ABSTRACT
Here we developed a tripartite biodegradable rib implant with tissue-specific mechanical properties by employing polycaprolactone and a dual-structure-reinforced selective laser sintering (SLS) strategy. Computational simulation revealed that the tripartite rib implant, with a 9 mm diameter load-bearing structure, exhibited a rib-like three-point bending stiffness of 31.3 N/mm, can withstand a maximum force of 121.5 N, and restore similar respiration movements of natural ribs. The modified SLS enabled the fabrication of the as-designed rib implants with improved mechanical properties while maintaining structural fidelity compared to conventional SLS, and their mechanical properties were validated through three-point bending, impact, and flexural fatigue testing. The 3D-printed biodegradable tripartite rib implant effectively prevented abnormal breathing and significantly improved the chest wall's morphological and physiological functions in a large-scale canine rib defect model. This exploration provides a promising approach to engineer biodegradable rib implants with tissue-specific mechanical properties for the long-term repair of chest wall defects.
1. Introduction
Rib defects resulting from tumour resection, physical trauma, and congenital abnormalities often lead to chest wall deformities, abnormal chest wall movements, insufficient protection of internal thoracic organs, and endanger patients’ lives [Citation1]. Chest wall reconstruction with surgical implants to prevent thorax instability has become a consensus among surgeons [Citation2]. By far, clinically available implants for the treatment of chest wall defects include allografts, non-absorbable synthetic woven meshes (e.g. polypropylene, polyester, and polytetrafluoroethylene patches), and metallic titanium plates [Citation3–5]. Nevertheless, the sources of bone grafts are limited, and synthetic woven meshes rarely provide adequate protection to internal organs. Although titanium plates exhibit remarkable strength, they commonly necessitate manual bending and trimming to conform to patient-specific rib defects during surgeries, leading to time-consuming operations and limited potential for ameliorating chest wall deformities.
Compared to conventional implants with standardised shapes, 3D printing technologies have gradually emerged as compelling approaches for customising implants with patient-specific shapes based on medical data, which provides significant advantages in achieving precise restoration of both aesthetic appearance and normal functionality of the diseased or missing tissues/organs [Citation6]. Initial endeavours in 3D printing of rib implants commence with titanium materials [Citation7,Citation8]. In one relevant study, a 3D-printed titanium alloy rib implant with a patient-specific anatomical shape was used for the reconstruction of large-scale chest wall defects [Citation9,Citation10]. During an 18-month follow-up period, the implanted 3D-printed metallic rib demonstrated remarkable clinical outcomes, as the patient reported no pain, localised tenderness, or breathlessness. Recently, polyether ether ketone (PEEK) has emerged as a promising alternative to titanium for 3D-printed orthopaedic implants due to its inherent bone-like biomechanical properties and excellent compatibility with medical radiologic imaging [Citation11,Citation12]. Clinically applied 3D-printed PEEK implants and their combination with fibula graft have successfully achieved personalised reconstruction of mandibular segmental defects while preserving the original shape and functionality of the mandible [Citation13,Citation14]. PEEK has also been utilised in the development of bionic rib implants for chest wall reconstruction, demonstrating efficacy in addressing substantial rib defects among adult patients [Citation15–17]. However, non-degradable implants have inherent limitations as they introduce permanent foreign entities into the dynamically changing human body, which fail to meet the growth characteristics required for chest wall reconstruction in paediatric patients and may break or displace in a collision, necessitating a second removal operation [Citation18,Citation19].
To overcome the limitations of non-degradable implants, biodegradable polymers have been introduced in the field of chest wall reconstruction [Citation20,Citation21]. Currently, clinically available biodegradable polymers suitable for 3D printing mainly include polycaprolactone (PCL), polylactic acid (PLA), polyglycolic acid (PGA), and poly(lactide-glycolide) (PLGA) [Citation22,Citation23]. However, PGA and PLGA degrade rapidly within a few weeks to months, which does not meet the long-term regenerative requirements for repairing large rib defects. On the other hand, PCL and PLA have a degradation period of up to 3–4 years. Nevertheless, the accumulation of acidic by-products from PLA leads to a reduction in pH in the surrounding tissue and triggers adverse immune responses that often necessitate further interventions [Citation24]. In contrast, PCL materials exhibit a slow degradation rate and possess a chemical structure that minimises physiological issues associated with local pH shifts, making them suitable for long-term treatment of tissue defects in vivo [Citation25]. For instance, Yu et al. [Citation26] employed 3D printing techniques to fabricate biodegradable PCL fixators for mending rib fractures, resulting in decreased postoperative pain and improved fracture healing. Cheng et al. [Citation27] further propelled the clinical application of 3D-printed PCL implants for large-scale chest wall defect reconstruction. However, the substantial discrepancies in mechanical properties between biodegradable polymers (with a modulus of hundreds of MPa) and natural ribs (with a modulus of several GPa) necessitate compromises in the design of implants to balance their aesthetic appearances and mechanical supporting functions, resulting in unmatched block-like appearances of the implants and potentially impacting chest wall dynamics [Citation27].
Here, we proposed to design and fabricate tripartite PCL rib implants (RIs) with tissue-specific mechanical properties for large-scale chest wall reconstruction by utilising clinically available biodegradable polymers and a dual-structure-reinforced selective laser sintering (SLS) strategy (). The biodegradable RI consists of a load-bearing structure to restore the supporting functions and anatomical appearance of the thoracic cage, a porous minimal surface structure to enhance the integration with host tissues, and fixed structures for secure attachment to the residual ribs. The dual-structure-reinforced SLS strategy enables two rounds of sintering for the load-bearing structure, effectively improving the mechanical properties of the fabricated RIs while simultaneously maintaining their structural integrity as compared to conventional SLS. The 3D-printed biodegradable PCL RIs were implanted in canine models with large-scale chest wall defects for 12 months to evaluate their efficacy in restoring the physiological volume and biomechanical supporting functions of the thoracic cages. Moreover, the time-dependent dynamic properties of the biodegradable RIs as well as the surrounding tissues were investigated, providing valuable insights into their long-term performances.
2. Materials and methods
2.1. Characterisation of natural ribs
The structural parameters of beagles’ native ribs were obtained based on medical computerised tomography data provided by Tangdu Hospital (Air Force Medical University, China). The rib contour lines were fitted with circles and utilised for the design of biodegradable RIs. Quasi-static three-point bending was performed on beagles’ ribs by using an electromechanical universal testing apparatus (103A, Wance, China) with a span length of 7.5 cm and a load cell of 200 N. Before testing, the muscles and soft tissues surrounding the ribs were removed. The native rib was oriented in a convex direction onto the testing machine according to previously established literature [Citation28]. It was securely affixed to the testing plate using a specially designed metallic clamp, ensuring no slippage occurred during the experiment. All tests were performed via a 10 mm diameter indenter with a maximum displacement of 4 mm at a speed of 1 mm/min. The reaction force and displacement data of six specimens were recorded, from which the average stiffness and maximum loading capability were calculated as the criteria for the evaluation of 3D-printed biodegradable RIs. Additionally, the elastic modulus of native ribs was tested using the same testing apparatus [Citation29]. In brief, the native ribs were trimmed into a short cylinder segment with a height of 5 mm, which was placed on the testing plate for quasi-static compression. The strain–stress curves were derived from the load-displacement data, and the initial slopes of the curves were calculated as the elastic modulus of natural ribs.
2.2. Finite element analyses of the as-designed rib implants
Finite element analysis (FEA) was used to investigate the biomechanical properties of the as-designed load-bearing structures. All material properties were set as given in and assumed to be homogeneous, isotropic, and linearly elastic. For the native rib model, the geometric model has meshed automatically with the largest element size of 0.3 mm using 3-Matic software (Materialise, Leuven, Belgium) to generate an FE volumetric mesh. For the as-designed load-bearing structure with a circular cross-section, the C3D4 mesh with an average size of 0.3 mm was directly generated by Abaqus 6.14/CAE (Dassault Systemes Simulia Corp., USA). The boundary conditions for simulating the three-point bending of the models are shown in (a). Both ends of the load-bearing structure were fully fixed, while a displacement of 4 mm was applied to the middle of the load-bearing structure along the Z-axis via a rigid indenter. The reaction force was extracted to draw the force-displacement curves for the calculation of the stiffness of the load-bearing structures with varying diameters.
Figure 2. Optimisation of the mechanical properties of the load-bearing structure in PCL RIs. (a) FE models of the load-bearing structures featuring rib-like cross-sections and circle cross-sections. (b) Force-displacement curves of the load-bearing structures with varying cross-sectional diameters. (c) The stiffness and (d) maximum reaction force at a displacement of 4 mm for the load-bearing structures with varying cross-sectional diameters.
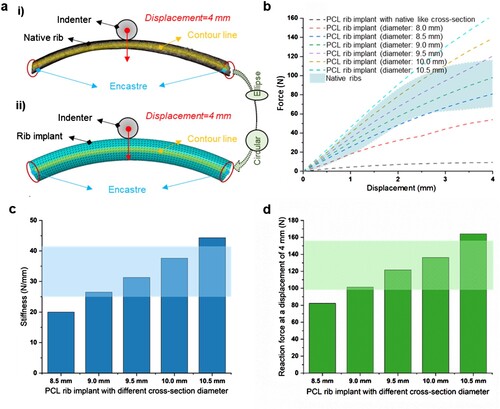
Table 1. Material properties of the components used in FEA.
The biomechanical responses and safety of the as-designed RIs at the implantation site under respiration conditions were simulated by FEA as well. In brief, the RIs without/with minimal surface structures were installed at the rib defect regions. The contact interface between the RIs and residual ribs was considered rigidly bonded to simulate the surgery suture fixation. In nature, the dogs’ chest wall fluctuates about 2 mm during respiration (Figure S1). Consequently, the boundary condition for the posterior extremity of the rib near the spine is fully fixed, while the anterior extremity of the rib closed to the sternum is subjected to an extreme displacement (2, 4, and 6 mm) along the Z-direction, as illustrated in (a). The translation (Ux and Uy) or rotation (URx, URy, and URz) of the rib anterior extremity was constrained. The bending performances of the tripartite RI were further evaluated by extracting the moving trajectory of 6 representative points on the residual rib and implant. In addition, the models employing RIs without the minimal surface structure (L) as well as a natural rib model (R) were also subjected to FEA under the same boundary conditions for comparison.
Figure 3. Computational simulation of the mechanical responses of the designed PCL RI at the implantation site. (a) The boundary conditions for simulating the mechanical responses of L and RI at the implantation site. (b) Contour plot of von Mises stress and displacement predicted for the three FE models corresponding to R, L, and RI. (c) Contour plot of von Mises stress predicted for the load-bearing structures of L and RI. (d) The deformation patterns and (e) quantitative results of the displacement of different representative points for models with R, L, and RI at identical boundary conditions.
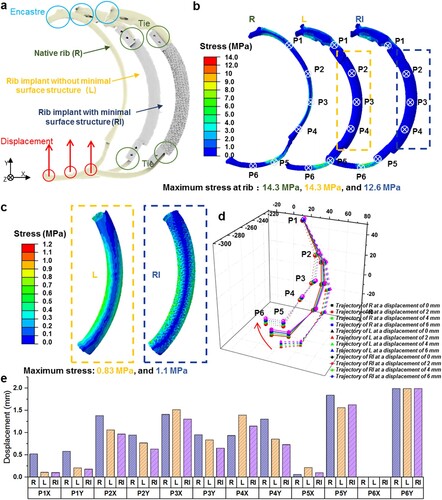
2.3. 3D printing of biodegradable rib implants
Currently, SLS and Fused Filament Fabrication (FFF) are the two primary 3D printing strategies utilised in the fabrication of polymeric medical implants, which have shown significant potential in clinical applications [Citation32]. In comparison to FFF, SLS possesses distinct fabrication flexibility for constructing intricate structures [Citation33,Citation34], making it particularly suitable for fabricating both standalone fixed structures and minimal surface structures of the presented RIs. Therefore, a home-built SLS system with a laser beam diameter of 60 μm was used for the 3D printing of RIs. PCL (CAPA™ 6500, Perstorp, Malmö, Sweden) was grinded into powders with a size smaller than 100 μm for SLS. During the SLS process, processing parameters such as laser power and layer thickness were fixed at 4 W and 0.1 mm, while the scanning speeds for the fabrication of different components of RIs were set as given in . Since the cross-sectional profile of the load-bearing structure for laser scanning is much larger than that of the porous minimal surface structure and fixed structure, it is more prone to accumulate heat and thus leads to structural distortion. To address this issue, a dual-structure-reinforced SLS strategy was proposed to improve the mechanical properties of the RIs and simultaneously maintain their dimensional accuracy ((a)). The effect of laser scanning speed on the mechanical properties of the load-bearing structure was investigated. For the illustration of the heat accumulation during the SLS process, an infrared thermal imager (FLIR X6520 sc, USA) with the built-in software (FLIR ResearchIR) was used to record the temperature distribution.
Figure 4. 3D printing of the designed PCL RI via dual-structure-reinforced SLS strategy. (a) Schematic for the laser scanning of the cross-sections of the RI in conventional single-structure SLS and dual-structure-reinforced SLS strategy. (b) The morphology and (c) stiffness of the SLS-fabricated PCL RI using different laser scanning speeds and strategies. (d) Infrared thermal images during the SLS of the PCL RI using different laser scanning speeds and strategies.
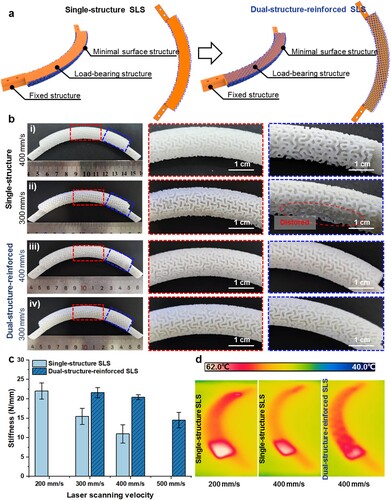
Table 2. Laser scanning speeds for the SLS of different components in PCL RIs.
2.4. Mechanical characterisation of the 3D-printed rib implants
Three-point bending, impact testing, and fatigue testing were used to evaluate the mechanical properties of the 3D-printed RIs. Before the mechanical testing, the partially-melted PCL particles attached on the RIs were effectively removed through the implementation of an in-situ re-melting and re-solidification treatment utilising our previously proposed method [Citation35]. The testing conditions for the three-point bending of the as-built RIs were consistent with those of the native ribs. The force-displacement curves were obtained to compare their mechanical properties to those of natural ribs. The impact test of the 3D-printed RIs was carried out using a pendulum impact tester (Yasuda, Japan) with a pendulum length of 335 mm and a maximum energy of 5.5 J, and the impact toughness (impact energy) was recorded. The fatigue life of RIs is directly affected by the expansion and contraction of the thoracic cage during respiration. To evaluate the flexural fatigue properties of the 3D-printed RIs, fixed structures in the RIs were tightly bonded to specially designed clamps to simulate their surgical fixation with residual ribs in vivo. An electromechanical universal testing apparatus was used to apply a periodical vertical displacement of 2 mm at a loading speed of 40 mm/min for fifty thousand cycles. The evolution of the force-displacement relationship and the maximum reaction were recorded.
2.5. Implantation of the 3D-printed rib implants in a canine model with rib defects
The RIs were implanted in vivo to investigate their biological performances for chest wall reconstruction and the time-dependent dynamic properties. In this study, we employed a large canine model to create critical rib defects, as canines are widely recognised as effective models for investigating bone-related diseases and bone tissue engineering due to their similarities in bone composition and density with humans [Citation36,Citation37]. Furthermore, rib ostectomy models have been developed to evaluate potential strategies for rib regeneration, aiming to address the challenges of instability and cosmesis associated with significant defects. In this aspect, canines are also frequently chosen as suitable models for thoracic wall reconstruction studies due to their resemblance in thoracic cavity dynamics to humans [Citation38,Citation39]. Briefly, eight healthy adult male beagle dogs weighing 12–15 kg, were purchased from Dilepu Biomedical Co., Ltd. (Xian, China). The beagles were randomly divided into two groups: (a) Chest wall defects group without any treatment (Control group, n = 2) and (b) Chest wall defects with the implantation of 3D-printed biodegradable RIs (Implantation group, n = 6). Before operations, the beagle was initially anaesthetised with Zoletil (tiletamine/zolazepam, 10 mg/kg) by intramuscular injection, and then anaesthesia by supplying propofol (0.25 mg/kg/min) and rocuronium (7 mg/kg/min) [Citation40]. The surgical site was prepared by removing hair and disinfecting with Iodophor solutions. As shown in (a), large-scale chest wall defects involving 5th-9th ribs with a total scale of c.a. 10 cm × 10 cm were constructed. After sanitisation, five PCL RIs were fitted and tied to the residual ribs using a silk braided suture (ETHICON, 1-0/T, SA845G). After implantation, the subcutaneous soft tissue was sutured and the incision was closed. The entire experimental procedures were conducted by the surgeons of the Tangdu Hospital, with approval from the Animal Ethics Committee of the Airforce Military Medical University (No. 20230722) and adherence to the National Institutes of Health Guidelines for Care and Use of Laboratory Animals. At 3, 6, and 12 months postoperatively, the beagles in the Implantation group and Control group were assessed by computerised tomography (CT) for the evaluation of the recovery of the chest area. At each time point, 2 beagles from the Implantation group were painless euthanized, and the chest wall containing biodegradable RIs was harvested for further assessments.
2.6. Histological evaluation of the retrieved tissues surrounding the 3D-printed rib implants
The host tissues surrounding the 3D-printed biodegradable PCL RIs were retrieved at 3, 6, and 12 months postoperatively, which were fixed in 10% saline-buffered formalin for 7 days and subjected to routine histological techniques. The specimens were embedded in paraffin blocks and sectioned at 5–10 μm thickness. Haematoxylin and eosin (H&E) staining and Masson trichrome (MT) staining were employed to assess morphological changes and collagen formation of the surrounding tissues. For immunohistochemistry (IHC) staining, antibodies including alpha smooth muscle actin (α-SMA, GB111364-100, dilution 1:500, Servicebio), TGF beta 1 (TGF-β, GB11179-100, dilution 1:500, Servicebio), and alpha fibroblast activation (α-FAP, EPR20021, dilution 1:500, Abcam) were selected to evaluate the soft tissue regeneration and remodelling process surrounding the PCL RI. Briefly, the sample slides were incubated with the above-mentioned primary antibodies overnight at 4 °C, followed by species-appropriate HRP-conjugated secondary antibodies (1:200, GB23303, Servicebio). Immunoreactivity was visualised with diaminobenzidine (DAB, P0202, Beyotime, Shanghai, China). All slices were analyzed and photographed by a microscope (E100, Nikon). Integrated optical density (IOD) was measured in ten randomly selected visual fields per section using ImageJ software (National Institutes of Health, Bethesda, MD, USA). The average optical density (AOD) was calculated as AOD = IOD/area [Citation41].
2.7. Time-dependent dynamic properties of 3D-printed biodegradable rib implants
The biodegradable PCL RIs were harvested and dried using a vacuum dryer before the characterisation of the time-dependent properties. Molecular degradation of RIs as a function of time was determined by advanced polymer chromatography (Waters ACQUITY APC and Malvern OMNISEC REVEAL, USA), using HPLC-grade tetrahydrofuran as the mobile phase (a flow rate of 0.5 ml/min) and polystyrene as a standard [Citation42,Citation43]. The number of molecular weights (Mn), the weight-average molecular weights (Mw), and the polydispersity index, (PDI = Mw/Mn) of specimens were determined.
Quasi-static three-point bending testing (n = 3) was conducted to study the time-dependent mechanical properties of the RIs according to the above-mentioned method (Section 2.5.). The force-displacement curves were recorded, and the stiffness, as well as the maximum bearing capacity, was extracted. Furthermore, the mechanical properties of the chest walls with RIs were assessed. Two halves of the thoracic cage were separately removed from the beagles and placed onto the testing plate of a mechanical testing instrument (PLD-5, Xi’an Lichuang Material Testing Technology Co., Ltd., China). Each half of the thoracic cage experienced a maximum displacement of 8 mm with a compression speed of 0.5 mm/min, and the force-displacement profiles of the chest walls with 3D-printed biodegradable RIs were recorded and compared with those of the healthy chest walls.
2.8. Statistical analysis
Unless otherwise specified, a minimum of three independent replicates were employed for each experiment. All experimental data were expressed as mean ± standard deviation. Statistical comparisons were conducted using one-way analysis of variance (ANOVA) with Tukey’s tests (GraphPad Prism 8 software) to determine statistical significance. P < 0.05 was considered to be a statistically significant difference.
3. Results and discussion
3.1. Design of biodegradable rib implants with tissue-specific mechanical properties
The biodegradable RI was designed to have three key components, including the load-bearing structure, fixed structures, and minimal surface structure. According to the anatomical structure of a natural rib, the contour line diameter for the load-bearing structure of the RI was fixed at 16 cm. As shown in (a), we first keep the profile of the load-bearing structure identical to that of the native rib. However, the simulated force-displacement curve was significantly lower than those of the native ribs (as marked with blue regions in (b)). This was mainly caused by the low elastic modulus of PCL material as compared with natural ribs. To meet the supporting function requirements, we redesigned the cross-section profile of the load-bearing structure to a circle and enlarged the cross-sectional diameter from 8.0 mm to 10.5 mm. It was found that the slopes of the force-displacement curves gradually increased with the increase of the load-bearing structures’ cross-sectional diameter. For the load-bearing structures with a cross-sectional diameter ranging from 9.0 mm to 10.0 mm, the corresponding force-displacement curves were in the range of natural ribs. The stiffness and maximum reaction forces of the load-bearing structures were further calculated for varying diameters ranging from 9 mm to 10 mm. The obtained results revealed a stiffness range of 26.5 N/mm to 37.6 N/mm, along with maximum reaction forces ranging from 101.3 N to 136.2 N ((c,d)). As a result, a median diameter of 9.5 mm was chosen for the load-bearing structure in the RIs, which exhibits a stiffness of 31.3 N/mm and a maximum load-bearing force of 121.5 N, similar to those of natural ribs.
To connect the load-bearing structure to residual ribs, three models of fixed structures (namely, the D design, C design, and O design) were integrated at both ends of the load-bearing structure as shown in Figure S3. The interfaces between the load-bearing structure to the fixed structures were shaded in pink, the interfaces between the fixed structure and residual ribs were indicated with blue, and the pinholes for fixation were presented by yellow asterisks. To ensure the safety of the fixed structures, the surgical fixation procedure was computationally simulated by applying a binding force of 80 N at the pinholes and simultaneously fully constraining the middle of the load-bearing structure and the ending of the residual rib. It was found that the D design structures showed a minimum stress concentration of 8.5 MPa, smaller than that of the C design (11.38 MPa) and O design (15.39 MPa), and thus D design model was selected as the fixed structures for the RIs.
Gyroid-typed triply periodic minimal surface structures possess high specific surface area, which has been proven to promote cell and tissue ingrowth in diverse tissue engineering applications [Citation44,Citation45]. To improve host tissue-implant integration, the load-bearing structure was finally covered with a thin layer of Gyroid-typed minimal surface structure (thickness: 1 mm, line width: 500 μm) to form the resultant tripartite RI.
3.2. Simulating respiration-induced deformation of the rib implants at the implantation site
To further assess the mechanical responses of the designed RIs at the implantation site, the designed implants were fixed at the residual ribs and then subjected to external displacement to simulate normal breath movements. A non-contact three-dimensional full-field strain measurement and analysis system (XTDIC), developed by XTOP 3D Technology (Shenzhen) Co., Ltd., was employed to quantitatively analyze the displacement of ribs during respiration by tracking the images of the chest wall. It was found that the chest wall rises and falls regularly by c.a. 2 mm (Figure S1, Video S1). Therefore, the distribution of von Mises stress and displacement for RIs under a compressive displacement of 2 mm were analyzed as shown in (a). The results of Mises stress for the three rib models (R, L, and RI) are shown in (b). It can be seen that the patterns of the stress distribution and the locations of maximum von Mises stress are highly similar among the three FE models. The maximum von Mises stress of the rib in model R reached up to 14.3 MPa, while the maximum von Mises stress of the rib in model RI and model L reached up to 12.6 and 14.3 MPa. Additionally, the maximum von Mises stress in load-bearing structures, both with and without minimal surface structures, primarily located in the middle of the implants, remained similar at 0.83 and 1.14 MPa respectively ((c)). The maximum stress observed at the ribs and RIs was significantly below the yield strength of bone and PCL materials, suggesting no risk of fracture under normal respiratory conditions.
We also captured the trajectory of the marked 6 representative points on the FE models to obtain the deformation patterns of R, L, and RI ((d,e)). The three models exhibited similar deformation patterns, and the maximum displacement deviation was found to be located at the P1 and P4 regions with a discrepancy not exceeding 0.5 mm. These findings demonstrated that the rib defects repaired by the designed RI can remain the deformation patterns of native ribs, which had the potential to effectively restore respiratory motions of the reconstructed chest walls when implanted in vivo.
3.3. The dual-structure-reinforced SLS strategy for fabricating biodegradable rib implants
The SLS process was employed for the 3D printing of the as-designed tripartite RIs with load-bearing structure, fixed structure, and minimal surface structure due to its unique advantages to fabricating complex porous architectures without the need for additional supporting structures [Citation46]. Since the sintering area varied from one structure to another, we applied different laser scanning speeds for the solid structures and porous structures of the RI ().
We found that when the load-bearing structures were sintered at a speed of 400 mm/s, the minimal surface structure at the surface of the RI was visible and well-defined (b(i)). As the laser scanning speed decreased to 300 mm/s, distorted regions can be clearly observed on the as-fabricated RI (b(ii)). As the laser scanning speed further decreased to 200 mm/s, the minimal surface structure at the surface of the RI was destroyed and became invisible (Figure S4). On the contrary, the stiffness of the fabricated RI significantly increased by c.a. 2.25 times as the laser scanning speed decreased from 400 mm/s to 200 mm/s ((c)). As shown in (d), the slower laser scanning speed of 200 mm/s resulted in more heat accumulation, reaching a peak temperature of 58.2 °C, as compared with that of 400 mm/s (53.6 °C). This led to an enhanced fusion between the PCL powders and improved mechanical performances of the fabricated RI. However, exceeded heat accumulation compromised the surface porous morphology of the RI.
To tackle this predicament, a dual-structure-reinforced SLS strategy was proposed, wherein the load-bearing structure and the minimal surface structure were superimposed along the same centreline. Consequently, continuous scanning of the porous minimal surface structure both externally and internally to the solid load-bearing structure was achieved. The consecutive two rounds of sintering at the load-bearing region are anticipated to enhance mechanical strength while preserving the structural fidelity of the as-designed RIs ((a)).
b(iii-iv) show the morphology of the as-fabricated RI at a scanning speed of 400 and 300 mm/s via the proposed dual-structure-reinforced SLS strategy, demonstrating good structural fidelity. Moreover, the stiffness of the fabricated RI derived from a dual-structure-reinforced SLS strategy at a scanning speed of 400 and 300 mm/s was similar to that of the RI derived from conventional single-structure SLS at a lower scanning speed of 200 mm/s ((c)). The enhanced mechanical properties, while preserving the dimensional accuracy of the RIs, mainly resulted from the improved sintering density inside the load-bearing regions. Micro-CT was employed to visualise the internal morphology of the 3D-printed RIs. The load-bearing structures uniformly laser-sintered at a speed of 400 mm/s exhibited indistinct boundaries and relatively porous morphology (Figure S5a). In contrast, when implementing the dual-structure-reinforced SLS strategy, both the load-bearing structure and minimal surface structure displayed dense and well-defined boundaries. Moreover, organised minimal surface structures with relatively dense grey values were observed within the reinforced load-bearing structure (Figure S5b). On the other hand, infrared thermal images showed the difference in heat accumulation between dual-structure-reinforced SLS and single-structure SLS of RI, as seen in (d). The results indicate that the dual-structure-reinforced SLS has a more uniform temperature distribution and a lower peak temperature compared to the single-structure SLS, thereby leading to less heat-induced distortion.
3.4. The load-bearing capacity of the resultant biodegradable rib implants
Afterward, the SLS-derived RIs were treated by previously-established in-situ remelting and re-solidification procedure at 65°C for 35 minutes to further improve their surface quality. As shown in (a), the stiffness of the resultant RI reached 30.3 ± 0.9 N/mm, which is completely covered by the stiffness of natural ribs. As shown in (b), the impact energy of the resultant PCL RI reached 1.27 ± 0.08 J, which was even higher than that of the native ribs (0.93 ± 0.16 J). Besides the quasi-static mechanical properties, the capability of bearing dynamic loading and deformation is also an important property of the biopolymeric implants for their potential applications in chest wall reconstruction and restoring respiration movements. To address this issue, 50,000-time cyclic three-point bending testing at a maximum displacement of 2 mm was performed on the resultant PCL RI. The series of hysteretic cycles of the RI are shown in (c).
Figure 5. Mechanical characterisation of the 3D-printed biodegradable PCL RI. (a) The force-displacement curve of the resultant PCL RI in a three-point bending test. (b) Evaluation of impact energy of native ribs and 3D-printed PCL RI. (c) The evolution history of load-displacement curves and (d) the maximum reaction forces of the 3D-printed biodegradable PCL RI in a 50000 cyclic three-point bending test.
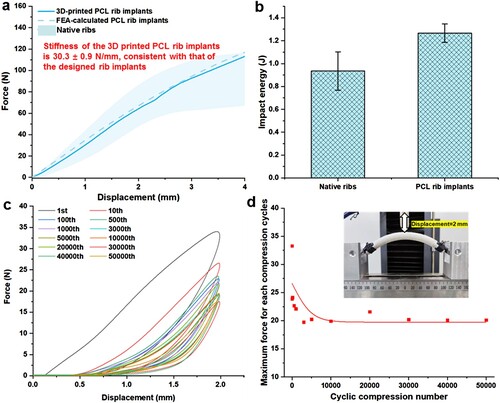
The area of the hysteretic cycles at maximum displacement was observed to rapidly decrease during the initial 10 bending cycles, which gradually reached a stable state for subsequent bending cycles. This phenomenon is similar to the deformation responses of SLS-derived lattice PCL scaffolds under periodical compression as reported in previous literature [Citation34]. (d) shows the changes in the maximum reaction forces at different bending cycles. The maximum reaction force of RI decreased rapidly from c.a. 28.2 N to c.a. 20.3 N during the first 3000 cycles of bending, followed by a relatively stable force level of about 19.8 N even after undergoing a total of 50,000 cycles of bending. These findings demonstrated that the 3D-printed RI possesses rib-specific and robust mechanical properties. Additionally, previous studies have also demonstrated the favourable biocompatibility of the 3D-printed PCL implants both in vitro and in vivo [Citation33]. Therefore, utilising the 3D-printed RI offers potential advantages in terms of providing adequate structural support and biocompatibility for chest wall reconstruction.
3.5. Respiration and morphological function recovery were induced by the rib implants
To further elucidate the role of 3D-printed RI in chest wall reconstruction, resection of the 5th-9th ribs was performed on canine models, resulting in a total defect size of approximately 10 cm × 10 cm ((a)). For the Implantation group, five RIs were fixed to the residual ribs, while no RI was used for the Control group. After closing the chest wall, the Control group showed significant abnormal breathing, while the beagle dogs receiving RI had stable breathing immediately post-operation (Video S2, S3).
(b) illustrates the CT scan images of the implanted PCL implants and chest walls at post-implantation 3, 6, and 12 months. The radiographic examination results show that the dogs’ chest walls of the Control group remained distorted during 12 months of follow-up. In contrast, the chest walls of the dogs in the experimental group shrank significantly at 3 months post-surgery, which gradually restored relatively normal at 6 – and 12-months post-surgery. For the quantitative evaluation of the recovery of the chest wall, the area of the thoracic cage was measured and then compared with that of the corresponding healthy side to obtain a normalised chest area ((c)). It was found that the normalised chest area at the rib defect side and the implantation side gradually increased with the extension of time. At all the time points, the normalised chest area for the implantation sides was higher than those of the defect sides. At the RI implantation side, the normalised chest area at 12 months was significantly higher than those of the defect side at 3 – and 6-months post-operation. The above-mentioned results verified the efficacy of the 3D-printed RI for restoring the morphological and physiological functions of chest walls.
Figure 6. The chest wall morphology and chest area were improved after the implantation of biodegradable PCL RI. (a) Construction of rib defects in canine models and implantation of the 3D-printed RI. (b) CT scan images of the chest wall without and with the implantation of RI during 12 months of follow-up. (c) Changes in the normalised chest area of the rib defect side (marked by orange dash lines) and implantation side (marked by green dash lines) as normalised by the healthy chest area (marked by red dash lines) during 12 months of follow-up.
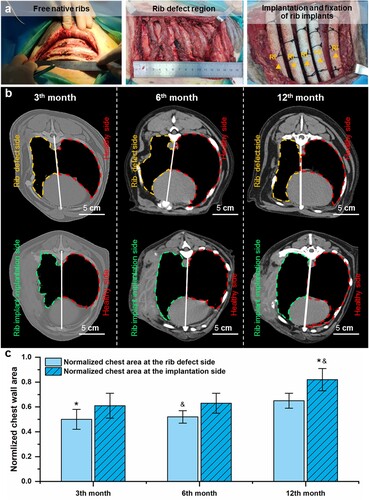
3.6. Robust fibrous tissue integration with the rib implants as confirmed by histological staining
After implantation for 3, 6, and 12 months, the RI with the surrounding tissues were harvested from sacrificed dogs. The typical morphology of the RI with surrounding host tissues is displayed in (a). The 3D-printed RI remained intact and well integrated with host tissues, and no obvious differences were observed at all the time points. H&E staining ((b)) reveals that the host tissues gradually adhered and integrated with the RI, resulting in a concave–convex tissue morphology that replicated the minimal surface structures of the RI. At the interface between the RI and the host tissues, there is a noticeable shift from granulation tissues to fibrosis tissues. The inflammatory cells gradually decreased as the implantation time extended to 6 and 12 months. The histological staining of MT also revealed that the tissues integrated with the RI were fibrous tissues ((c)). At 3 months of implantation, the surrounding tissues consisted of thin muscle fibers and collagen fibers. The collagen fibers gradually interconnected with each other, resulting in dense collagen bundles after 12 months of implantation. Collagen volume fraction (CVF = total collagen area ÷ the total tissue area) was measured and shown in g(i), which significantly increased from 58.48 ± 7.87% to 67.82 ± 13.32%.
Figure 7. Gross views and histological examinations of the harvested PCL RI and its surrounding tissues. (a) Macroscopic observation of RIs with the surrounding tissues at 3-, 6-, and 12-month post-implantation. (b) H&E staining, (c) MT staining, (d) IHC staining for α-FAP, (e) TGF-β, and (f) α-SMA of the surrounding soft tissues at 3, 6, and 12 months after implantation. (g) IHC semiquantitative analyses of the surrounding soft tissues at 3, 6, and 12 months after surgery. (*p < 0.05).
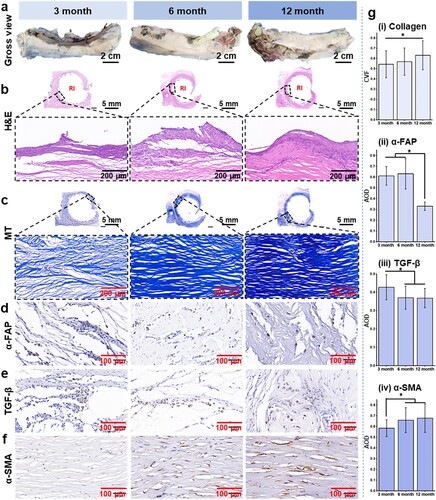
In addition, IHC staining of α-SMA, TGF-β, and α-FAP were conducted to investigate the remodelling process of the surrounding soft tissues. (d,e), and (g)(ii, iii) reveal that both α-FAP and TGF-β exhibited a similar decreasing trend as the implantation time prolonged. The levels of α-FAP and TGF-β reached their peak at 3 months but significantly decreased at 6 – and 12 months post-operation. Conversely, immunostaining images of α-SMA displayed an opposite trend compared to α-FAP and TGF-β, with the level of α-SMA constantly upregulated over time (f and g(iv)). These observations suggest a gradual transition of fibroblasts from an active to a resting state during the tissue healing process, while myofibroblast accumulation occurs in a delayed manner, resulting in fibrosis and abundant collagen production surrounding RI [Citation47]. In the previous view, foreign body reaction stands as a pivotal factor leading to the failure in achieving desired functional tissue regeneration outcomes [Citation48,Citation49]. The presence of fibrous tissue coating on implant surfaces results in fibrosis formation. Conversely, the dense deposition of collagen bundles surrounding the regenerative interface may contribute significantly to restoring mechanical properties in chest wall defects.
3.7. Time-dependent dynamic properties of the rib implants and the retrieved chest walls
The in vivo degradation of 3D-printed PCL implants has been reported to result in a reduction in molecular weight, leading to an initial preservation of mechanical properties followed by a subsequent rapid decline [Citation35]. To confirm the degradation behaviours of the presented PCL RI in rib defect of canine models, the molecular weight, mechanical properties of the RI, and overall mechanical properties of the reconstructed chest walls were tested at different time points. Since the RI presents two distinct regions of solid load-bearing structure (inner region) and porous minimal surface structure (outer region), the samples were separately obtained at these two regions to track their changes in molecular weight.
A continuous decrease of Mn and Mw was found on all samples as a function of the time (a, b). Specifically, the Mn of the minimal surface structure decreased from 22.2 ± 0.52 kDa before the implantation to 15.3 ± 0.44 kDa at month 3, to 11.2 ± 0.33 kDa at month 6, and eventually to 7.5 ± 0.29 kDa at month 12. The Mn of the load-bearing structure of the RI exhibited a slightly slower decrease, with the Mn decreasing to 16.4 ± 0.19 kDa at month 3, to 13.0 ± 0.46 kDa at month 6, and further to 8.5 ± 0.36 kDa by month 12. The slight difference in molecular weight degradation might be mainly caused by the direct and non-direct contact between living tissues and PCL materials. A similar phenomenon was also found for the changes of Mw, with the Mw gradually decreasing from 39.0 ± 0.67 kDa before the implantation to 27.1 ± 0.79 kDa (month 3), 21.3 ± 0.29 kDa (month 6), and 14.6 ± 0.83 kDa (month 12) at the outer regions of the implants. The degradation of Mn and Mw also resulted in a slightly increased PDI over the degradation time (Figure S6), which showed similar degradation trends to previous findings [Citation25,Citation35,Citation43].
Figure 8. Evaluation of time-dependent properties of the retrieved PCL RI at different time points. (a) Mn and (b) Mw changes of the PCL RI over 12 months of implantation. (c) Force-displacement curves of the retrieved PCL RI at different time points. (d) Maximum load-bearing capability and stiffness of the retrieved PCL RI at different time points. (e) Photograph of the compression testing for half of the thoracic cage with RI. (f) Evolution of the force-displacement curves for half of the thoracic cages with RI at different time points.
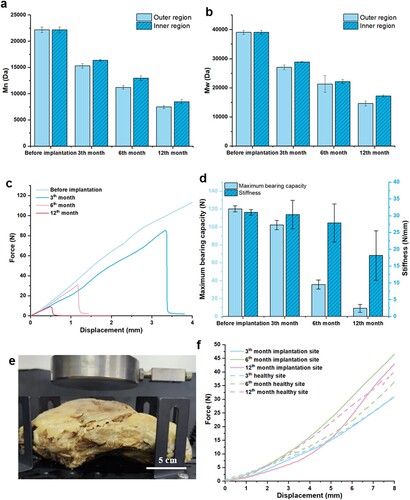
The time-dependent mechanical properties of the 3D-printed RI are shown in (c,d). The typical force-displacement curves obtained from the three-point bending testing of the RI retrieved after in vivo implantation show a linear pattern similar to that before the implantation. However, a sudden fracture appeared when the displacement exceeded c.a. 3.4 mm for implants retrieved at month 3, indicating increased fragility of the implants. This phenomenon became more obvious with the extension of the implantation period. The RI fractured at c.a. 1.2 mm at month 6 and further reduced to c.a. 0.5 mm at month 12 ((c)). Concurrently, the maximum load-bearing capability gradually decreased from 120.1 ± 3.4 N before the implantation to 102.1 ± 5.2 N at month 3, to 35.6 ± 5.1 N at month 6, and finally to 9.0 ± 4.3 N at month 12. On the other hand, the stiffness of the RI first remained at c.a. 30 N/mm for the first 6 months of implantation, and then remarkably decreased to 18.2 ± 7.4 N/mm at month 12 ((d)).
We also conducted compression testing on the extracted half-thoracic cages to assess the restored mechanical functionality of the chest walls with PCL RI ((e)). The force-displacement curves obtained from the compression testing are presented in (f), revealing consistent patterns among all samples at all time points. These findings revealed that, despite the degradation of the mechanical properties of 3D-printed RIs to less than 10% of their initial values after 12 months of implantation, the surrounding tissues dynamically compensated to ensure the overall mechanical integrity of the reconstructed chest walls. This remarkable outcome can mainly be attributed to the bulk degradation process that occurs within these implanted RIs. Previous studies [Citation25,Citation35] have also reported similar degradation trends where PCL undergoes three distinct stages of degradation: an initial phase characterised by chain scission leading to a reduction in Mn during weeks 0-20, followed by progressive loss of mechanical properties from week 20-46, culminating with a decline in mass between week 46-80. Furthermore, the upregulated α-SMA in the surrounding host tissues suggests that the RIs may promote muscle tissue regeneration, leading to long-term stability of the reconstructed chest wall.
Although the results are promising, there are several limitations in this study. Firstly, due to the requirements of animal protection and ethics, the number of animal samples is small. However, our results have clearly shown the efficacy of the presented biodegradable RIs in treating chest wall defects. It is also noteworthy that a few cartilages were formed near the fixed structures of the RI (Figure S7), facilitating the biofixation between the RI and host tissues. However, the complete regeneration of host bone tissues to replace these artificial RIs remains challenging. To this goal, longer-term observations with additional time points should be conducted to gather more comprehensive data regarding the complete degradation of the implants as well as the tissue ingrowth. On the other hand, the incorporation of porous structural designs, osteogenesis inductive biomaterials, and autologous periosteum should be considered in future biodegradable RIs to further enhance their capacity for promoting bone regeneration to achieve improved outcomes in chest wall reconstruction [Citation50,Citation51].
4. Conclusion
In summary, we have developed a tripartite RI with tissue-specific mechanical properties by utilising PCL and implementing a dual-structure-reinforced SLS strategy. FE simulation demonstrated that the load-bearing structure should possess a diameter of 9 mm to provide rib-like mechanical properties and effectively restore normal respiratory movement. The fabrication of well-defined RIs with a stiffness of 30.3 ± 0.9 N/mm, which meets the stiffness requirement of natural ribs, was successfully achieved through two rounds of sequential sintering involving the load-bearing structure and overlapped porous minimal surface structure. The resultant RIs demonstrated histocompatibility and effectively enhanced the aesthetic appearance and area of the deformed chest wall in a canine model. Despite degradation in terms of molecular weight and mechanical properties, the 3D-printed tripartite RIs can effectively maintain the stability and integration of the reconstructed thorax cages via the integration with host soft tissues over 12 months of implantation. It is anticipated that the 3D-printed biodegradable RIs with tissue-specific mechanical properties could emerge as a viable and efficacious solution for chest wall reconstruction in the clinic.
Supplemental Material
Download MS Word (2.5 MB)Disclosure statement
No potential conflict of interest was reported by the author(s).
Data availability statement
The supplementary videos that support the results and findings of this study are available in Figshare at daoi:10.6084/m9.figshare.25476475
.Additional information
Funding
References
- Mansour KA, Thourani VH, Losken A, et al. Chest wall resections and reconstruction: a 25-year experience. Ann. Thorac. Surg. 2002;73(6):1720–1726. doi:10.1016/S0003-4975(02)03527-0
- Liu F-Z, Wang D-W, Zhang Y-J, et al. Comparison of rabbit rib defect regeneration with and without graft. J Mater Sci Mater Med. 2016;28(1):1–5.
- Sanna S, Brandolini J, Pardolesi A, et al. Materials and techniques in chest wall reconstruction: a review. J Vis Surg. 2017;3:95. doi:10.21037/jovs.2017.06.10
- Berthet JP, Wihlm JM, Canaud L, et al. The combination of polytetrafluoroethylene mesh and titanium rib implants: an innovative process for reconstructing large full thickness chest wall defects. Eur J Cardiothorac Surg. 2012;42(3):444–453. doi:10.1093/ejcts/ezs028
- Liptak JM, Dernell WS, Rizzo SA, et al. Reconstruction of chest wall defects after Rib tumor resection: A comparison of autogenous, prosthetic, and composite techniques in 44 dogs. Vet Surg. 2008;37(5):479–487. doi:10.1111/j.1532-950X.2008.00413.x
- Ng WL, An J, Chua CK. Process, material, and regulatory considerations for 3D printed medical devices and tissue constructs. Engineering; 2024. doi:10.1016/j.eng.2024.01.028
- Wen X, Gao S, Feng J, et al. Chest-wall reconstruction with a customized titanium-alloy prosthesis fabricated by 3D printing and rapid prototyping. J Cardiothorac Surg. 2018;13(1):4. doi:10.1186/s13019-017-0692-3
- Danker SJ, Mericli AF, Rice DC, et al. Custom 3D-printed titanium implant for reconstruction of a composite chest and abdominal wall defect. Plast Reconstr Surg Glob Open. 2021;9(11):e3885. doi:10.1097/GOX.0000000000003885
- Goldsmith I, Evans PL, Goodrum H, et al. Chest wall reconstruction with an anatomically designed 3-D printed titanium ribs and hemi-sternum implant. 3D Print Med. 2020;6(1):26. doi:10.1186/s41205-020-00079-0
- Simal I, García-Casillas M, Cerdá J, et al. Three-Dimensional custom-made titanium ribs for reconstruction of a large chest wall defect. Eur J Pediatr Surg Rep. 2016;04(01):0026-030. doi:10.1055/s-0036-1593738
- Kang J, Tian Y, Zheng J, et al. Functional design and biomechanical evaluation of 3D printing PEEK flexible implant for chest wall reconstruction. Comput Methods Programs Biomed. 2022;225:107105. doi:10.1016/j.cmpb.2022.107105
- Honigmann P, Sharma N, Okolo B, et al. Patient-Specific surgical implants made of 3D printed PEEK: material, technology, and scope of surgical application. Biomed Res Int. 2018;2018:1–8. doi:10.1155/2018/4520636
- Li Y, Li Z, Tian L, et al. Clinical application of 3D-printed PEEK implants for repairing mandibular defects. Journal of Cranio-Maxillofacial Surgery. 2022;50(8):621–626. doi:10.1016/j.jcms.2022.06.002
- Kang J, Zhang J, Zheng J, et al. 3D-printed PEEK implant for mandibular defects repair - a new method. J Mech Behav Biomed Mater. 2021;116:104335. doi:10.1016/j.jmbbm.2021.104335
- Wang L, Huang L, Li X, et al. Three-Dimensional printing PEEK implant: A novel choice for the reconstruction of chest wall defect. Ann Thorac Surg. 2019;107(3):921–928. doi:10.1016/j.athoracsur.2018.09.044
- Kang J, Wang L, Yang C, et al. Custom design and biomechanical analysis of 3D-printed PEEK rib prostheses. Biomech Model Mechanobiol. 2018;17(4):1083–1092. doi:10.1007/s10237-018-1015-x
- Wang L, Liu X, Jiang T, et al. Three-dimensional printed polyether-ether-ketone implant for extensive chest wall reconstruction: A case report. Thorac Cancer. 2020;11(9):2709–2712. doi:10.1111/1759-7714.13560
- Mancera J, Echeverri V, Castillo AM, et al. Clinical experience of thoracoplasty with absorbable rib substitutes. Pediatr Surg Int. 2023;39(1):146. doi:10.1007/s00383-023-05413-1
- Komatsu T, Sato T, Sakaguchi Y, et al. Development of a socket-type rib coaptation device made of poly-L-lactide fibers: feasibility study in a canine model. J Thorac Dis. 2018;10(4):2213–2222. doi:10.21037/jtd.2018.03.75
- Yu YH, Hsu YH, Chou YC, et al. Sustained relief of pain from osteosynthesis surgery of rib fracture by using biodegradable lidocaine-eluting nanofibrous membranes. Nanomedicine. 2016;12(7):1785–1793. doi:10.1016/j.nano.2016.04.015
- Bai B, Hao J, Hou M, et al. Repair of large-scale Rib defects based on steel-reinforced concrete-designed biomimetic 3D-printed scaffolds with bone-mineralized microenvironments. ACS Appl Mater Interfaces. 2022;14(37):42388–42401. doi:10.1021/acsami.2c08422
- Youssef A, Hollister SJ, Dalton PD. Additive manufacturing of polymer melts for implantable medical devices and scaffolds. Biofabrication. 2017;9(1):0012002. doi:10.1088/1758-5090/aa5766
- Meng Z, He J, Li J, et al. Melt-based, solvent-free additive manufacturing of biodegradable polymeric scaffolds with designer microstructures for tailored mechanical/biological properties and clinical applications. Virtual Phys Prototyp. 2020;15(4):417–444. doi:10.1080/17452759.2020.1808937
- Maduka CV, Alhaj M, Ural E, et al. Polylactide degradation activates immune cells by metabolic reprogramming. Adv Sci. 2023;10(31):2304632.
- Bartnikowski M, Dargaville TR, Ivanovski S, et al. Degradation mechanisms of polycaprolactone in the context of chemistry, geometry and environment. Prog Polym Sci. 2019;96:1–20. doi:10.1016/j.progpolymsci.2019.05.004
- Yu YH, Lin YT, Hsu YH, et al. Biodegradable antimicrobial agent/analgesic/bone morphogenetic protein-loaded nanofibrous fixators for bone fracture repair. Int J Nanomed. 2021;16:5357–5370. doi:10.2147/IJN.S325885
- Cheng ME, Janzekovic J, Theile HJ, et al. Pectus excavatum camouflage: a new technique using a tissue engineered scaffold. Eur J Plast Surg. 2022;45(1):177–182. doi:10.1007/s00238-021-01902-5
- Ayagara AR, Langlet A, Hambli R. On dynamic behavior of bone: Experimental and numerical study of porcine ribs subjected to impact loads in dynamic three-point bending tests. J Mech Behav Biomed Mater. 2019;98:336–347. doi:10.1016/j.jmbbm.2019.05.031
- Albert DL, Katzenberger MJ, Agnew AM, et al. A comparison of rib cortical bone compressive and tensile material properties: Trends with age, sex, and loading rate. J Mech Behav Biomed Mater. 2021;122:104668. doi:10.1016/j.jmbbm.2021.104668
- Dias D, Belinha J. The computational simulation and impact analysis of the rib bones. J Comput Artif Intell Mech Biomech. 2021;1(3):71–80.
- Meng Z, He J, Li D. Additive manufacturing and large deformation responses of highly-porous polycaprolactone scaffolds with helical architectures for breast tissue engineering. Virtual Phys Prototyp. 2021;16(3):291–305. doi:10.1080/17452759.2021.1930069
- Wang L, Liu W, He J, et al. Treatment of bronchomalacia using three-dimensional printed polycaprolactone scaffold in a pediatric patient. J Thorac Cardiovasc Surg. 2019;157(5):e287–e290. doi:10.1016/j.jtcvs.2018.11.095
- Meng Z, Mu X, He J, et al. Embedding aligned nanofibrous architectures within 3D-printed polycaprolactone scaffolds for directed cellular infiltration and tissue regeneration. Int J Extreme Manuf. 2023;5(2):0025001. doi:10.1088/2631-7990/acbd6c
- Meng Z, He J, Cai Z, et al. Design and additive manufacturing of flexible polycaprolactone scaffolds with highly-tunable mechanical properties for soft tissue engineering. Mater Des. 2020;189:108508. doi:10.1016/j.matdes.2020.108508
- Meng Z, He J, Cai Z, et al. In-situ re-melting and re-solidification treatment of selective laser sintered polycaprolactone lattice scaffolds for improved filament quality and mechanical properties. Biofabrication. 2020;12(3):0035012. doi:10.1088/1758-5090/ab860e
- Lee NN, Kramer JS, Stoker AM, et al. Canine models of spine disorders. Jor Spine. 2020;3(4):e1109. doi:10.1002/jsp2.1109
- Soltani P, Shahnaseri S, Sheikhi M, et al. Comparison of autogenous bone graft and tissue-engineered bone graft in alveolar cleft defects in canine animal models using digital radiography. Indian J Dent Res. 2020;31(1):118–123. doi:10.4103/ijdr.IJDR_156_18
- Li Z, Zhu W, Zhang B, et al. A novel minimally invasive fixation method for flail chest management in a Canine model: an animal research. J Cardiothorac Surg. 2023;18(1):359. doi:10.1186/s13019-023-02445-5
- Taguchi T, Lopez MJ. An overview of de novo bone generation in animal models. J Orthop Res. 2021;39(1):7–21. doi:10.1002/jor.24852
- Liu W, Meng Z, Zheng K, et al. Development of three-dimensional printed biodegradable external airway splints with native-like shape and mechanical properties for tracheomalacia treatment. Mater Des. 2021;210:110105. doi:10.1016/j.matdes.2021.110105
- Kong L, Deng J, Zhou X, et al. Sitagliptin activates the p62–Keap1–Nrf2 signalling pathway to alleviate oxidative stress and excessive autophagy in severe acute pancreatitis-related acute lung injury. Cell Death Dis. 2021;12(10):928. doi:10.1038/s41419-021-04227-0
- He Y, Zhu B, Tan S, et al. Improving the energy storage performance of a chlorinated poly(vinyl chloride) film at elevated electric field and temperature via a simple annealing process. ACS Appl Energy Mater. 2023;6(10):5407–5415. doi:10.1021/acsaem.3c00518
- Sajkiewicz P, Heljak MK, Gradys A, et al. Degradation and related changes in supermolecular structure of poly(caprolactone) in vivo conditions. Polym Degrad Stab 2018;157:70–79. doi:10.1016/j.polymdegradstab.2018.09.023
- Poltue T, Karuna C, Khrueaduangkham S, et al. Design exploration of 3D-printed triply periodic minimal surface scaffolds for bone implants. Int J Mech Sci. 2021;211:106762. doi:10.1016/j.ijmecsci.2021.106762
- Feng J, Fu J, Yao X, et al. Triply periodic minimal surface (TPMS) porous structures: from multi-scale design, precise additive manufacturing to multidisciplinary applications. Int J Extreme Manuf. 2022;4(2):022001), doi:10.1088/2631-7990/ac5be6
- Han W, Kong L, Xu M. Advances in selective laser sintering of polymers. Int J Extreme Manuf. 2022;4(4):042002. doi:10.1088/2631-7990/ac9096
- Nho RS, Ballinger MN, Rojas MM, et al. Biomechanical force and cellular stiffness in lung fibrosis. Am J Pathol. 2022;192(5):750–761. doi:10.1016/j.ajpath.2022.02.001
- Qi Y, Wang C, Wang Q, et al. A simple, quick, and cost-effective strategy to fabricate polycaprolactone/silk fibroin nanofiber yarns for biotextile-based tissue scaffold application. Eur Polym J. 2023;186:111863. doi:10.1016/j.eurpolymj.2023.111863
- Song Y, Li L, Zhao W, et al. Surface modification of electrospun fibers with mechano-growth factor for mitigating the foreign-body reaction. Bioactive Mater. 2021;6(9):2983–2998. doi:10.1016/j.bioactmat.2021.02.020
- Li X, Yuan Y, Liu L, et al. 3D printing of hydroxyapatite/tricalcium phosphate scaffold with hierarchical porous structure for bone regeneration. Bio-Des Manuf. 2020;3(1):15–29. doi:10.1007/s42242-019-00056-5
- Han X, Sun M, Chen B, et al. Lotus seedpod-inspired internal vascularized 3D printed scaffold for bone tissue repair. Bioactive Mater. 2021;6(6):1639–1652. doi:10.1016/j.bioactmat.2020.11.019