ABSTRACT
This study aimed to report forces and moments delivered by 3D-printed aligners. Tera Harz TC-85 resin and Zendura FLX (ZF) thermoformed sheets (0.76 mm) were used. Rectangular strip specimens (50 × 10 × 0.6 mm) were produced for a three-point bending test (3PB) at different temperatures. Full anatomical aligners were produced for 3D force measurements on different teeth and directions at 37 °C, using a custom-made device. ZF exhibited flexural forces between 1.7 and 2.3 N, while TC-85 from 0.3 N to 2.7 N, with temperature affecting TC-85 more significantly. At lower temperatures, TC-85 was stiffer than ZF, but at higher temperatures, it became less rigid. The 3D-force measurements indicated comparable values for both materials. Force ranged from 0.3–1.7 N and moment from 4.8–9.4 Nmm. The study concluded that TC-85 3D-printed aligners deliver biologically compatible forces for orthodontic movement and offer better control over thickness.
The 3D-printed Tera Harz TC-85 resin revolutionises aligner production.
Tera Harz TC-85 aligners generate forces within the orthodontic accepted range.
Shape recovery of TC-85 can enable adaptability and larger incremental step size.
The 3D printing offers greater control over aligner design and thickness.
Highlights
Introduction
Orthodontic clear aligners have emerged as an increasingly attractive, hygienic, and more comfortable alternative to traditional braces [Citation1]. Moreover, a reduced risk of root resorption with orthodontic aligner treatment was reported [Citation2]. They apply forces to teeth based on predetermined movements in a virtual model, typically ranging from 0.2–0.4 mm in translation and 2–3 degrees in rotation [Citation3]. While the sequential orthodontic treatment system associated with aligners has proven to deliver effective tooth movement, the increased number of splints per treatment raises the risk of deviation between the intended treatment outcomes and the actual clinical results [Citation4]. Additionally, it escalates the financial burden per treatment and substantially increases plastic consumption, posing a significant environmental concern [Citation5].
The biomechanical behaviour of aligners is controlled by multiple factors, such as aligner thickness [Citation6–8], extension and trimming line design [Citation8–10], the amount of activation [Citation8, Citation11], and the use of auxiliaries [Citation12, Citation13]. However, the aligner materials remain one of the most essential variables associated with mechanical and clinical efficiency [Citation14, Citation15]. Conventional thermoformed aligners are made of a single layer of plastic sheets made of different types of polymers, such as polyethylene terephthalate glycol (PETG) or thermoformed polyurethane (TPU) [Citation14, Citation16–18] Moreover, multi-layer hybrid materials have been introduced to overcome the limitations of single-layer materials, providing enhanced mechanical properties and greater patient comfort [Citation19].
Shape memory polymers (SMPs) have been introduced recently as an innovative aligner material that can overcome the limited aligner step size, by replacing several conventional aligners with a single aligner of SMPs [Citation5, Citation18, Citation20]. SMPs can effectively maintain a temporary shape until they encounter an appropriate stimulus, at which point they promptly return to their original form [Citation21, Citation22]. Hence, they show the capacity to achieve higher strain levels and can adopt multiple temporary shapes [Citation23]. Their shape memory mechanism involves two key components: A stable polymer network that controls the initial shape, and a reversible polymer network that enables temporary shape recovery [Citation21, Citation24]. The current concept involves utilising the shape recovery of this material to apply sustained force to teeth over a wide range of movements [Citation18, Citation20].
The rising popularity of clear aligner therapy in orthodontic care has provoked advancements in intraoral scanning, design software, and three-dimensional (3D) printing technologies [Citation25]. Traditionally, orthodontic aligners were crafted using thermoforming, a widely used technique in orthodontics for making appliances like retainers and aligners. This method involves heating a thermoplastic material until it softens, then shaping it over a dental model. After cooling and hardening, the material retains the molded shape, resulting in a custom-fitted appliance [Citation26]. The traditional thermoforming process is negatively affecting the material's mechanical properties [Citation27, Citation28]. Thus, as an alternative strategy, direct 3D printing of aligners has recently been introduced [Citation29, Citation30]. The 3D printing procedure eliminates the cumulative errors associated with the conventional thermoforming workflow. Besides, 3D printing provides improved precision, greater control over aligner design and thickness, reduced complexity in the supply chain, decreased material wastage, and lower production costs [Citation31, Citation32]. This development highlights the importance of evaluating the efficiency and precision of these materials in applying orthodontic forces [Citation33].
The emergence of 3D-printed resin into the aligner orthodontic market has sparked a transformative shift in the field. However, there remains a need for comprehensive investigations, especially in areas critical to clinicians. Among these areas, the examination of forces and moments applied to different teeth during various directional movements is vital. In this regard, our research team is conducting an extensive project to explore this advancement on various levels, including their physical and mechanical characteristics [Citation20], biocompatibility, geometric precision, and force dynamics. In the current experimental study, the primary objective was to present data on the forces and moments exerted by the first photocurable 3D-printed aligners launched in the market (Tera Harz TC-85; Graphy, Seoul, Republic of Korea) on different teeth of the upper jaw under varying temperature conditions. These findings were compared to those of a commonly used conventional thermoformed aligner (Zendura FLX; Bay Materials, Fremont, CA, USA).
Materials and methods
Two materials were used in the current study: One 3D-printed material, namely Tera Harz TC-85 (TC-85), and one thermoformed material as a control, namely Zendura FLX (ZF) (). The testing specimens were produced in two configurations: rectangular strips (for a three-point bending test) and full anatomical aligners (for 3D force measurements) ().
Figure 1. Schematic diagram illustrating the workflow employed to produce specimens (Rectangular Strips or Full Anatomical Aligners) in the present study, using either the thermoforming process or 3D printing.
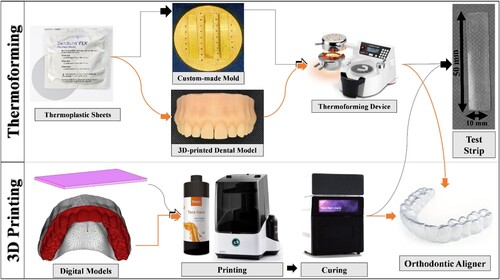
Table 1. Data on the investigated aligner materials.
To produce the rectangular strips, thermoplastic sheets of ZF underwent a thermoforming process over a custom-made mold per the manufacturer's guidelines outlined in . This thermoforming process led to the thinning of the sheets from 0.75 mm to approximately 0.6 mm [Citation34], confirmed with a digital calliper (Fisher Scientific International Inc., Hampton, NH, USA). Using scissors, the resulting specimens were subsequently cut into rectangular strips measuring 50 × 10 × 0.6 mm, with the edges refined using a polishing machine. In contrast, TC-85 specimens were directly 3D-printed in a rectangular strip form with dimensions of 50 × 10 × 0.6 mm using 3D image processing and editing software (3-matic 16.0; Materialise, Leuven, Belgium) ().
The production of anatomical aligners involved utilising a digital model of a maxillary arch created from a 3D data set (Digimation Corp., St Rose, Louisiana, USA). This model was exported as an STL file and processed in two ways. First, it was sent to a digital light processing (DPL) 3D printer (P20+; Straumann AG, Basel, Switzerland), where it was printed using P pro resin (Straumann AG, Basel, Switzerland). This resin model was used in the thermoforming process to fabricate the ZF sheets. On the other hand, the digital model was employed to design full anatomical aligners in 3-matic software, 3D-printed from TC-85, following the specified conditions outlined in . In total, eighteen aligners were produced for each tested material. Each aligner featured a straight trimming line precisely positioned 2 mm above the gingival line (). A skilled technician executed the thermoforming and trimming processes to ensure standardisation across the specimens.
In the current study, two types of tests were conducted: A three-point bending test (3PB) and a 3D force measurements test using a custom-made device (Orthodontic Measurement and Simulation System, OMSS; Oral Technology, University Hospital Bonn, Bonn, Germany) (). OMSS is a biomechanical setup that consists of a measuring unit, coupled to a sensor, that can replicate 3D tooth movements while measuring force/deflection or moment/rotation ratios. Moreover, it is enclosed in a temperature-controlled dry chamber, allowing the tests to be conducted at different temperatures [Citation35, Citation36].
Figure 2. The orthodontic measurement and simulation system (OMSS) was configured in two ways. The upper setup was employed to assess the maximum flexural strength through a 3-point bending test. Meanwhile, the lower configuration was utilised to gauge the force and moment exerted by orthodontic aligners on an individual separated tooth, connected to a sensor.
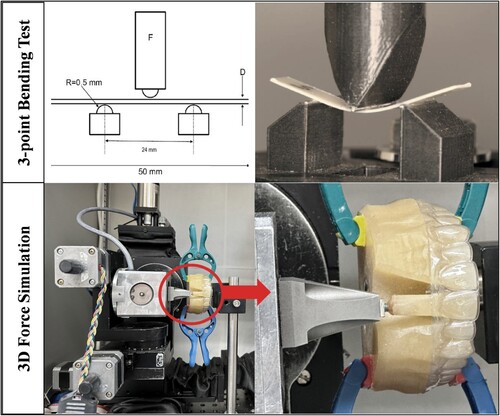
For the 3PB test by OMSS, the device was adjusted at a three-point configuration with a span length of 24 mm and a consistent rate of 5 mm/min (). A vertical deflection of 2 mm was applied to each specimen, and the maximum force at the maximum deflection was subsequently measured. This test was conducted at 20 °C, 25 °C, 30 °C, 37 °C, and 40 °C, with a permissible temperature deviation of ±0.5 °C. For each material, a total of 30 specimens were prepared, with six specimens assigned to each temperature (n = 6). The test involved two cycles, each of which involved loading and unloading. The force/deflection curves from these two cycles were recorded and analyzed.
For force and moment measurements by OMSS, three different models (duplicates of the model used for thermoforming) were made from a self-cure resin (Technovit 4004; Kulzer, Hanau, Germany). As shown in , in each model, one targeted tooth was made movable apart from the model, namely the upper right central incisor (Tooth 11), the upper right canine (Tooth 13), and the upper right second premolar (Tooth 15), and the neighbouring teeth were slightly ground proximally for smooth insertion of the tooth without resistance. The movable tooth was then affixed to the measuring unit sensor, while the model was securely fixed with the occlusal plane set parallel to the sensor axis. This setup ensured the tooth was neutrally positioned in the dental arch, and no forces or moments were applied to the tooth in its initial position ().
The eighteen aligners of each material were divided into three groups (n = 6) based on the type of the tested tooth. For each aligner, the measuring unit with the tooth was moved in the X – (intrusion/extrusion) and Y – (oro-vestibular translation) axes by ±0.4 mm in 0.01 mm increments and rotated around the X-axis (rotation around the tooth axis) by ±4° in both directions in 0.1° increments, where positive (+) represents intrusion, oral, mesial movement, and vice versa. The simulations were conducted in dry conditions and at a constant temperature of 37 °C, which reflects the normal oral temperature. The OMSS was preheated to ensure thermoregulation of the testing heat chamber and minimise destabilised temperature during testing. Additionally, the aligners and the typodont, with the removable tooth, were pre-placed in the preheated OMSS chamber for consistent conditions. Force/translation and moment/rotation curves were recorded for each measurement. Following each measurement, the setup's adjustment was re-checked and corrected if necessary.
Statistical analysis
Calculation of sample size was performed using software G*Power (version 3.1, Duesseldorf, Germany) at a significance level of 0.05 and a study power of 80%. Effect size was derived based on the data from Hahn et al. [Citation37]. The minimum sample size was four per group, but the present study used six samples (n = 6) [Citation38].
The primary data for each group underwent analysis to determine the maximum values for force and/or moment. The normal distribution of all data was assessed using the Kolmogorov-Smirnov test, which confirmed that all data followed a normal distribution. Thus, mean and standard deviation were calculated, and Student’s t-test (two-sided, unpaired) for normally distributed data was employed. To account for multiple tests, a Bonferroni correction was applied. A difference was considered significant if the p-value was less than 0.05. Mean values and standard deviations were presented, and the results were depicted in bar graphs to visualise the changes in force and/or moment for each group. Statistical analyses were conducted using Microsoft Excel 2016 (Microsoft Corporation, Redmond, WA, USA), utilising both its built-in statistical functions and custom routines.
Results
As illustrated in , the 3-point bending (3PB) test conducted at different temperatures from 20 °C to 40 °C revealed that the mean maximum flexural force values for Zendura FLX (ZF) consistently fell within a narrow range of 1.7 N to 2.3 N. However, the mean maximum flexural force values for Tera Harz TC-85 (TC-85) spanned a broader range of 0.3 N to 2.7 N. Within the testing conditions, TC-85 exhibited temperature-dependent responses. Specifically, the flexural force notably decreased with rising temperature. This was in contrast with ZF, which exhibited less sensitivity to temperature within the specified range. At lower temperatures (20 °C and 25 °C), TC-85 demonstrated higher force values than ZF, indicating greater rigidity at room temperature. Conversely, at higher temperatures (37 °C and 40 °C), TC-85 exhibited lower values of maximum flexural force falling within the range of 0.3 N to 0.9 N at oral temperature. ZF specimens maintained consistent force levels over the two testing cycles, whereas TC-85 exhibited a decrease in force during the second cycle, indicative of its plastic behaviour within the specified temperature range.
Figure 3. The maximum flexural force measured over two cycles of a 2 mm deflection for two different aligner materials, Zendura FLX (right) and Tera Harz TC-85 (left), at various temperatures.
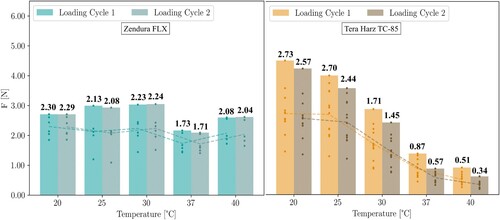
As shown in of the force/moment measurements at 37 °C, both materials exhibited generally comparable values, although significant differences were observed in certain directions of movement, but with no discernible trend. The force/moment values exhibited minor variations between different investigated teeth, with more significant differences observed in the oro-vestibular translation. In addition, the force/moment values showed a directional dependence, with the force (Fx) being higher during intrusion than extrusion in all teeth, and the force (Fy) being higher during oral translation than vestibular translation in Tooth 13 and Tooth 15, and vice versa in Tooth 11. For intrusion-extrusion movements, the force (Fx) ranged from 1.2 N to 2.7 N for intrusion and 0.3 N to 0.8 N for extrusion. For oro-vestibular movements, the force (Fy) ranged from 0.6 N to 1.7 N for oral translation and 0.4 N to 1.7 N for vestibular translation. For mesiodistal rotation, the moment (Mx) ranged from 5.6 Nmm to 9.4 Nmm for mesial rotation and 4.8 Nmm to 7.0 Nmm for distal rotation.
Discussion
As an aesthetic and a more hygienic alternative to conventional braces, orthodontic clear aligners are a focus of ongoing orthodontic research designed to improve their efficiency and functionality. The thermoforming manufacturing process influences the mechanical properties of conventional aligner materials [Citation27], hence, using advanced materials with enhanced properties could greatly improve the effectiveness of aligner treatments [Citation5, Citation18]. Moreover, the sequential systems of aligner treatment may lead to lower efficacy, increased costs, and negative environmental impact [Citation4]. The restricted tooth movement per individual aligner splint leads to a higher quantity of splints required for treatment. This escalation not only raises the financial cost per treatment but also substantially increases plastic consumption, posing an environmental concern. Hence, the introduction of SMPs and 3D printing in aligner technology may offer a more accurate, cost-effective, and economically friendly solution [Citation5, Citation18, Citation20, Citation30, Citation39]. The primary objective of the current experimental study was to present data on the force system of the first 3D-printed aligners introduced to the market (Tera Harz TC-85).
A mechanical property of high interest, when analyzing orthodontic aligner materials, is their flexural strength during deflection. In orthodontic appliances like aligners, flexural strength is an indicator of their effectiveness for tooth movement [Citation28]. The 3-point bending test (3PB) is one method used to determine the flexure modulus and the maximum force delivered upon deflection [Citation5]. This test assesses the ability of aligners to facilitate tooth movement, based on the principle that a misaligned tooth applies deflection to the aligner while adjacent teeth serve as anchorage points, similar to the action of an archwire [Citation5, Citation28]. The current study replicated the oral translation of an upper central incisor. Hence, the distance between the supports (span length) was established at 24 mm, representing the average widths of two maxillary central incisors and one lateral incisor [Citation40].
Numerous studies [Citation5, Citation20, Citation28, Citation40, Citation41] have reported the maximum flexural force of aligner materials using the 3PB test. An orthodontic force ranging from 0.1–1.2 N is recommended in clinical conditions, depending on the specific tooth and the type of movement required [Citation42]. In the current study, the 3PB test was conducted at temperatures ranging from 20 to 40 °C. ZF exhibited flexural forces between 1.7 and 2.3 N, while TC-85 ranged from 0.3 N to 2.7 N, with temperature having a more significant impact on TC-85. Kwon et al. [Citation40] reported a force of 1.0 N for a 1.0 mm deflection on Essix aligner materials at room temperature, which aligns closely with the current results considering the 2.0 mm deflection. Furthermore, Atta et al. [Citation20] reported forces of 2.4 N for ZF and 0.4 N for TC-85 at 37 °C on a 2.0 mm deflection. This outcome slightly deviated from our findings but is still within a comparable range. Additionally, Elshazly et al. [Citation5] reported forces of 1.7 and 1.0 N for another 4D aligner material (ClearX) at 37 and 45 °C, respectively.
In line with the findings of Lee et al. [Citation39], the low flexural force of the 3D-printed aligner materials (TC-85) at oral temperature observed in the current study is more compatible with orthodontic tooth movement and achieved a superior performance to the conventional thermoformed aligner material (ZF). TC-85 consists of an aliphatic vinyl ester-urethane polymer with methacrylate functionalization, as determined by a recent ATR-FTIR study [Citation31]. In contrast, ZF is a multi-layer TPU-based polymer comprising high molecular weight copolymers with aromatic rings that show strong pi-pi stacking interactions [Citation43]. The interactions between the aliphatic polymer chains are relatively weaker than those in aromatic chains [Citation44]. Moreover, a thermosetting polymer changes from a rigid, glassy state to a more flexible, rubbery state at the glass transition temperature (Tg) [Citation45]. Below Tg, the polymer is in a glassy state, exhibiting typically high flexural strength. As the temperature approaches Tg, the polymer begins transitioning to a rubbery state, resulting in a rapid decrease in flexural force [Citation45]. Atta et al. [Citation20] reported a Tg of TC-85 at the midpoint of 42.3 °C, which started at 30.4 °C, while Tg of ZF was at the midpoint of 107.1 °C. This may explain why a force drop was observed with TC-85 during the 3PB test between 20 and 40 °C, whereas ZF exhibited minimal changes due to its temperature range being well far from its Tg.
Additionally, the minor deformation and the decrease in force observed in TC-85 material specimens during the second cycle can be attributed to its low Tg being near the testing temperature, a characteristic not demonstrated by ZF. Nevertheless, this deformity, together with the shape recovery property documented for TC-85 at oral temperature [Citation20, Citation39], can guarantee more adaptability, long-lasting efficacy, and larger incremental movement size per aligner. Thermoplastic aligner materials, which exhibit a viscoelastic nature, partially revert to their original shape when deformed over teeth, generating forces used in tooth movement [Citation4]. Therefore, the presence of shape memory properties at oral temperature can enhance the material's ability to efficiently recover its shape over extended treatment periods [Citation18, Citation26]. This is in marked contrast to the conventional aligner materials, which experience significant force decay within the initial days [Citation46].
Measuring the complex 3D system of forces and moments generated by an aligner is challenging, especially since the optimal orthodontic force varies from tooth to tooth [Citation34]. In the current study, initial force and moment were measured on three different maxillary teeth at 37 °C using a reliable custom-designed biomechanical device (OMSS) [Citation36]. Proffit et al. [Citation42] gave estimated recommendations for orthodontic force application for various tooth movements. They recommended a force of between 0.1 and 0.2 N for intrusion, 0.7 and 1.2 N for tipping and bodily movement, and 1.0 and 1.5 N for rotation control [Citation42]. In the current study, at a 0.4 mm deflection of Tooth 11, Tooth 13, and Tooth 15, the force was recorded within the range of 0.3 N to 0.8 N, for extrusion, 1.2 N to 2.7 N for intrusion, and from 0.4 N to 1.7 N for oro-vestibular translation. The mesiodistal moment at a 4° rotation ranged from 4.8 Nmm to 9.4 Nmm. The direction-dependent pattern of the force/moment generation and divergence between different teeth are likely attributable to the distinct facial and lingual morphologies of individual teeth and variations between teeth [Citation7, Citation8].
The current findings on thermoformed aligners are matching with previous studies that used similar mechanical setups. Hahn et al. [Citation7, Citation47] reported oro-vestibular forces, on a 0.15 mm deflection of a maxillary central incisor ranging from 2.7 N (oral) to 3.1 N (vestibular). Likewise, Elkholy et al. [Citation48] found that the average moment during a 15° distal rotation of a mandibular canine was 42.5 Nmm [Citation49]. Elshazly et al. [Citation46] reported moment values ranging from 13.6 Nmm (mesial rotation) to 40.0 Nmm (distal rotation) for a 2° rotation of an upper second premolar, using Zendura A material which is more rigid than Zendura FLX. Engelke et al. [Citation50] recorded moments ranging from 4.3 Nmm to 20.2 Nmm for 10° rotations of an upper central incisor. Kohda et al. [Citation51] reported forces of 2.91 N generated at a 0.5 mm movement of an upper central incisor.
There is limited comparative data in the literature regarding the force system of 3D-printed aligners fabricated from TC-85. Hertan et al. [Citation52] reported force values of 1.2 N with a 0.2 mm facio-lingual displacement of Tooth 11. Furthermore, Grant et al. [Citation53] reported a force level of 1.4 N for a 0.2 mm oro-vestibular displacement of Tooth 11, extrusive forces of 0.12 N, and a mesiodistal moment of 5.97 Nmm. These findings align with the current results and indicate that the forces produced by TC-85 aligners are compatible with the recommended orthodontic force levels.
Experimental testing of biomechanical systems has inherent limitations. In OMSS the sensor and tooth were rigidly connected, limiting the simulation of some clinical parameters such as the periodontal ligament, mastication, and soft-tissue reactions. Moreover, OMSS records the resultant force, providing limited insights into force distribution across the tooth's surface. Additionally, force/moment was measured for an isolated experimental movement of a single tooth, which is a simplified model not reflective of complex clinical cases.
An additional limitation is that the printed material underwent testing only once, which might not adequately capture its long-term response to repeated cyclic loads. Evaluating the material's durability and stability over time is essential to determine its suitability for orthodontic purposes. During functional use, each aligner undergoes intermittent thermal and mechanical stresses. Short-term mechanical stresses arise during aligner insertion and removal, while long-term stresses emanate from sustained contact between the aligners and malaligned teeth, as well as the forces exerted during teeth contact [Citation54]. These factors, together with other intraoral conditions such as salivation and humidity, were not comprehensively considered in the present study. Although these conditions have been reported to impact force generation over time with thermoformed materials [Citation46, Citation55], their effects on 3D-printed materials have not yet been documented.
Future research should explore variations in force distribution over the tooth surface and between different aligner materials. The potential influence of oral aging conditions on the physiochemical and mechanical characteristics of 3D-printed materials should also be assessed. Conducting a deflection load analysis on 3D-printed aligner materials could offer further validation of their mechanical properties, particularly their performance under varying load conditions. Further experimental studies and clinical trials are necessary for a comprehensive assessment of the performance and long-term implications of 4D aligners.
Conclusion
Compared to conventional thermoformed aligners, 3D-printed aligners using Tera Harz TC-85 demonstrate the ability to deliver orthodontically compatible forces for orthodontic tooth movement in experimental settings. Unlike thermoformed aligners, 3D-printed aligners provide improved control over thickness.
Credit authorship contribution statement
Madina Sharif: Investigation, Writing – original draft, Validation, Resources, Data curation. Christoph Bourauel: Writing – review & editing, Visualization, Supervision, Resources. Ahmed Ghoneima: Writing – review & editing, Visualization, Resources. Jörg Schwarze: Writing – review & editing, Visualization. Abdulaziz Alhotan: Writing – review & editing, Visualization, Resources. Tarek M. Elshazly: Conceptualization, Supervision, Writing – original draft, Writing – review & editing, Visualization, Validation, Resources, Data curation.
All authors have read and agreed to the published version of the manuscript.Declarations.
Compliance with ethics requirements
This article does not contain any studies with human or animal subjects.
Declaration of Generative AI and AI-assisted technologies in the writing process
During the preparation of this work, the authors used the ChatGPT AI tool to improve the readability of the English language. After using this tool, the authors reviewed and edited the content as needed and take full responsibility for the content of the publication.
Acknowledgment
The authors thank the Researchers Supporting Project Number (RSPD2024R790), King Saud University, Riyadh, Saudi Arabia.
Disclosure statement
No potential conflict of interest was reported by the author(s).
Data availability statement
Data will be made available on request.
Additional information
Funding
References
- Ashari A, Mohamed AM. Relationship of the dental aesthetic index to the oral health-related quality of life. Angle Orthod. 2016;86(2):337–342. doi:10.2319/121014-896.1
- Elhaddaoui R, Qoraich HS, Bahije L, et al. Orthodontic aligners and root resorption: a systematic review. Int Orthod. 2017;15(1):1–12.
- Moutawakil A. Biomechanics of aligners: literature review. Adv Dent Oral Heal. 2021: 13, doi:10.19080/ADOH.2020.13.555872
- Upadhyay M, Arqub SA. Biomechanics of clear aligners: hidden truths & first principles. J World Fed Orthod. 2022;11(1):12–21. doi:10.1016/j.ejwf.2021.11.002
- Elshazly TM, Keilig L, Alkabani Y, et al. Potential application of 4D technology in fabrication of orthodontic aligners. Front Mater. 2022;8:794536, doi:10.3389/fmats.2021.794536
- Liu DS, Chen YT. Effect of thermoplastic appliance thickness on initial stress distribution in periodontal ligament. Adv Mech Eng. 2015;7(4):1687814015578362.
- Hahn W, Dathe H, Fialka-Fricke J, et al. Create your own stimulus package and improve access to care. Am J Orthod Dentofac Orthop. 2009;136(1):e1–12.e7. doi:10.1016/j.ajodo.2009.05.003
- Elshazly TM, Bourauel C, Aldesoki M, et al. Computer-aided finite element model for biomechanical analysis of orthodontic aligners. Clin Oral Investig. 2023;27(1):115–124. doi:10.1007/s00784-022-04692-7
- Elshazly TM, Salvatori D, Elattar H, et al. Effect of trimming line design and edge extension of orthodontic aligners on force transmission: A 3D finite element study. J Mech Behav Biomed Mater. 2023: 105741, doi:10.1016/j.jmbbm.2023.105741
- Elshazly TM, Bourauel C, Chavanne P, et al. Numerical biomechanical finite element analysis of different trimming line designs of orthodontic aligners: an in silico study. J World Fed Orthod. 2024;13(2):65–71. doi:10.1016/j.ejwf.2024.01.001
- Min S, Hwang CJ, Yu HS, et al. Longitudinal measurements of tooth mobility following orthodontic treatment. Korean J Orthod. 2010;40(1):34–26. doi:10.4041/kjod.2010.40.1.34
- Elshazly TM, Bourauel C, Aldesoki M, et al. Effect of attachment configuration and trim line design on the force system of orthodontic aligners: a finite element study on the upper central incisor. Orthod Craniofacial Res. 2024. doi:10.1111/ocr.12779
- Elkholy F, Mikhaiel B, Repky S, et al. Effect of different attachment geometries on the mechanical load exerted by PET-G aligners during derotation of mandibular canines. J Orofac Orthop der Kieferorthopaedie. 2019;80(6):315–326. doi:10.1007/s00056-019-00193-7
- Cremonini F, Vianello M, Bianchi A, et al. A spectrophotometry evaluation of clear aligners transparency: comparison of 3D-printers and thermoforming disks in different combinations. Appl Sci. 2022;12(23):11964, doi:10.3390/app122311964
- Momtaz P. (2016). The effect of attachment placement and location on rotational control of conical teeth using clear aligner therapy.
- Thukral R, Gupta A. Invisalign: invisible orthodontic treatment-a review. J Adv Med Dent Sci Res. 2015;3(5):S42.
- Martorelli M, Gerbino S, Giudice M, et al. A comparison between customized clear and removable orthodontic appliances manufactured using RP and CNC techniques. Dent Mater. 2013;29(2):e1–e10. doi:10.1016/j.dental.2012.10.011
- Elshazly TM, Keilig L, Alkabani Y, et al. Primary evaluation of shape recovery of orthodontic aligners fabricated from shape memory polymer (a typodont study). Dent J. 2021;9(3):31, doi:10.3390/dj9030031
- Lombardo L, Martines E, Mazzanti V, et al. Stress relaxation properties of four orthodontic aligner materials: a 24-hour in vitro study. Angle Orthod. 2016;87(1):11–18. doi:10.2319/113015-813.1
- Atta I, Bourauel C, Alkabani Y, et al. Physiochemical and mechanical characterisation of orthodontic 3D printed aligner material made of shape memory polymers (4D aligner material). J Mech Behav Biomed Mater. 2023;150:106337.
- Meng H, Li G. A review of stimuli-responsive shape memory polymer composites. Polymer (Guildf). 2013;54(9):2199–2221. doi:10.1016/j.polymer.2013.02.023
- Lendlein A, Kelch S. Shape-memory polymers. Angew Chemie Int Ed. 2002;41(12):2034–2057. doi:10.1002/1521-3773(20020617)41:12<2034::AID-ANIE2034>3.0.CO;2-M
- Liu C, Qin H, Mather PT. Review of progress in shape-memory polymers. J Mater Chem. 2007;17(16):1543–1558. doi:10.1039/b615954k
- Behl M, Lendlein A. Shape-memory polymers. Mater Today. 2007;10(4):20–28. doi:10.1016/S1369-7021(07)70047-0
- Panayi NC. Directly printed aligner: aligning with the future. Turkish J Orthod. 2023;36(1):62, doi:10.4274/TurkJOrthod.2023.2023.20
- Zecca PA, Bocchieri S, Borgese M, et al. In vitro investigation of the mechanical properties of blended 3D-printing resins for orthodontic aligners: a comparison between commercial resin and nickel-titanium wire. Appl Sci. 2023;13(15):9020, doi:10.3390/app13159020
- Golkhani B, Weber A, Keilig L, et al. Variation of the modulus of elasticity of aligner foil sheet materials due to thermoforming. J Orofac Orthop der Kieferorthopädie. 2022;83(4):233–243. doi:10.1007/s00056-021-00327-w
- Ryu JH, Kwon JS, Jiang HB, et al. Effects of thermoforming on the physical and mechanical properties of thermoplastic materials for transparent orthodontic aligners. Korean J Orthod. 2018;48(5):316–325. doi:10.4041/kjod.2018.48.5.316
- Seo JH, Eghan-Acquah E, Kim MS, et al. Comparative analysis of stress in the periodontal ligament and center of rotation in the tooth after orthodontic treatment depending on clear aligner thickness – finite element analysis study. Materials (Basel). 2021;14(2):324, doi:10.3390/ma14020324
- Park SY, Choi SH, Yu HS, et al. Comparison of translucency, thickness, and gap width of thermoformed and 3D-printed clear aligners using micro-CT and spectrophotometer. Sci Rep. 2023;13(1):10921, doi:10.1038/s41598-023-36851-5
- Can E, Panayi N, Polychronis G, et al. In-house 3D-printed aligners: effect of in vivo ageing on mechanical properties. Eur J Orthod. 2022;44(1):51–55. doi:10.1093/ejo/cjab022
- Tartaglia GM, Mapelli A, Maspero C, et al. Direct 3D printing of clear orthodontic aligners: current state and future possibilities. Materials (Basel). 2021;14(7):1799, doi:10.3390/ma14071799
- Panayi N, Cha JY, Kim KB. 3D printed aligners: material science, workflow and Clinical applications. Seminars in Orthodontics; 2023;29:25–33.
- Elshazly TM, Keilig L, Salvatori D, et al. Effect of trimming line design and edge extension of orthodontic aligners on force transmission: an in vitro study. J Dent. 2022;125:104276, doi:10.1016/j.jdent.2022.104276
- Bourauel C, Drescher D, Thier M. An experimental apparatus for the simulation of three-dimensional movements in orthodontics. J Biomed Eng. 1992;14(5):371–378. doi:10.1016/0141-5425(92)90081-U
- Drescher D, Bourauel C, Thier M. Application of the orthodontic measurement and simulation system (OMSS) in orthodontics. Eur J Orthod. 1991;13(3):169–178. doi:10.1093/ejo/13.3.169
- Hahn W, Zapf A, Dathe H, et al. Torquing an upper central incisor with aligners acting forces and biomechanical principles. Eur J Orthod. 2010;32(6):607–613. doi:10.1093/ejo/cjq007
- Barbur I, Opris H, Crisan B, et al. Statistical comparison of the mechanical properties of 3D-printed resin through triple-jetting technology and conventional PMMA in orthodontic occlusal splint manufacturing. Biomedicines. 2023;11(8):2155, doi:10.3390/biomedicines11082155
- Lee SY, Kim H, Kim HJ, et al. Management of validation of HPLC method for determination of acetylsalicylic acid impurities in a new pharmaceutical product. Sci Rep. 2022;12(1):1–10. doi:10.1038/s41598-021-99269-x
- Kwon JS, Lee YK, Lim BS, et al. Force delivery properties of thermoplastic orthodontic materials. Am J Orthod Dentofac Orthop. 2008;133(2):228–234. doi:10.1016/j.ajodo.2006.03.034
- Cremonini F, Vianello M, Bianchi A, et al. The effect of thickness and deflection of orthodontic thermoplastic materials on its mechanical properties. Dent J. 2019;85(5):16–26.
- Proffit WR, Fields Jr HW, Sarver DM. Contemporary orthodontics. St. Louis: Elsevier; 2000.
- Hunter CA, Sanders JKM. The nature of .pi.-.pi. interactions. J Am Chem Soc. 1990;112(14):5525–5534. doi:10.1021/ja00170a016
- Olabis O. Polymer-Polymer Miscibility. Elsevier; 2012.
- Zin MH, Abdan K, Norizan MN. The effect of different fiber loading on flexural and thermal properties of banana/pineapple leaf (PALF)/glass hybrid composite. In: Structural health monitoring of biocomposites, fibre-reinforced composites and hybrid composites. Woodhead Publishing; 2019. p. 1–17.
- Elshazly TM, Nang D, Golkhani B, et al. Effect of thermomechanical aging of orthodontic aligners on force and torque generation: an in vitro study. J Mech Behav Biomed Mater. 2023;143:105911, doi:10.1016/j.jmbbm.2023.105911
- Hahn W, Fialka-Fricke J, Dathe H, et al. Initial forces generated by three types of thermoplastic appliances on an upper central incisor during tipping. Eur J Orthod. 2009;31(6):625–631. doi:10.1093/ejo/cjp047
- Elkholy F, Mikhaiel B, Schmidt F, et al. Mechanical load exerted by PET-G aligners during mesial and distal derotation of a mandibular canine. J Orofac Orthop. 2017;78:361–370. doi:10.1007/s00056-017-0090-4
- Elkholy F, Schmidt F, Jäger R, et al. Forces and moments applied during derotation of a maxillary central incisor with thinner aligners: an in-vitro study. Am J Orthod Dentofac Orthop. 2017;151(2):407–415. doi:10.1016/j.ajodo.2016.08.020
- Engelke B. (2010). Kraft-und Drehmomentabgabe thermoplastisch geformter Schienen bei Frontzahnderotation vor und nach Alterungssimulation. ediss.uni-goettingen.
- Kohda N, Iijima M, Muguruma T, et al. Effects of mechanical properties of thermoplastic materials on the initial force of thermoplastic appliances. Angle Orthod. 2013;83(3):476–483. doi:10.2319/052512-432.1
- Hertan E, McCray J, Bankhead B, et al. Force profile assessment of direct-printed aligners versus thermoformed aligners and the effects of non-engaged surface patterns. Prog Orthod. 2022;23(1):49, doi:10.1186/s40510-022-00443-2
- Grant J, Foley P, Bankhead B, et al. Forces and moments generated by 3D direct printed clear aligners of varying labial and lingual thicknesses during lingual movement of maxillary central incisor: an in vitro study. Prog Orthod. 2023;24(1):23, doi:10.1186/s40510-023-00475-2
- Elshazly TM, Nang D, Golkhani B, et al. Effect of aging of orthodontic aligners in different storage media on force and torque generation: an in vitro study. Oral. 2023;3(1):67–76. doi:10.3390/oral3010007
- Elshazly TM, Keilig L, Nang D, et al. Effect of thermomechanical aging on force system of orthodontic aligners made of different thermoformed materials. J Orofac Orthop der Kieferorthopädie. 2024: 1–9. doi:10.1007/s00056-024-00527-0