl S, et al. A pyrrole–carbazole photoinitiator for radical and cationic visible light LED photopolymerization. Eur Polym J. 2023;182:111700, doi:10.1016/j.eurpolymj.2022.111700
- Lang M, Hirner S, Wiesbrock F, et al. A review on modeling cure kinetics and mechanisms of photopolymerization. Polymers (Basel). 2022;14:10.
- Chen M, Zhong M, Johnson JA. Light-controlled radical polymerization: mechanisms, methods, and applications. Chem Rev. 2016;116:10167–10211. doi:10.1021/acs.chemrev.5b00671
- Chatani S, Kloxin CJ, Bowman CN. The power of light in polymer science: photochemical processes to manipulate polymer formation, structure, and properties. Polym Chem. 2014;5:2187–2201. doi:10.1039/C3PY01334K
- Shao J, Huang Y, Fan Q. Visible light initiating systems for photopolymerization: status, development and challenges. Polym Chem. 2014;5:4195–4210. doi:10.1039/C4PY00072B
- Wang P, Li J, Yang J, et al. Enabling 3D multilayer electronics through the hybrid of vat photopolymerization and laser-activated selective metallization. Addit Manuf. 2023;74:103717.
- Muflikhun MA, Syahril M, Mamba’udin A, et al. A novel of hybrid laminates additively manufactured via material extrusion – vat photopolymerization. J Eng Res. 2023;1:100146, doi:10.1016/j.jer.2023.100146
- Fantoni A, Ecker J, Ahmadi M, et al. Green monomers for 3D printing: epoxy-methacrylate interpenetrating polymer networks as a versatile alternative for toughness enhancement in additive manufacturing. ACS Sustain Chem Eng. 2023;11:12004–12013. doi:10.1021/acssuschemeng.3c02194
- Bardakova KN, Kholkhoev BC, Farion IA, et al. 4D printing of shape-memory semi-interpenetrating polymer networks based on aromatic heterochain polymers. Adv Mater Technol. 2022;7:1, doi:10.1002/admt.202100790
- Aldeen TS, Ahmed Mohamed HE, Maaza M. ZnO nanoparticles prepared via a green synthesis approach: physical properties, photocatalytic and antibacterial activity. J Phys Chem Solids. 2022;160:110313, doi:10.1016/j.jpcs.2021.110313
- Sirelkhatim A, Mahmud S, Seeni A, et al. Review on zinc oxide nanoparticles: antibacterial activity and toxicity mechanism. Nanomicro Lett. 2015;7:219–242.
- Raha S, Ahmaruzzaman M. ZnO nanostructured materials and their potential applications: progress, challenges and perspectives. Nanoscale Adv. 2022;4:1868–1925. doi:10.1039/D1NA00880C
- EL-Dafrawy SM, Tarek M, Samra S, et al. Synthesis, photocatalytic and antidiabetic properties of ZnO/PVA nanoparticles. Sci Rep. 2021;11:1, doi:10.1038/s41598-021-90846-8
- Korčušková M, Sevriugina V, Ondreáš F, et al. Photoactivity, conversion kinetics, nanoreinforcement, post-curing, and electric/dielectric properties of functional 3D printable photopolymer resin filled with bare and alumina-doped ZnO nanoparticles. Polym Test. 2022;116:107798. doi:10.1016/j.polymertesting.2022.107798
- Zboncak M, Ondreas F, Uhlir V, et al. Translation of segment scale stiffening into macroscale reinforcement in polymer nanocomposites. Polym Eng Sci. 2020;60:587–596. doi:10.1002/pen.25317
- Jancar J, Ondreas F, Lepcio P, et al. Mechanical properties of glassy polymers with controlled NP spatial organization. Polym Test. 2020;90:106640. doi:10.1016/j.polymertesting.2020.106640.