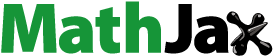
ABSTRACT
3D printing has emerged as an attractive manufacturing technique in supercapacitor electrodes owing to the precise and customisable fabrication of complex electrode designs, enhancing the performance and efficiency of the device. Despite the advantages, 3D-printed electrodes are limited by their low conductivity and electrochemical properties, predominantly due to the lack of availability of suitable conductive materials. To address this limitation, we modified the 3D-printed nanocarbon (3D-PnC) electrode by activation and surface deposition of Ti3C2Tx MXene. A solid-state asymmetric supercapacitor was fabricated by using 3D-PnC/Ti3C2Tx as the negative electrode and polyaniline (PANI) electrodeposited 3D-printed nanocarbon electrode (3D-PnC@PANI) as the positive electrode. The fabricated symmetric supercapacitor exhibits enhancement in overall voltage window, areal capacitance and energy density. The successful operation of the supercapacitor was demonstrated by the illumination of the red light-emitting diodes. Furthermore, this research opens the possibility of utilising MXene-modified 3D-printed electrodes for various electrochemical applications and devices.
1. Introduction
Supercapacitors have emerged as an exciting energy storage solution due to their unique properties [Citation1, Citation2]. Unlike traditional batteries, supercapacitors are ideal for high-power performance applications due to their ability to charge and discharge quickly, as well as their long cycle life. Materials such as transition metal carbides and nitrides (MXenes) [Citation3, Citation4], transition metal dichalcogenides (TMDs) [Citation5], binary transition metal oxide (BTMOs) [Citation6], metal–organic frameworks (MOFs) [Citation7], hexagonal-boron nitride (h-BN) [Citation8], perovskites [Citation9], etc. have recently been widely explored for supercapacitor applications. Challenges such as limited energy density, reduced working voltage window and the need for scalable manufacturing techniques continue to prevent their widespread implementation [Citation10–12]. To address these challenges, there has been a concerted effort to develop energy storage devices through advanced fabrication technologies, such as energy storage and conversion technologies.
Recently, there has been a natural evolution toward designing and manufacturing using additive manufacturing or 3D printing for a wide range of applications in biomedical [Citation13], automotive [Citation14], robotics[Citation15], defence [Citation16], etc owing to its ease of fabrication of complex geometry, with minimal after-processing and maximum material utilisation to achieve zero waste compared to traditional techniques [Citation17, Citation18]. Among other 3D printing processes, fused deposition modelling (FDM) 3D printing is known for its simplicity and accessibility [Citation19]. The FDM method involves the controlled extrusion of thermoplastic filaments through a heated nozzle, resulting in successive layers that produces intricate and fully customised structures. The development of conductive filaments is a notable advancement in FDM 3D printing, as traditionally used thermoplastics are mostly insulating polymers such as acrylonitrile butadiene styrene (ABS), polylactic acid (PLA), polyethylene terephthalate (PET), etc. [Citation20]. However, the introduction of conductive filaments enriched with fillers such as graphene or carbon nanotubes has broadened the application spectra of FDM printing [Citation21–24]. Even though the conductive fillers improve the electrochemical activity of the device, the presence of insulating polymer hinders its electrochemical performance drastically compared to conventional electrodes [Citation25–27]. Various techniques have been reported recently to mitigate this problem by removing the surface polymer content and further exposing the conductive fillers; these techniques include chemical activation [Citation25], thermal activation [Citation28, Citation29], electrochemical activation [Citation30], etc. To further enhance the electrochemical performance of the 3D-printed electrode, efforts have also been made to modify the electrodes by depositing electrochemically active material on the conductive electrodes [Citation25, Citation26, Citation31]. Although, several reports on 3D printed electrodes for supercapacitor applications exist, the need for 3D-printed supercapacitors with enhanced energy density and extended voltage windows still persists. Fabrication of asymmetric supercapacitors that operate in wide voltage window of ≈2 V in aqueous based electrolyte using customised 3D printed electrodes can overcome and the issue of low energy density. Besides, using 3D printing, any complex-shaped electrode can be designed with the same footprint area.
Graphene-like 2D MXenes have recently been studied for various applications [Citation32]. The inherent surface groups and 2D layered structure, in addition to their high conductivity, mechanical stability, and theoretical capacitance, make them desirable for electrochemical applications [Citation33]. Among the MXene family, Ti3C2Tx is particularly attractive for electrochemical applications due to its high specific capacitance, excellent rate capability, and long cycle life, making it suitable for supercapacitors and batteries [Citation34]. Thus, modification of 3D-printed conductive electrodes with Ti3C2Tx can effectively increase the performance of the supercapacitor. On the other hand, polyaniline (PANI) is a versatile conductive polymer that has gained wide attention in energy storage applications due to its fast reversible redox reactions, which show a typical pseudocapacitive behaviour [Citation35–37]. Its simple and cost-effective synthesis methods, such as chemical oxidation, electrochemical polymerisation, etc., make it suitable for large-scale production [Citation38]. Thus, a coaxial coating on a 3D-printed nanocarbon network will provide additional pseudocapacitive reactions that will enhance the performance of a 3D-printed supercapacitor.
In this work, we aim to develop a 3D-printed asymmetric supercapacitor that operates at a wide voltage window. Hence, we developed MXene (Ti3C2Tx) coated 3D-printed nanocarbon (3D-PnC/Ti3C2Tx) to apply as a negative electrode and PANI electrodeposited 3D-printed nanocarbon (3D-PnC@PANI) electrode as a positive electrode. The modification of the 3D-PnC by 2D Ti3C2Tx MXene, and coaxial coating of PANI, boost the capacitive performance of the 3D-PnC. The electrodes are assembled using a gel electrolyte (PVA/Li2SO4) to obtain the solid-state asymmetric supercapacitor (SS-ASC). The fabricated SS-ASC demonstrated enhancement in working voltage (1.6 V). The gel electrolyte improves mechanical stability of the device, and prevents the leakage of electrolyte. This novel approach involves the collective use of 3D-printed nanocarbon-based electrodes, modified with 2D Ti3C2Tx MXene and PANI, to construct an SS-ASC that operates in wide potential window and heightened safety during usage. This work proposes a novel method for fabricating bespoke designed energy storage devices utilising 3D-printed electrodes that are suitable for customised electronic devices.
2. Experimental
2.1. Materials
All materials used were of analytical grades such as aniline, lithium sulfate anhydrous (Li2SO4), and polyvinyl alcohol (PVA) (MW 85000–124000) obtained from Sigma Aldrich, Germany. Sodium hydroxide (NaOH) was purchased from Penta Chemicals, Czech Republic. MXene power (Ti3C2Tx) was procured from Laizhou Kai Kai Ceramic Materials Co. Ltd. (Hong Kong S.A.R.). The conductive graphene/polylactic acid (PLA) filament (Blackmagic®) was acquired from Graphene Laboratories Inc., New York, USA. Deionised water (resistivity >18 MΩ) was used in all experiments.
2.2. 3d printing parameters
The initial electrode design was carried out using the open-access software Autodesk Fusion 360. The electrode shape resembled a circular lollipop on a stick; it consisted of a 15 mm diameter disc-shaped electrode with a 15 mm rectangular stick protruding from it, having a thickness of 0.5 mm, as shown in Figure S5a. The .stl file from Autodesk Fusion 360 was sliced and converted to a .gcode file using PrusaSlicer software. During slicing, the infill was set to 100%, and print settings were adjusted to achieve a 0.10 mm detail. The fill pattern was monotonic for the top and bottom layers, while a gyroid pattern was used for the inner layers. The 3D printing was performed on a Prusa 3D Printer (Prusa i3 MK3S, Czech Republic) using the commercially available conductive nanocarbon PLA filament, Blackmagic® (Graphene Laboratories Inc, USA). For the 3D printing, the nozzle and bed temperatures were set to 215 and 60 °C, respectively. The printed electrodes were then chemically activated by soaking in 4 M NaOH for 5 h, followed by washing with ethanol and deionised water, and then dried in an electric oven at 55°C for 2 h. The activated electrode also demonstrated about a 20% reduction in mass from its original form due to the removal of PLA. Before the electrochemical study, the electrode was further activated by electrochemical activation applying a constant potential of 2 V for 150 s in a phosphate buffer solution (PBS) at pH 7.2, using Ag|AgCl (1 M KCl) as reference electrode and Pt wire as the counter electrode following chronoamperometry technique. These activated electrodes are denoted as 3D-PnC.
2.3. Electrode preparation
The positive electrode was fabricated by electrodeposition of polyaniline (PANI) on 3D-PnC by running cyclic voltammetry (CV) following a previously reported study [Citation39, Citation40]. In short, for the electrodeposition, 10 mM of aniline solution was prepared in 0.1 M HCl solution. The deposition was optimised by carrying out various CV cycles (5, 10, 20, and 40 cycles) in the voltage window of −0.2 to +0.8 V. The PANI-coated 3D-PnC is represented as 3D-PnC@PANI in the subsequent sections.
For negative electrode (3D-PnC/Ti3C2Tx) preparation, it is necessary to balance the charges obtained from positive and negative electrodes, which is done by varying the mass of the active materials of the electrodes [Citation31, Citation41]. As the amount of electrodeposited PANI is optimizsd before, we take the mass of the PANI to determine the required mass of the Ti3C2Tx MXene following equation (Eq. 1) [Citation42]
(1)
(1) A Ti3C2Tx dispersion was prepared by mixing 3 mg of Ti3C2Tx dispersion, 10 wt% carbon blank and 20 µL Nafion™ 117 in 5 mL deionised water and sonicating for 30 minutes. The Nafion was added for better binding of the Ti3C2Tx and carbon blank was added to provide additional conductivity. The prepared dispersion (4.38 mL) was drop-casted on the 3D-PnC electrodes and kept in the oven at 60 °C for 45 minutes for drying to obtain the 3D-PnC/Ti3C2Tx electrode.
2.4. Characterisation
The surface features of the electrode were visualised by scanning electron microscopy (SEM) FEI Verios 460L (Thermo Fisher, Czech Republic) and energy-dispersive X-ray spectroscopy (EDS) using LYRA3 instrument (Tescan Orsay Holding, Czech Republic). Additionally, the crystallographic structure of the electrodes was examined using X-ray diffraction (XRD) with an X-ray powder diffractometer Rigaku SmartLab 3kW (RIGAKU3). The atomic composition of the 3D-printed electrodes was analysed by X-ray photoelectron spectroscopy (XPS) using an AXIS Supra instrument (Kratos Analytical, Japan) with a monochromatic Al Kα (1486.7 eV) excitation source and the spectra were fitted using CasaXPS software.
All electrochemical measurements were carried out on a potentiostat (Metrohm Autolab, PGSTAT 204, Netherlands) operated by Nova 2.1.4 software. In the three-electrode test set-up, the CV Galvanostatic charge–discharge (GCD) and electrochemical impedance spectroscopy (EIS) measurements were carried out in 1 M Li2SO4 using Ag|AgCl (1 M KCl) as the reference electrode and Pt as the counter electrode. The specific capacitances of a single electrode in a three-electrode system, cell capacitance in a two-electrode system from CV and GCD, and energy density and power density are calculated using Eq (S1-5), Supporting Information.
3. Results and discussion
The fabrication route of SS-ASC is decipated in . Initially, the lollipop-shaped nanocarbon electrodes were 3D printed using FDM printer and activated by dissolving the surface PLA, which also enhanced the electrochemical activity of the 3D-PnC electrodes by exposing the carbon nanotube fillers without compromising the structure (a,b). The activated electrode exhibited a notable reduction in sheet resistance, with a significant decrease of 80.89% observed compared to its initial value (Table S2). These 3D-PnC electrodes were then used for the fabrication of positive and negative electrodes for SS-ASC. Positive electrodes were fabricated by electrodeposition of PANI onto the activated 3D-PnC electrodes to obtain a coaxial deposition of PANI on the carbon nanotubes of 3D-PnC, as illustrated in c. For negative electrode preparation, 2D MXene layers were dropcasted on carbon nanotubes of 3D-PnC electrodes by drop casting method (d). This 3D-PnC@PANI positive electrode and 3D-PnC/Ti3C2Tx negative electrode were used for SS-ASC fabricated by sandwiching PVA/Li2SO4 gel electrolyte between positive 3D-PnC@PANI and negative 3D-PnC/Ti3C2Tx electrodes as shown in e.
Figure 1. Schematic presentation of the fabrication of modified 3D-printed nanocarbon electrodes based positive and negative electrodes for all-solid-state asymmetric supercapacitor and its practical application.
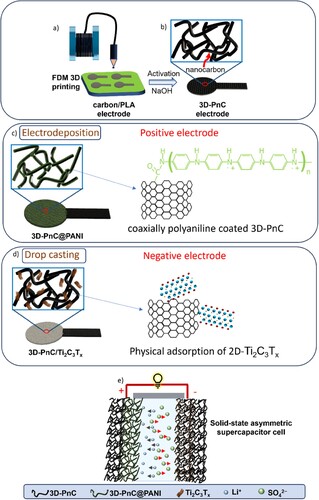
3.1. Negative electrode
The negative electrode was prepared by drop casting of Ti3C2Tx on chemically and electrochemically activated 3D-PnC. Prior to drop casting, Ti3C2Tx powder was sonicated to delaminate the 2D layers for better electrochemical performance [Citation43]. The SEM images of Ti3C2Tx before and after delamination are shown in Figures S1a,b, respectively. The delamination opens up the layers of Ti3C2Tx separation as expected from the sonication process. The SEM images of blank 3D-PnC and 3D-PnC/Ti3C2Tx deposited are shown in a,b, respectively. Energy dispersive X-ray (EDX) analysis was used to confirm the elemental composition of 3D-PnC/Ti3C2Tx. The presence of Ti, C, and F was found as anticipated in EDX mapping, as shown in d−g. Based on the EDX spectra analysis, the atomic percentages Ti, C, F, and O are found to be 77.28, 20.65, 9.49, 1.15 and 0.92%, respectively (Figure S2a).
Figure 2. Scanning electron microscopy (SEM) image of (a) 3D-PnC and (b) Ti3C2Tx deposited 3D-PnC, (c) SEM-energy dispersive X-ray (EDX) spectroscopy mapping area and (d) overlay and (e-g) elemental mapping of Ti, C, and F, respectively of 3D-PnC/Ti3C2Tx. Scale (a, b) = 5 µm and (c – f) = 8 µm.

The XRD analysis of the Ti3C2Tx was carried out to understand the crystallographic structure, as shown in a. The peaks acquired from the 3D-PnC were well-matched with the Ti3C2Tx reported [Citation44]. The intense characteristic peaks at 2θ of 7.9° correspond to the (002) plane. Furthermore, the bonding environment and elemental compositions were assessed using XPS. The survey spectrum reveals the presence of significant Ti 2p, C 1s, F 1s, and O 1s peaks, b. The concentration of F 1s in Ti3C2Tx shows that F was functionalised from the HF treatment, as observed in the EDX analysis. The high-resolution core-level spectra were studied for a better understanding of the bonding states of the component elements. The core level Ti 2p spectrum of Ti3C2Tx (c) shows doublet peaks at 454.6 and 460.6 eV corresponding to Ti 2p3/2 and Ti 2p1/2, respectively of the Ti-C carbide bond of Ti3C2Tx [Citation45, Citation46]. The four pairs of peak at 455.4/461.1, 456.9/462.6, 459.5/465.2, and 460.3/466.0 eV correspond to the Ti 2p3/2 and Ti 2p1/2 peaks of the Ti(II), Ti(III), TiO2-xFx, and TiFx, respectively [Citation47–50]. The core level C 1s spectrum of Ti3C2Tx exhibits five peaks located at 281.7, 284.8, 286.4, 288.7, and 284.6 eV corresponding to the C-Ti-Fx, C–C (sp2), C–C (sp3), C–O, and C=O, respectively, as depicted in d [Citation31, Citation51, Citation52]. Furthermore, the high-resolution spectra of F 1s contain two peaks at 684.9 and 686.4 eV, which are of C-Ti-Fx and C-Ti2-Fx, respectively (Figure S3) [Citation53].
Figure 3. Materials characterisation of Ti3C2Tx (a) X-ray diffraction pattern (XRD) of Ti3C2Tx, X-ray photoelectron spectroscopy (XPS), (b) survey spectra, and high-resolution core-level spectra of (c) Ti 2p (d) C 1s of Ti3C2Tx.
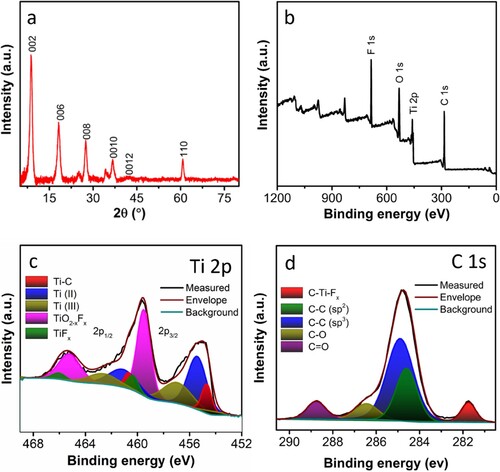
Electrochemical measurements were carried out in a three-electrode system using Pt as the counter electrode and Ag|AgCl as the reference electrode in 1 M Li2SO4. The CV analysis was carried out from 10 to 100 mV s−1, showing an increase in current density with the scan rate, and the electrode offered good rate capability. The voltammogram shows a distorted rectangular pattern which points towards the pseudocapacitive and electric double-layer charge storage mechanism of the electrode. The electrochemical enhancement of 3D-PnC/Ti3C2Tx can be attributed to the.
The galvanostatic charge–discharge (GCD) study was carried out for the 3D-PnC/Ti3C2Tx in the same voltage window of CV measurement from −0.5 to +0.3 V, as presented in b. The charging and discharging curves were similar and displayed a triangular pattern, proving the predominant nature of double-layer capacitance. The 3D-PnC/Ti3C2Tx displayed an areal capacitance of 39.5 mF cm−2 at the current density of 0.51 mA cm−2. Furthermore, the high electrical conductivity of Ti3C2Tx improves the overall conductivity of the electrode material. This facilitates efficient charge transfer between the electrode and the electrolyte, reducing internal resistance and improving the electrochemical performance of the supercapacitor. Cyclic stability tests were carried out in a three-electrode system, demonstrating a notable capacitance retention of 81.6% after 8000 charge–discharge cycles (Figure S8).
3.2. Positive electrode
The positive electrode was fabricated by electrodeposition of PANI on the 3D-PnC. Electrodeposition was carried out by running multiple cycles of CV in a KCl-based aniline solution. The following cyclic deposition CV is shown in supporting information in Figure S4b. The SEM images of 3D-PnC and 3D-PnC@PANI show a coaxial deposition of polyaniline on top of carbon nanotubes, as observed in a,b. The PANI deposition was optimised by doing deposition of cycles 5, 10, 20, and 40. As shown in Figure S4d, the CV comparison at 50 mV s−1 demonstrated that 20 cycles of deposited electrodes were the most effective. All further research was conducted on a 20-cycles PANI deposited 3D-PnC. The average diameter of the carbon nanotubes is shown to have risen by around 10 nm as a result of this coaxial deposition of PANI is measured from the SEM image in b. The EDX elemental mapping for the C and N elements is shown in d,e, respectively, for the scan area in c, which confirms again the deposition of PANI.
Figure 5. Scanning electron microscopy (SEM) images of (a) 3D printed nanocarbon (3D-PnC) and (b) 3D-PnC@PANI electrode (c) SEM-energy dispersive X-ray (EDX) spectroscopy mapping area and (d,e) elemental mapping of C and N, respectively of PANI. scale (a, b) = 300 nm and (c–e) = 800 nm.
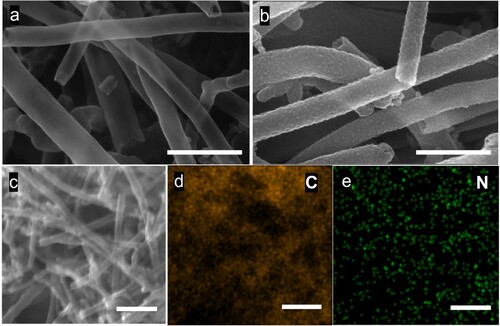
Furthermore, XPS analysis of the 3D-PnC@PANI was carried out to study the bonding environment and elemental composition. The survey spectrum of 3D-PnC@PANI can be observed in a. From the survey spectra, the presence of N, C, Ti and O was observed. The presence of Ti can be attributed to the inherent impurity in 3D-PnC. High-resolution core-level spectra are used for detailed analysis. The N 1s spectra of 3D-PnC@PANI can be resolved into four peaks associated with quinoid imine, benzenoid imine, positively charged imine (bipolaron state), and protonated amine (polaron state), centred at 398.8, 399.5, 400.8, and 402.2 eV, respectively (b) [Citation54, Citation55]. High-resolution spectra of C 1s shown in c reveal the presence of four different types of carbon functional groups, that is, nonoxygenated carbon (C−C) at 284.6 eV, oxygenated carbon (C=O) in the range 288 eV, oxygenated carbon (C−O) in the range 286.8 eV and nitrogenated carbon (C−N) near 285.5 eV. In addition, the O 1s spectrum of 3D-PnC@PANI is shown in d. The peak at the binding energy of 530.5 eV represents C=O. The peak at 532.1 eV is assigned to the hydroxyl (C−OH) and ether (C−O−C) group peak at 533.0 eV.
Figure 6. X-ray photoelectron spectroscopy. (a) survey spectra high-resolution core-level spectra of (b) N 1s, (c) C 1s, (d) O 1s of 3D-PnC@PANI.
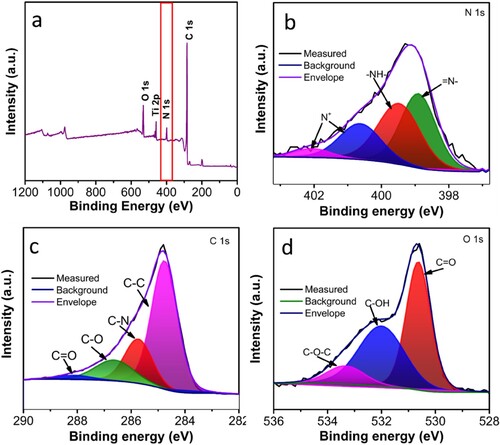
Electrochemical measurements of the 3D-PnC@PANI positive electrode were also carried out in a three-electrode system using Pt as the counter electrode and Ag|AgCl (1 M KCl) as the reference electrode in 1 M Li2SO4. The CV analysis was carried out from 10 to 100 mV s−1, showing an increase in current density with the scan rate, and the electrode offered good rate capability (a). The cyclic voltammogram displayed a distorted rectangular pattern pointing to the electric double-layer prominence of the electrode.
Figure 7. Electrochemical analysis of 3D-PnC@PANI electrode. (a) Cyclic voltammetry study at different scan rates (inset: 3D-PnC@PANI electrode schematic), (b) galvanostatic charge-discharge study at various current densities 0.18, 0.25, and 0.76 mA cm−2.
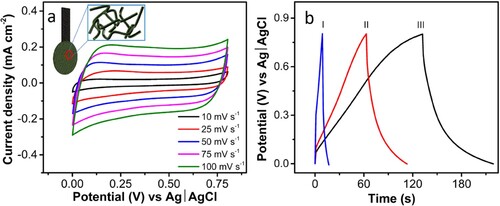
The GCD study was carried out in different current densities 0.18, 0.25, and 0.76 mA cm−2, as shown in b. The typical triangular charge–discharge curve is observed, indicating the dominance of the electric double-layer capacitance of the electrode. At different current densities of densities 0.18, 0.25, and 0.76 mA cm−2 an areal capacitance of 19.61, 15.92 and 7.64 mF cm−2 were recorded for the 3D-PnC@PANI electrode, respectively.
Electrochemical impedance analysis of the electrodes was carried out in the frequency range of 100 kHz to 0.1 Hz. The Nyquist plot of 3D-PnC@PANI, 3D-PnC/Ti3C2Tx and 3D-PnC are shown in Figure S5. In the high-frequency region, the point at Zreal (Z′) denotes the equivalent series resistance (Rs) of the electrodes, which provides insight into the current collector−electrode material contact resistance, intrinsic resistance of active material, and electrolyte resistance [Citation56]. From the EIS spectra (Figure S5), the Rs values for 3D-PnC@PANI, 3D-PnC/Ti3C2Tx and 3D-PnC electrodes are observed to be 201, 146, and 394 Ω, respectively. The subsequent deposition of PANI and MXene acts as a conductive bridge across the electrode–electrolyte interface, facilitating rapid charge transfer and enhancing overall electrochemical performance. The cyclic stability test revealed a steady drop in capacitance after the initial 700 cycles. Capacitance retention of 44.2% was obtained after 4000 charge–discharge cycles (Figure S8). This could be due to the volumetric expansion and contraction of PANI during its electrochemical doping and developing process, leading to structural alterations and mechanical deterioration, resulting in irreversible and rapid decay in capacitance throughout electrochemical cycling [Citation57–59].
3.3. Solid-State asymmetric supercapacitor (SS-ASC)
From the electrochemical analysis of individual electrodes in the three-electrode system, the voltage window for 3D-PnC/Ti3C2Tx and 3D-PnC@PANI electrode was obtained to be from +0.3 to −0.5 V (ΔV = 0.8 V) and from 0 to +1.0 V (ΔV = 1.0 V), respectively as shown in a. Seemingly, the ASC comprising of 3D-PnC/Ti3C2Tx as the negative electrode and 3D-PnC@PANI as a positive electrode can reach a higher potential window and energy density than identical electrode cells (symmetric supercapacitor). Considering this, a solid-state asymmetric supercapacitor is fabricated using Li2SO4/PVA gel electrolyte. The voltage window of the SS-ASC is optimised by carrying out CV in 50 mV s−1 at different voltage windows from 0–1 V to 0–2 V, and the consequent CVs are shown in b. From the optimisation, the 0–1.6 V window was found to be ideal, and further characterisation was carried out in this range.
Figure 8. Electrochemical analysis. (a) Cyclic voltammetry (CV) study of the 3D-PnC/Ti3C2Tx and 3D-PnC@PANI in three-electrode test system at the scan rate of 50 mV s – 1, (b) CV study of the asymmetric supercapacitor (ASC) with increasing potential window from 0–1 to 0–2 V, (c) CV study of the ASC at the potential window of 0–1.6 V with different scan rates (inset: schematic of the ASC cell), (d) galvanostatic charge-discharge (GCD) study of ASC at various current densities 0.28, 0.40, and 0.57 mA cm−2.
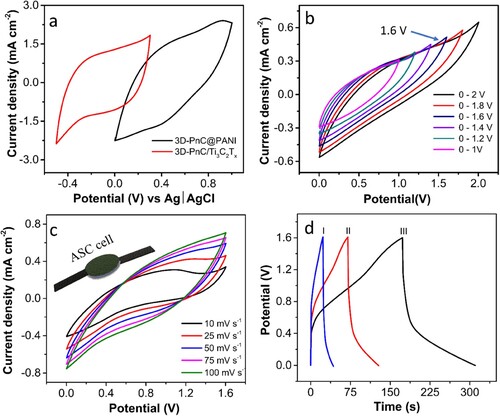
The CV study of SS-ASC was then carried out at different scan rates from 10 to 100 mV s−1 at the voltage window of 0–1.6 V, as shown in c. The CV appears to be stable under the chosen scan rate. The rate capability of the device is also reasonable, considering the increase in current density with the increase in scan rate.
GCD study was carried out at different current densities in the same potential window of 0–1.6 V (d). The charging and discharging curves showed a similar pattern. At different current densities of 0.28, 0.40, and 0.57 mA cm−2, the areal capacitance of 24.42, 14.36 and 7.78 mF cm−2, respectively and areal power density of 226.50, 317.10, and 453.00 µW cm−2, respectively were observed.
Cyclic stability test was carried out on the SS-ASC under a constant current density 0.5 mA cm−2 for 20000 cycles, and the results are shown in Figure S6. From the capacitance retention plot Figure S6a, an increment in capacitance during the initial 3000 cycles, reaching 120% of the initial capacitance, was observed. This increase in the capacitance can be attributed to the initial activation of the electrode materials that will expose the inner active sites for the electrochemical reactions [Citation41, Citation60]. In the subsequent cycles, a slow decrease in capacitance was observed, reaching 74% after 20,000 cycles, suggesting good supercapacitive features for the fabricated cell [Citation41]. Additionally, coulombic efficiency of 97% is observed even after 20,000 cycles, which indicates the excellent electrochemical reversibility of the cell (Figure S6b) [Citation61].
GCD studies were carried out in series and parallel configurations to observe the performance of the cell for real-world applications. While two- and three-cells were connected in series, the corresponding voltage was able to increase two- and three- times, respectively, that of the single cell, which is 1.6 V, keeping the original charging and discharging time (a). Similarly, the GCD of the two- and three-cells in parallel was observed. As anticipated, in the parallel configuration of two- and three-cells, the charging time was able to increase, keeping the voltage range constant. Furthermore, a 1.6 V red LED was illuminated connecting the cells in series (Video S1,S2). The devices with three cells connected in series could illuminate a red-LED for ≈2 minutes while it was charged for 1 minute.
4. Conclusion
In summary, this study demonstrates the scalable fabrication route utilising 3D-printed nanocarbon-based (3D-PnC) electrodes to fabricate positive and negative electrodes for asymmetric supercapacitor applications. We explored Ti3C2Tx MXene and polyaniline-based electrodes, integrating them with the 3D-PnC to fabricate free-standing 3D electrodes of any complex shape without using any external current collector. The solid-state asymmetric supercapacitor (SS-ASC), comprising o 3D-PnC/Ti3C2Tx as a negative electrode, 3D-PnC@PANI as a positive electrode with Li2SO4/PVA gel electrolyte demonstrates a stable potential window to 1.6 V, with a high energy density of 5.10 µWh cm−2 at the power density of 317.10 µW cm−2. The SS-ASC cell can be used to light up the LEDs while connected in series. Towards future direction, the work will pave a to explore other 2D materials, including novel MXenes and conducting polymers-based ASC for energy storage applications.
Authors contribution
S. M. carried out materials synthesis and fabrication, characterisations, and analysis and wrote the original draft. K. G. conceptualised and mentored the project from electrode fabrication to characterisations and data analysis. M. P. supervised the project. All authors contributed to the writing.
Supplemental Material
Download MS Word (4.2 MB)Acknowledgements
The work was supported by ERDF/ESF project TECHSCALE (No. CZ.02.01.01/00/22_008/0004587). This research was co-funded by the European Union under the REFRESH – Research Excellence For REgion Sustainability and High-tech Industries project number CZ.10.03.01/00/22_003/0000048 via the Operational Programme Just Transition. K. G. acknowledges the financial support of Grant Agency of the Czech Republic (EXPRO: 19-26896X). CzechNanoLab project LM2018110 funded by MEYS CR is gratefully acknowledged for the financial support of the measurements/sample fabrication at CEITEC Nano Research Infrastructure.
Disclosure statement
No potential conflict of interest was reported by the author(s).
Data availability statement
The data that support the findings of this study are available from the corresponding author, M. P., upon reasonable request.
Additional information
Funding
References
- Zhou Y, Qi H, Yang J, et al. Two-birds-one-stone: multifunctional supercapacitors beyond traditional energy storage. Energy Environ Sci. 2021;14:1854–1896. doi:10.1039/D0EE03167D
- Lakshmi KCS, Vedhanarayanan B. High-performance supercapacitors: a comprehensive review on paradigm shift of conventional energy storage devices. Batteries. 2023;9(4):202. doi:10.3390/batteries9040202
- Zhang C, Ma Y, Zhang X, et al. Two-dimensional transition metal carbides and nitrides (MXenes): synthesis, properties, and electrochemical energy storage applications. Energy Environ Mater. 2020;3(1):29–55. doi:10.1002/eem2.12058
- Wang H, Wang Y, Chang J, et al. Nacre-inspired strong MXene/cellulose fiber with superior supercapacitive performance via synergizing the interfacial bonding and interlayer spacing. Nano Lett. 2023;23(12):5663–5672. doi:10.1021/acs.nanolett.3c01307
- Yun Q, Li L, Hu Z, et al. Layered transition metal dichalcogenide-based nanomaterials for electrochemical energy storage. Adv Mater. 2020;32:1903826.
- Zhang Y, Li L, Su H, et al. Binary metal oxide: advanced energy storage materials in supercapacitors. J Mater Chem A. 2015;3:43–59. doi:10.1039/C4TA04996A
- Xia W, Mahmood A, Zou R, et al. Metal-organic frameworks and their derived nanostructures for electrochemical energy storage and conversion. Energy Environ Sci. 2015;8:1837–1866. doi:10.1039/C5EE00762C
- Han R, Liu F, Wang X, et al. Functionalised hexagonal boron nitride for energy conversion and storage. J Mater Chem A. 2020;8:14384–14399. doi:10.1039/D0TA05008C
- Jia Z, Cheng C, Chen X, et al. Applications of all-inorganic perovskites for energy storage. Mater Adv. 2023;4:79–104. doi:10.1039/D2MA00779G
- Dong W, Xie M, Zhao S, et al. Materials design and preparation for high energy density and high power density electrochemical supercapacitors. Mater Sci Eng R Rep. 2023;152:100713. doi:10.1016/j.mser.2022.100713
- Mouchani P, Sarraf-Mamoory R, Aghajani H, et al. Low mass loading of graphene quantum dots on titanium nitride nanotube arrays for boosting capacity and operating voltage of symmetric supercapacitor in an aqueous electrolyte. J Energy Storage. 2023;73:108858. doi:10.1016/j.est.2023.108858
- Khudiyev T, Lee JT, Cox JR, et al. 100 m long thermally drawn supercapacitor fibers with applications to 3D printing and textiles. Adv. Mater. 2020;32:2004971. doi:10.1002/adma.202004971
- Bozkurt Y, Karayel E. 3D printing technology; methods, biomedical applications, future opportunities and trends. Mater Res Technol. 2021;14:1430–1450. doi:10.1016/j.jmrt.2021.07.050
- Nichols MR. How does the automotive industry benefit from 3D metal printing? Met Powder Rep. 2019;74(5):257–258. doi:10.1016/j.mprp.2019.07.002
- Sachyani Keneth E, Kamyshny A, Totaro M, et al. 3D printing materials for soft robotics. Adv Mater. 2021;33:2003387. doi:10.1002/adma.202003387
- Rezende IHWS, Semaan FS, Borges LEP, et al. Energy devices and defense: metal oxides and supercapacitors. In: Á Rocha, CH Fajardo-Toro, JMR Rodríguez, editor. Developments and advances in defense and security. Singapore: Springer Singapore; 2022. p. 241–248.
- Lakkala P, Munnangi SR, Bandari S, et al. Additive manufacturing technologies with emphasis on stereolithography 3D printing in pharmaceutical and medical applications: a review. Int J Pharm. 2023;5:100159.
- Joseph TM, Kallingal A, Suresh AM, et al. 3D printing of polylactic acid: recent advances and opportunities. Int J Adv Manuf Technol. 2023;125:1015–1035. doi:10.1007/s00170-022-10795-y
- Bodaghi M, Sadooghi A, Bakhshi M, et al. Glass fiber reinforced acrylonitrile butadiene styrene composite gears by FDM 3D printing. Adv Mater Interfaces. 2023;10:2300337. doi:10.1002/admi.202300337
- Wojtyła S, Klama P, Baran T. Is 3D printing safe? Analysis of the thermal treatment of thermoplastics: ABS, PLA, PET, and nylon. J Occup Environ Hyg. 2017;14(6):D80–D85. doi:10.1080/15459624.2017.1285489
- Gnanasekaran K, Heijmans T, van Bennekom S, et al. 3D printing of CNT- and graphene-based conductive polymer nanocomposites by fused deposition modeling. Appl Mater Today. 2017;9:21–28. doi:10.1016/j.apmt.2017.04.003
- Guo H, Lv R, Bai S. Recent advances on 3D printing graphene-based composites. Nano Mater Sci. 2019;1(2):101–115. doi:10.1016/j.nanoms.2019.03.003
- Mello G, Pinto E, Ponzio EA, et al. Graphite: sailing in a cost-effective electron sea. In: Campbell QC, editor. Graphite properties occurrencesand uses. New York: Nova Science Publishers, Inc; 2013. p. 189–214.
- Tan HW, Choong YY, Kuo CN, et al. 3D printed electronics: processes, materials and future trends. Progr Mater Sci. 2022;127:100945. doi:10.1016/j.pmatsci.2022.100945
- Mappoli S, Ghosh K, Pumera M. Integrated free-standing WS2 3D-printed carbon supercapacitor with solid state electrolyte. Virtual Phys Prototyp. 2024;19(1):1–10.
- Ghosh K, Pumera M. Free-standing electrochemically coated MoS x based 3D-printed nanocarbon electrode for solid-state supercapacitor application †. Nanoscale. 2021;13(11):5744–5756. doi:10.1039/D0NR06479C
- Barbosa J, Amorim P, Gonçalves M, et al. Evaluation of 3D printing parameters on the electrochemical performance of conductive polymeric components for chemical war … evaluation of 3d printing parameters on the electrochemical performance of conductive polymeric components for chemical warfare agent sensing developments and advances in defense and security; 2019; p. 425−435.
- Ghosh K, Ng S, Iffelsberger C, et al. Inherent impurities in graphene/polylactic acid filament strongly influence on the capacitive performance of 3D-printed electrode. Chem Eur J. 2020;26(67):15746–15753. doi:10.1002/chem.202004250
- Redondo E, Muñoz J, Pumera M. Green activation using reducing agents of carbon-based 3D printed electrodes: turning good electrodes to great. Carbon N Y. 2021;175:413–419. doi:10.1016/j.carbon.2021.01.107
- Richter EM, Rocha DP, Cardoso RM, et al. Complete additively manufactured (3D-printed). Electrochem Sensing Platform Anal Chem. 2019;91(20):12844–12851.
- Ghosh K, Pumera M. Mxene and MoS3−x coated 3D-printed hybrid electrode for solid-state asymmetric supercapacitor. Small Methods. 2021;5(8):2100451. doi:10.1002/smtd.202100451.
- Mohammadi AV, Rosen J, Gogotsi Y. The world of two-dimensional carbides and nitrides (MXenes). Science. 2021;372(6547):eabf1581. doi:10.1126/science.abf1581
- Xu X, Zhang Y, Sun H, et al. Progress and perspective: MXene and MXene-based nanomaterials for high-performance energy storage devices. Adv Electron Mater. 2021;7:2000967. doi:10.1002/aelm.202000967
- Ahouei MA, Syed TH, Bishop V, et al. Ti3C2Tx MXene framework materials: preparation, properties and applications in energy and environment. Catal Today. 2023;409:162–172.
- Heme HN, Alif MSN, Rahat SMSM, et al. Recent progress in polyaniline composites for high-capacity energy storage: a review. J. Energy Storage. 2021;42:103018. doi:10.1016/j.est.2021.103018
- Ghosh K, Yue CY, Sk MM, et al. Development of 3D urchin-shaped coaxial manganese dioxide@polyaniline (MnO2@PANI) composite and self-assembled 3D pillared graphene foam for asymmetric all-solid-state flexible supercapacitor application. ACS Appl Mater Interfaces. 2017;9(18):15350–15363. doi:10.1021/acsami.6b16406
- Ghosh K, Yue CY, Sk MM, et al. Development of a 3D graphene aerogel and 3D porous graphene/MnO2 @polyaniline hybrid film for all-solid-state flexible asymmetric supercapacitors. Sustain Energy Fuels. 2018;2(1):280–293. doi:10.1039/C7SE00433H
- Bhadra S, Singha NK, Khastgir D. Electrochemical synthesis of polyaniline and its comparison with chemically synthesized polyaniline. J Appl Polym Sci. 2007;104(3):1900–1904. doi:10.1002/app.25867
- Li N, Xiao Y, Xu C, et al. Facile preparation of polyaniline nanoparticles via electrodeposition for supercapacitors. Int J Electrochem Sci. 2013;8(1):1181–1188. doi:10.1016/S1452-3981(23)14090-9
- Zhang H, Cao G, Wang W, et al. Influence of microstructure on the capacitive performance of polyaniline/carbon nanotube array composite electrodes. Electrochim Acta. 2009;54(4):1153–1159. doi:10.1016/j.electacta.2008.09.004
- Chodankar NR, Dubal DP, Kwon Y, et al. Direct growth of FeCo2O4 nanowire arrays on flexible stainless steel mesh for high-performance asymmetric supercapacitor. NPG Asia Mater. 2017;9(8):1–10. doi:10.1038/am.2017.145
- Shao Y, El-Kady MF, Sun J, et al. Design and mechanisms of asymmetric supercapacitors. Chem Rev. 2018;118(18):9233–9280. doi:10.1021/acs.chemrev.8b00252
- Malaki M, Maleki A, Varma RS. MXenes and ultrasonication. J Mater Chem A. 2019;7(18):10843–10857. doi:10.1039/C9TA01850F
- Alhabeb M, Maleski K, Anasori B, et al. Guidelines for synthesis and processing of two-dimensional titanium carbide (Ti3C2Tx MXene). Chem Mater. 2017;29(18):7633–7644. doi:10.1021/acs.chemmater.7b02847
- Wang Q, Mei Y, Zhou R, et al. Persulfate activation of CuS@Ti3C2-based MXene with Bi-active centers toward orange II removal under visible light. Colloids Surf A: Physicochem Eng Asp. 2022;648:129315. doi:10.1016/j.colsurfa.2022.129315
- Xu S, Liu C, Jiang X, et al. Ti3C2 MXene promoted Fe3+/H2O2 fenton oxidation: comparison of mechanisms under dark and visible light conditions. J Hazard Mater. 2023;444:130450. doi:10.1016/j.jhazmat.2022.130450
- Wei H, Dong J, Fang X, et al. Ti3C2Tx MXene/Polyaniline (PANI). Ti3C2Tx MXene/Polyaniline (PANI). Sandwich intercalation structure composites constructed for microwave absorption. Compos Sci Technol. 2019;169:52–59.
- Al Mayyahi A, Sarker S, Everhart M, et al. Synthesis of ultrathin, nano-sized Ti3C2Tx with abundant = O and –OH terminals and high transparency as a cocatalyst: enabling design of high-performance titania-Ti3C2Tx hybrid photocatalysts. J Phys Chem Solids. 2022;170:110875), doi:10.1016/j.jpcs.2022.110875
- Peng Y, Zhang Y, Deng L, et al. Electrostatic self-assembly of Ti3C2Tx/Fe nanochain hybrids for efficient microwave absorber. J Magn Magn Mater. 2023;570:170536.
- Cao LCT, Jong CA, Hsu SH, et al. A simple approach to MXene micropatterning from molecularly driven assembly. ACS Omega. 2021;6(51):35866–35875. doi:10.1021/acsomega.1c06662
- Pan Z, Cao F, Hu X, et al. A facile method for synthesizing CuS decorated Ti3C2 MXene with enhanced performance for asymmetric supercapacitors. J Mater Chem A Mater. 2019;7(15):8984–8992. doi:10.1039/C9TA00085B
- Zhi W, Xiang S, Bian R, et al. Study of MXene-filled polyurethane nanocomposites prepared via an emulsion method. Compos Sci Technol. 2018;168:404–411. doi:10.1016/j.compscitech.2018.10.026
- Lu W, Mustafa B, Wang Z, et al. PDMS-encapsulated MXene@Polyester fabric strain sensor for multifunctional sensing applications. J Nanomater. 2022;12(5):871. doi:10.3390/nano12050871
- Kim M, Lee C, Jang J. Fabrication of highly flexible, scalable, and high-performance supercapacitors using polyaniline/reduced graphene oxide film with enhanced electrical conductivity and crystallinity. Adv Funct Mater. 2014;24(17):2489–2499. doi:10.1002/adfm.201303282
- Liu F, Luo S, Liu D, et al. Facile processing of free-standing polyaniline/SWCNT film as an integrated electrode for flexible supercapacitor application. ACS Appl Mater Interfaces. 2017;9(39):33791–33801. doi:10.1021/acsami.7b08382
- Sk MM, Yue CY. Synthesis of polyaniline nanotubes using the self-assembly behavior of vitamin C: a mechanistic study and application in electrochemical supercapacitors. J Mater Chem A Mater. 2014;2(8):2830–2838. doi:10.1039/C3TA14309K
- Sun Z, Zhang J, Ye F, et al. Vulcanization treatment: an effective way to improve the electrochemical cycle stability of polyaniline in supercapacitors. J Power Sources. 2019;443:227246. doi:10.1016/j.jpowsour.2019.227246
- Cong HP, Ren XC, Wang P, et al. Flexible graphene-polyaniline composite paper for high-performance supercapacitor. Energy Environ Sci. 2013;6(4):1185–1191. doi:10.1039/c2ee24203f
- Wang K, Wu H, Meng Y, et al. Conducting polymer nanowire arrays for high performance supercapacitors. Small. 2014;10(1):14–31. doi:10.1002/smll.201301991
- Ji SH, Chodankar NR, Kim DH. Aqueous asymmetric supercapacitor based on RuO2-WO3 electrodes. Electrochim Acta. 2019;325:134879.
- Ji SH, Chodankar NR, Jang WS, et al. High mass loading of H-WO3 and α-MnO2 on flexible carbon cloth for high-energy aqueous asymmetric supercapacitor. Electrochim Acta. 2019;299:245–252.