Abstract
Ho3+ ion-substituted nanocrystalline cobalt ferrite materials with the chemical formula CoFe2− x Ho x O4 for x = 0.0, 0.025, 0.05, 0.075 and 0.1 have been synthesised by standard citrate precursor method. Crystal structure and phase purity have been studied by powder X-ray diffraction (XRD) method by employing Rietveld refinement technique. The distribution of cations between the tetrahedral site (A-site) and octahedral site (B-site) has been estimated by Rietveld analysis. The refinement result shows that Ho3+ ion has a strong preference for octahedral sites (B-sites). The lattice constants decrease with the Ho3+ concentration up to x = 0.05. Crystallite size decreases with the Ho3+ concentration. The magnetic hysteresis loop measurements have been carried out at room temperature using a vibrating sample magnetometer (VSM) over a field range of ±2 T. The magnetisations in saturation have been analysed by employing the ‘law of approach (LA)’ technique. The magnetocrystalline anisotropy constant and saturation magnetisation are found to decrease with the Ho3+ concentration up to x = 0.05. The coercivity decreases with the Ho3+ concentration. The vibrational modes of the octahedral and tetrahedral metal complex in the sample have been carried out using Fourier transform infrared spectroscopy (FT-IR). The FT-IR spectra of the samples have been analysed in the wave number range of 350–1000 cm−1. We have observed two prominent absorption bands which are assigned to tetrahedral and octahedral metal complexes. The elemental analysis has been carried out using energy dispersive spectroscopy (EDS) with the help of field emission scanning electron microscope (FE-SEM) and the results reveal that, elements are as per the stoichiometric ratio in all the samples.
1. Introduction
Ferrimagnetic spinel ferrites having general molecular formula (M)2+[Fe2]3+O4: M = Co2+, Fe2+, Ni2+, etc.) are important classes of magnetic ceramics which crystallise to a cubic closed-pack structure of oxygen ions with space group. In spinel ferrite, the unit cell consists of eight formula units, i.e. 56 ions, in which 32 divalent oxygen ions are in direct contact to one another forming interstitial sites called tetrahedral (A-sites) and octahedral (B-sites) sites. The unit cell consists of 64 tetrahedral and 32 octahedral sites, out of which eight tetrahedral and 16 octahedral sites are occupied by divalent and trivalent metal cations, respectively Citation1. In general, simple single-magnetic cation spinel ferrites have limited technological applications but mixed spinel ferrites are widely used because they exhibit wide variety of physical properties. In mixed spinel ferrites, the magnetic properties depend on several factors such as, method of preparation, chemical composition, annealing temperature and distribution of cations among the tetrahedral (A) and octahedral (B) sites Citation1. However, understanding the cation distribution in these mixed spinel ferrites and their effect on the magnetic properties is still a challenging problem. Among the mixed spinel ferrites, cobalt ferrite (CoFe2O4) has drawn considerable attention due to its high coercivity, moderate saturation magnetisation along with good mechanical hardness and chemical stability Citation2. It is a hard ferrimagnetic material with a high-magnetic ordering temperature around ∼520°C and it is represented as (
)[
]O4, where cations inside the round and square brackets occupy A-sites and B-sites, respectively, and x depends on the thermal history and preparation conditions Citation3. This material is a versatile magnetic material for technological applications such as, magnetic resonance imaging contrast agents, data storage devices, transformer cores, ferrofluid technology, magneto caloric refrigeration, etc. Citation4–6.
The cubic CoFe2O4 has large magnetocrystalline anisotropy energy (MAE) with positive anisotropy constant and it has six easy directions along the cubic edges of the crystal represented as ⟨100⟩, four hard directions across the body diagonals denoted as ⟨111⟩ and 12 saddle points across the face diagonals which lead to large and positive magnetocrystalline anisotropy constant (K 1) Citation7–9. The large magnetocrystalline anisotropy of pure cobalt ferrite (CoFe2O4) is primarily due to a strong spin(S)–orbit (L) coupling in Co2+ ions at the octahedral sites. The crystal field (trigonal field) is not capable of removing the orbital degeneracy of Co2+ at the octahedral sites so that the orbital magnetic moment is not quenched and therefore there is strong L–S coupling which produces large MAE Citation10. Thus large multiaxial positive K 1 makes it hard magnetic material. The reported values of K 1 of bulk CoFe2O4 at room temperature range from 2.1 × 106 to 3.9 × 106 erg/cm3 depending on the stoichiometry of the material and heat treatment Citation11,Citation12. The K 1 of nanocrystalline cobalt ferrite are found to depend on synthesis method as well as annealing temperature Citation13. The K 1 of nanocrystalline cobalt ferrite synthesised by citrate precursor method and annealed at 400°C (crystallite size about ∼12 nm) is found to be 0.843 × 106 erg/cm3 and increase with the crystallite size Citation13. The K 1 of CoFe2O4 particles of size about ∼3.3 nm is found to be 3.15 × 107 erg/cm3 Citation14. This magnetocrystalline anisotropy is a very important parameter for the characterisation of the magnetic materials, which decides whether the magnetic material is magnetically soft or hard material and, it can be tuned by replacing A site and/or B site by different transition and non-transition elements. The recent investigations of the substitution at the Fe sites of CoFe2O4 by various cations like Ga, Mn, Al, Cr, etc., have revealed the change in atomic level magnetic interaction, which has modified the K 1 and magnetic properties Citation15–18.
The magnetic properties in mixed spinel cobalt ferrite are largely governed by various superexchange interactions (i.e. Fe3+(B)–O–Fe3+(B), Fe3+(B)–O–Co2+(B), Fe3+(B)–O–Fe3+(A), Co2+(B)–O–Fe3+(A) and Co2+(A)–O–Fe3+(B). Hence, the magnetic behaviour is largely governed by the spin coupling of the unpaired transition metal 3 d electrons present at the A- and B-sites Citation19. The RE3+ (RE ≈ Rare Earth) ions possess a variety of magnetic properties such as, their magnetic moment vary from 0 (La3+) to 10.64 µB (Dy3+) Citation20–23. If there is substitution of small amount of RE cations (RE3+, i.e. the 4f elements series) in place of Fe ions, one can expect an appearance of spin coupling of 3 d-4f electrons, which will modify the magnetic properties such as change of magnetic moment, K 1, etc. Several authors have reported the synthesis of RE3+ substituted nanocrystalline spinel ferrites using solid-state route. However, it suffers from lot of drawbacks like phase segregation such as orthoferrites (REFeO3) Citation24–28, hematite (α-Fe2O3) Citation29,Citation30 and metal monoxides Citation31 even for very low RE3+ concentration. However, there are few reports available, which have mentioned the synthesis of RE3+ substituted nanocrystalline spinel ferrites in single-phase form using different chemical routes despite having big difference in ionic radius of RE3+ and Fe3+ ions. For instance, using micellar route, Zhao et al. Citation23 have prepared the pure and chemically homogeneous CoFe2− x Nd x O4 up to x = 0.2. Also CoFe2− x RE x O4 with RE = Ce, Sm, Eu, Gd, DY and Er up to x = 0.12 have been prepared by Kahn et al. Citation20 using the same micellar route. Kim et al. Citation32 have successfully synthesised pure and homogeneous CoFe1.9RE0.1O4 nanocrystals with RE = Y, La, Nd and Gd. Panda et al. Citation21 have prepared CoFe2− x RE x O4 with RE = Gd and Pr in single phase without any chemical heterogeneities up to x = 0.2. Tahar et al. Citation33 have successfully synthesised CoFe1.9RE0.1O4 (RE = La, Ce, Nd, Sm, Eu, Gd, Tb and Ho) in single phase using polyol method. Here, we have used citrate precursor method to synthesise the Ho3+ substituted cobalt ferrite samples and observed that, the samples are in crystalline state and single-phase form even at low annealing temperature despite having large difference in ionic radius of Ho3+ and Fe3+.
The carriers of magnetism in RE elements are the 4f electrons. The holonium (Ho3+) has 4f electrons Citation10 and it has very high magnetic moment (10.60 µB for Ho3+). Hence, due to its magnetic nature, substituting Ho3+ ion in the place of Fe3+ in cobalt ferrite, it gives rise to 3 d–4f electron coupling. Therefore, one can expect to modify the structural and magnetic properties. The structural and magnetic properties of Ho3+ substituted nanocrystalline cobalt ferrite have been reported by various groups. Muthuselvam et al. Citation34 have synthesised CoFe1.95Ho0.05O4 spinel ferrite nanomaterial using mechanical alloying method and, results revealed that samples show soft ferromagnetic character. Tahar et al. Citation33 have prepared CoFe1.9Ho0.1O4 nanoparticles by polyol method and found that particles are superparamagnetic at room temperature. Even though there are a few reports on Ho3+ doped CoFe2O4, according to our knowledge there are no detailed studies on crystal structure of nanocrystalline CoFe2− x Ho x O4. Also, there are inadequate information on magnetic properties and magnetocrystalline anisotropy of Ho3+ doped cobalt ferrite. Hence in this report, we have studied detailed crystal structure and size-dependent K 1 at room temperature of CoFe2− x Ho x O4 (x = 0.0, 0.025, 0.05, 0.075 and 0.1) nanocrystalline materials.
2. Experimental
Nanocrystalline powders of CoFe2− x Ho x O4 for x = 0.0, 0.025, 0.05, 0.075 and 0.1 were prepared by the citrate precursor method. Cobalt nitrate (Co(NO3)2 · 6H2O), iron nitrate (Fe(NO3)3 · 9H2O), holonium oxide (Ho2O3) and Citric acid (C6H8O7 · H2O) with 99.9% purity were used as starting materials. The molar ratio of metal nitrates to citric acid was taken as 1 : 3. The metal nitrates were dissolved in a deionised water (milli Q grade) to get a solution. An aqueous solution of citric acid was mixed with metal nitrates solution. The mixed solution was heated at 80°C with constant stirring using hot plate. The solution became viscous and finally forming brown gel. The gel was dried overnight using an oven at 80°C in order to remove excess water. During the process of drying, the gel swells into the fluffy mass and eventually broke into brittle flakes. The resulting materials were heat treated in air atmosphere at 200°C and 600°C for 2 h at each temperature. The crystalline phase of the annealed samples was identified by the powder XRD method (Rigaku Miniflex) using Cu-Kα radiation. The magnetic hysteresis loops were recorded at room temperature (300 K = 27°C) by using a LakeShore (Model No. 7410) VSM. The maximum field used was ±2 T. FT-IR was recorded with a Perkin Elmer (Model Spectrum 400) within the wave number range of 325–1000 cm−1. The compositions of all the samples were studied by EDS using Hitachi S4800 FE-SEM.
3. Results and discussions
3.1. Structural analysis
Typical XRD pattern of the sample CoFe1.9Ho0.1O4 annealed at 200°C is shown in . The XRD pattern shows broader peaks and incomplete crystallisation. It reveals that, due to large difference in ionic radius of Ho3+ (0.90 Å) and Fe3+ (0.64 Å) cations, thermal energy for 200°C annealed samples is not sufficient for the complete incorporation of the Ho3+ ions into the spinel lattice. Therefore, more energy is needed to make Ho3+ ions enter into the spinel lattice to improve the crystallisation. So, we have annealed the samples at 600°C for 2 h and the XRD patterns are shown in . The XRD patterns show an improvement in the sharpness of the peaks and reduction in the peak broadening compared to 200°C annealed samples. It reveals that the growth of crystal take place with increasing heat treatment. All the samples are essentially in single-phase form. We have not observed any trace of impurity peaks which confirms that Ho3+ ions have been incorporated into the spinel lattice. All the XRD peaks could be indexed to space group with cubic symmetry. We have observed that, the XRD peaks for the samples annealed at 600°C appear to be broadened with increasing Ho3+ concentration (x = 0.1), as a result of incorporation of the Ho3+ ion into the spinel lattice. With the gradual increase in Ho3+ concentration for x>0.1, crystallisation of the samples become more difficult and some sort of amorphous-like phases (figure not shown) prevail because more dopant concentration leads to a higher potential barrier that Ho3+ ion has to overcome for entering the spinel crystal lattice. The segregation of different phases may have started x< 0.1 due to which we have observed anomaly in physical properties (magnetic, lattice parameters, etc.) with Ho3+ concentration for x > 0.05 (discussed later). However, we have not observed any phase segregation by our present XRD study. It requires more detailed study by other techniques to resolve the issue which is beyond the scope of this article.
Figure 2. XRD patterns of the samples CoFe2− x Ho x O4 (x = 0.0, 0.025, 0.05, 0.075 and 0.1) annealed at 600°C.
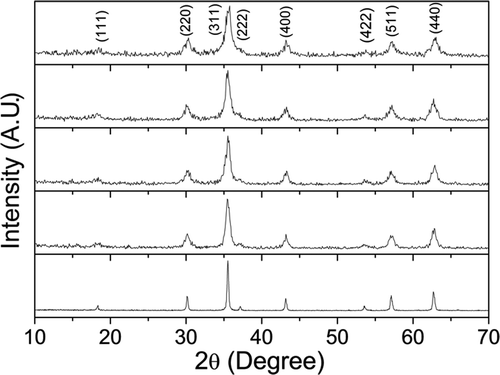
We tried to increase the crystallinity of high Ho3+ doped samples (x ≤ 0.1) by annealing above 600°C (i.e. 1000°C). However, we have observed that in addition to spinel phase, there is also another phase which has segregated as HoFeO3 (figure not shown). So we conclude that, the RE3+ ions (Ho3+ ion) which have a higher ionic radius than Fe3+ ion can be substituted for Fe3+, when the samples are in nanocrystalline form. In the nanocrystalline form, due to large surface to volume atoms reduces the symmetry of the crystals as well as crystal anisotropy, whereas in the bulk materials, crystals are highly anisotropic and have a high degree of symmetry which leads to phase segregation. Hence, in this report we have studied in detail for 600°C annealed nanocrystalline sample.
All XRD patterns were analysed employing Rietveld technique Citation35 with the help of Fullprof Suite 2009 release programme. The patterns for all the samples could be refined using the space group. Typical XRD patterns along with Rietveld refinement are shown in for the sample CoFe1.95Ho0.05O4 annealed at 600°C. Here the experimental data are shown as open circles and calculated intensities are shown as solid line. The bottom line represents the difference between measured and calculated intensities. The allowed Braggs positions for the
space group are marked as vertical lines. All the observed peaks are allowed at Bragg 2θ positions. The typical fractional atomic positions and isothermal parameters of the atoms for the sample CoFe1.95Ho0.05O4 annealed at 600°C are given in . The oxygen positions (x = y = z) were taken as free parameters, however all other atomic fractional positions were taken as fixed. Other parameters, such as, lattice constants, isothermal parameters, occupancies, scale factors and shape parameters were taken as free parameters. Background was refined by using pseudo voigt function.
Figure 3. Rietveld refined XRD pattern for the sample CoFe1.95Ho0.05O4 annealed at 600°C. The circles represent experimental points and the solid line represents Rietveld refined data. The bottom line shows the difference between the experimental and refined data. The marked 2θ positions are the allowed Bragg peaks.
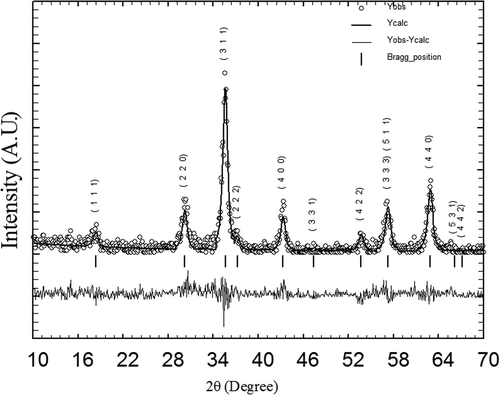
Table 1. Atomic coordinates and isothermal parameters for CoFe1.95Ho0.05O4 composition.
The R p (profile factor) and refined lattice parameters for all the samples annealed at 600°C are listed in . The R p factors are found to be large. A similar R p factor has been observed by the other research group for nanocrystalline samples Citation36. It could be due to the low signal-to-noise ratio of XRD patterns for nanocrystalline materials. However, we have observed a low value of χ 2 (goodness of fit) which justifies the goodness of refinement. In the diffraction pattern of nanocrystalline materials, diffuse scattering is dominant than in those of bulk crystalline materials due to large ratio of surface to volume atoms. The diffuse scattering becomes significant at the nanoscale while Bragg scattering gets diminished which leads to decline in crystallinity Citation37. Hence large reliability factors are observed.
Table 2. Parameters obtained from Rietveld analysis of XRD patterns for the sample CoFe2− x Ho x O4 (x = 0.0, 0.025, 0.05, 0.075 and 0.1) annealed at 600°C. Errors of the lattice parameters are shown in brackets.
The analysis of the crystallite size has been carried out using the broadening of the XRD peaks. Peak broadening comes from several sources such as instrumental effect, finite crystallite size and strain effect within the crystal lattice. The crystallite size of the samples have been obtained using Rietveld method because in Rietveld method all the instrumental factors are taken into account for the correction of peak broadening. A complete expression used in Rietveld method Citation35 is defined as,
The cation distributions among the tetrahedral and octahedral interstitial sites were estimated by the Rietveld refinement of the occupancy values. The estimated cation distributions from Rietveld analysis for the samples annealed at 600°C are listed in . One can observe that Ho3+ ions are within the error in the A-site. Hence, Ho3+ ions show a strong preference for octahedral sites. The valency of Ho atom is +3 only. The crystal field stabilisation energy of Ho3+ is high and element having high crystal field stabilisation energy have a strong affinity to the B-sites. Hence, the strong preference of Ho3+ ion for the B-sites may be due to its high crystal field stabilisation energy Citation38. In spinel lattice, the radius of the octahedral site is larger than the tetrahedral site. The ionic radius of the Ho3+ ion is larger than Fe ions. One can assume that when small amount of Ho3+ cation is substituted for Fe3+ cation, it enter into the octahedral sites and induces rearrangement of cations between the tetrahedral and octahedral sites to minimise the free energy of the system. By increasing the Ho3+ concentration, it induces partial migration of Co2+ ions (0.74 Å) from B to A sites accompanied by an opposite transfer of an equivalent number of Fe3+ ions (0.64 Å) from A to B sites in order to relax the strain at the B-sites. The migration of cations from tetrahedral sites to octahedral sites and vice-versa could produce a significant effect on the lattice constant.
Table 3. Site occupancies of cations for the samples CoFe2− x Ho x O4 (x = 0.0, 0.025, 0.05, 0.075 and 0.1) annealed at 600°C.
The values of lattice constants for all the samples annealed at 600°C are listed in . We have observed that the lattice constant decreases with the Ho3+ concentration up to x = 0.05. Similar decrease in lattice parameters have been observed for RE3+ ions substituted cobalt ferrite nanocrystals Citation39,Citation40. The observed decrease of lattice constant could be explained on the basis of rearrangement of cations between the A-site and B-site in order to relax the lattice strain (discussed in the above section). For x > 0.05, there is inconsistency in the variation of lattice constant. It appears that, there might exist some other phase (secondary phase) in a very small amount in addition to Co–Ho ferrite. This small amount of extra phase might induce some distortion in the spinel lattice corresponding to some expansion of the spinel lattice with the result of increasing lattice parameter. But the XRD pattern could not show the evidence of extra phase. This might be due to small Ho3+ doping concentration in the sample, the extra phase is below the detection limit of X-ray spectrum. It requires a more detailed study. In this regard, we have planned for high power X-ray radiation and Mösbauer spectroscopy study to understand these samples.
3.2. Spectral measurement
The FT-IR spectra for the sample CoFe2− x Ho x O4 annealed at 600°C with x = 0.0 and 0.025 are shown in . The spectra indicate the presence of two strong absorption bands in the range of 350–600 cm−1 which is a common feature of the spinel ferrite Citation41. For undoped sample (x = 0.0), the spectra show two prominent absorption bands with peaks at 502 (ν 1) and 435 (ν 2) cm−1. The band positions are found to be in agreement with the characteristic infrared absorption bands of cobalt ferrite nanocrystals Citation42. The band ν 1 is assigned to the vibration of the bond between the oxygen ion and the tetrahedral metal ion (O–MTet) and the band ν 2 is assigned to the vibration of the bond between the oxygen ion and the octahedral metal ion (O–MOct) Citation43. When Ho3+ ion is substituted for Fe ion (for x = 0.025), we observed that the absorption bands (ν 1) and (ν 2) slightly shift with peaks at 494 and 412 cm−1, which indicates that Ho3+ ion has been incorporated into the spinel lattice which supports the XRD analysis.
3.3. Elemental analysis
Typical FE-SEM image of the sample CoFe1.975Ho0.025O4 annealed at 600°C is shown in . The image shows that the particles have an almost homogeneous distribution. The composition of the samples CoFe2− x Ho x O4 is determined using the EDS analysis. The compositional analysis for all the samples was found to be close to stoichiometry. The typical EDS pattern of sample CoFe1.975Ho0.025O4 annealed at 600°C is shown in . The EDS pattern reveals the presence of Co, Fe, Ho, Au and O elements in the sample. One cansee that except the extra Au peak; no any other peaks have been traced. The gold peak appears due to the thin coating on the sample surface to make it conducting. It reveals that there is no contamination in the sample.
3.4. Magnetic studies
Magnetic hysteresis loops for CoFe2− x Ho x O4 samples annealed at 600°C are shown in . The saturation magnetisations M S for the samples CoFe2− x Ho x O4 were estimated from a fit over the high field data using the ‘LA to Saturation’ equation (discussed in the next section). The values of saturation magnetisation for all the samples are listed in . We have observed that saturation magnetisation decreases with the Ho3+ concentration up to x = 0.05 composition. The observed behaviour could be explained as follows: (1) the surface effect becomes prominent in nanocrystalline material due to large ratio of surface to volume atoms. At the surface, structure is distorted and atoms are under the effect of strain which leads to vacancies, variety of interatomic spacing and low coordination number. All of these factors induce a broken exchange bonds for the surface atoms, which leads to spin disorder Citation44. The disordered spins at the surface may exhibit low magnetisation. (2) The strength of superexchange interaction are expected to be modified in RE3+(rare earth) ion doped cobalt ferrite due to the appearance RE3+–Fe3+/Co2+ interaction which is a weak interaction relative to Fe3+–Fe3+/Co2+ interaction Citation45, hence affects the saturation magnetisation. For x > 0.05, there is unusual behaviour of saturation magnetisation with Ho3+ concentration.
Figure 7. Hysteresis loops for the samples CoFe2− x Ho x O4 (x = 0.0, 0.025, 0.05, 0.075 and 0.1) annealed at 600°C.
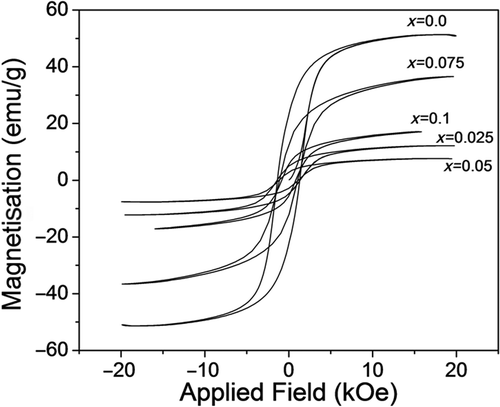
Table 4. Coercivity, saturation magnetisation (M S), and anisotropic constant (K 1) of the samples CoFe2− x Ho x O4 (x = 0, 0.025, 0.05, 0.075 and 0.1) annealed at 600°C.
The values of coercivity for all the samples are listed in . We have observed that the coercivity decreases with the Ho3+ concentration. In a single domain region the variation of coercivity with the crystallite size is expressed as Citation19,
In order to extract the anisotropy information of the samples CoFe2− x Ho x O4 annealed at 600°C, the ‘LA’ to saturation was employed to analyse the data in saturation region. LA describes the dependence of magnetisation M on the applied magnetic field for H ≫ H c. The magnetisation near the saturation M S can be written as Citation10,
The values of K 1 for all the samples are listed in . Our result of magnetocrystalline anisotropy for undoped sample (CoFe2O4) is comparable to the earlier reports Citation46. We have observed that the K 1 decreases with the Ho3+ concentration up to x = 0.05 composition. The origin of the magnetocrystalline anisotropy in the spinel ferrite is the L–S coupling at the crystal lattices and in nanocrystalline material it strongly depends on the crystallinity Citation47. Increasing Ho3+ ion in the place of Fe ion leads to decrease of crystallite size and enhancement of surface area in the sample. At the surface of the samples, surface strain is dominant, i.e. crystal lattice are not in well-defined order which may perturb the crystal symmetry at the surface and affects the L–S coupling, i.e. magnetocrystalline anisotropy. It reveals that for nanocrystalline material, surface effects play a dominant role rather than cation distribution for the determination of magnetic anisotropy, which we have planned to carry out by high-power XRD (Synchrotron radiation and Mossbauer spectroscopy). Coercivity, M S and K 1 are not consistent with the increase of Ho3+ concentration for x > 0.05. It could be due to phase segregation which has been discussed in the previous section (XRD analysis). Hence, the materials for x > 0.05 are under investigation.
4. Conclusions
Nanocrystalline Ho3+ substituted cobalt ferrite CoFe2− x Ho x O4 (x = 0.0, 0.025 and 0.05) have been synthesised successfully by citrate precursor method. The lattice constant strongly depends on the Ho3+ concentration. FT-IR spectra show two significant absorption bands which confirm the incorporation of the Ho3+ ion into the spinel lattice. The magnetic properties are strongly affected by surface effect rather than cation distribution. The samples with a low concentration of Ho3+ reveal that there is tendency of the material to show soft ferrimagnetic character. For higher concentration of Ho3+ (x > 0.05), it is difficult to control the physical parameters.
Acknowledgement
The authors are thankful to the Centre of Instrumentation facility (CIF), Indian Institute of Technology Guwahati, Guwahati – 781039, for extending the VSM facilities. The authors gratefully acknowledge Department of Atomic Energy, Government of India (Sanction No. 2011/20/37 P/03/BRNS/0076) for the financial support.
References
- Goldman , A . 2006 . Modern Ferrite Technology , NY : Springer .
- Valenzuela , R . 1994 . Magnetic Ceramics , Cambridge : Cambridge University Press .
- Haneda , K and Morrish , AH . 1988 . Non collinear magnetic structure of CoFe2O4 . J. Appl. Phys. , 63 : 4258 – 4263 . doi: 10.1063/1.340197
- Snelling , EC . 1988 . Soft Ferrites: Properties and Applications , London , UK : Butterworths .
- Dormann , JL and Fiorani , D . 1992 . Magnetic Properties of Fine Particles , Amsterdam : North-Holland .
- Hafeli , U , Schuut , W , Teller , J and Zborowski , M . 1997 . Scientific and Clinical Applications of Magnetic Carriers , New York : Plenum Press .
- Smit , J . 1959 . Ferrites , New York : Wiley .
- Hyeon , T . 2003 . Chemical synthesis of magnetic nanoparticle . Chem. Commun. , 8 : 927 – 932 . doi: 10.1039/b207789b
- Walker , M , Mayo , PI , O’Grady , K , Charles , SW and Chantrell , RW . 1993 . The magnetic properties of single-domain particles with cubic anisotropy. I. Hysteresis loops . J. Phys. Condens. Matter, , 5 : 2779 – 2783 . doi: 10.1088/0953-8984/5/17/012
- Chikazumi , S . 1997 . Physics of Ferromagnetism , NY : Oxford University Press .
- Bozorth , RM , Tilden , EF and Williams , AJ . 1955 . Anisotropy and magnetostriction of some ferrites . Phys. Rev. , 99 : 1788 – 1798 . doi: 10.1103/PhysRev.99.1788
- Shenker , H . 1957 . Magnetic anisotropy of cobalt ferrite and nickel cobalt ferrite . Phys. Rev. , 107 : 1246 – 1248 . doi: 10.1103/PhysRev.107.1246
- Kumar , L and Kar , M . 2011 . Effect of annealing temperature and preparation condition on magnetic anisotropy in nanocrystalline cobalt ferrite . IEEE Trans. Magn. , 47 : 3645 – 3648 . doi: 10.1109/TMAG.2011.2151841
- Tung , LD , Kolesnichenko , V , Caruntu , D , Chou , NH , O’Connor , CJ and Spinu , L . 2003 . Magnetic properties of ultrafine cobalt ferrite particles . J. Appl. Phys. , 93 : 7486 – 7489 . doi: 10.1063/1.1540145
- Zhou , B , Zheng , Y , Liao , C , Cheng , F , Yan , C , Yao , L and Wang , SY . 2001 . Enhanced magnetooptical kerr effects and decreased curie temperature in Co–Mn ferrite thin films . Appl. Phys. Lett. , 79 : 1849 – 1852 . doi: 10.1063/1.1402656
- Melikhov , Y , Snyder , JE , Jiles , DC , Ring , AP , Paulsen , JA , Lo , CCH and Dennis , KW . 2006 . Temperature dependence of magnetic anisotropy in Mn-substituted cobalt ferrite . J. Appl. Phys. , 99 : 08R102 – 08R105 . doi: 10.1063/1.2151793
- Ranvah , N , Melikhov , Y , Jiles , DC , Snyder , JE , Moses , AJ , Williams , PI and Song , SH . 2008 . Temperature dependence of magnetic anisotropy of Ga-substituted cobalt ferrite . J. Appl. Phys. , 103 : 07E506 – 07E509 . doi: 10.1063/1.2832503
- Kumar , L and Kar , M . 2011 . Influence of Al3+ ion concentration on the crystal structure and magnetic anisotropy of nanocrystalline spinel cobalt ferrite . J. Magn. Magn. Mater. , 323 : 2042 – 2048 . doi: 10.1016/j.jmmm.2011.03.010
- Culity , BD and Graham , CD . 2009 . Introduction to Magnetic Materials , Hoboken, NJ : John Wiley & Sons, Inc. .
- Kahn , ML and Zhang , ZJ . 2001 . Synthesis and magnetic properties of CoFe2O4 spinel ferrite nanoparticles doped with lanthanide ions . Appl. Phys. Lett. , 78 : 3651 – 3654 . doi: 10.1063/1.1377621
- Panda , RN , Shih , JC and Chin , TS . 2003 . Magnetic properties of nano-crystalline Gd-or Pr-substituted CoFe2O4 synthesized by the citrate precursor technique . J. Magn. Magn. Mater. , 257 : 79 – 86 . doi: 10.1016/S0304-8853(02)01036-3
- Tahar , LB , Smiri , LS , Artus , M , Joudrier , AL , Herbst , F , Vaulay , MJ , Ammar , S and Fievet , F . 2007 . Characterization and magnetic properties of Sm- and Gd-substituted CoFe2O4 nanoparticles prepared by forced hydrolysis in polyol . Mater. Res. Bull. , 42 : 1888 – 1896 . doi: 10.1016/j.materresbull.2006.12.014
- Zhao , L , Cui , Y , Yang , H , Yu , L , Jin , W and Feng , S . 2006 . The magnetic properties ofNi0.7Mn0.3GdxFe2 − xO4 ferrite . Mater. Lett. , 60 : 104 – 108 . doi: 10.1016/j.matlet.2005.07.083
- Bharathi , KK , Balamurugan , K , Santosh , PN , Pattabiraman , M and Markandeyulu , G . 2008 . Magnetocapacitance in Dy-doped Ni–ferrite . Phys. Rev. B. , 77 : 172401 – 172404 . doi: 10.1103/PhysRevB.77.172401
- Bharathi , KK and Markandeyulu , G . 2008 . Ferroelectric and ferromagnetic properties of Gd substituted Ni–ferrite . J. Appl. Phys. , 103 : 07E309 – 07E311 .
- Bharathi , KK , Chelvane , JA and Markandeyulu , G . 2009 . Magnetoelectric properties of Gd and Nd-doped nickel ferrite . J. Magn. Magn. Mater. , 321 : 3677 – 3680 . doi: 10.1016/j.jmmm.2009.07.011
- Haj , MA . 2006 . Structural characterization and magnetization of Mg0.7Zn0.3SmxFe2−xO4 ferrites . J. Magn. Magn. Mater. , 299 : 435 – 439 . doi: 10.1016/j.jmmm.2005.05.003
- Rezlescu , N , Rezlescu , E , Pasnicu , C and Craus , ML . 1994 . Effects of the rare-earth ions on some properties of a nickel–zinc ferrite . J. Phys. Condens. Matter , 6 : 5707 – 5712 . doi: 10.1088/0953-8984/6/29/013
- Sileo , EE , Rotelo , R and Jacobo , SE . 2002 . Nickel zinc ferrites prepared by the citrate precursor method . Physica B , 320 : 257 – 260 . doi: 10.1016/S0921-4526(02)00705-6
- Jacobo , SE , Fano , WG and Razzitte , AC . 2002 . The effect of rare earth substitution on the magnetic properties of Ni0.5Zn0.5MxFe2−xO4 (M: rare earth) . Physica B , 320 : 261 – 263 . doi: 10.1016/S0921-4526(02)00706-8
- Sileo , EE and Jacobo , SE . 2004 . Gadolinium–nickel ferrites prepared from metal citrates precursors . Physica B , 354 : 241 – 245 . doi: 10.1016/j.physb.2004.10.002
- Kim , WC , Kim , SJ , Sur , JC and Kim , CS . 2002 . Structural and magnetic properties of CoFe1.9RE0.1O4 (RE = Y, La) prepared by a sol–gel method . J. Magn. Magn. Mater. , 242 : 197 – 200 . doi: 10.1016/S0304-8853(01)01168-4
- Tahar , LB , Artus , M , Ammar , S , Smiri , LS , Herbst , F , Vaulay , MJ , Richard , V , Greneche , JM , Villian , F and Fievet , F . 2008 . Magnetic properties of CoFe1.9RE0.1O4 nanoparticles (RE = La, Ce, Nd, Sm, Eu, Gd, Tb, Ho) prepared in polyol . J. Magn. Magn. Mater. , 320 : 3242 – 3250 . doi: 10.1016/j.jmmm.2008.06.031
- Muthuselvam , IP and Bhowmik , RN . 2010 . Mechanical alloyed Ho3+ doping in CoFe2O4 spinel ferrite and understanding of magnetic monodomain . J. Magn. Magn. Mater. , 322 : 767 – 776 . doi: 10.1016/j.jmmm.2009.10.057
- Young , RY . 1996 . The Rietveld Method , Oxford : Oxford University Press .
- Bhagwat , M , Ramaswamy , AV , Tyagi , AK and Ramaswamy , V . 2003 . Rietveld refinement study of nanocrystalline copper doped zirconia . Mater. Res. Bull. , 38 : 1713 – 1724 . doi: 10.1016/S0025-5408(03)00201-0
- Jovic , NG , Masadeh , AS , Kremenovic , AS , Antic , BV , Blanusa , JL , Cvjeticanin , ND , Goya , GF , Antisari , MV and Bozin , ES . 2009 . Effect of thermal annealing on structural and magnetic properties of lithium ferrite nanoparticles . J. Phys. Chem. C , 113 : 20559 – 20567 . doi: 10.1021/jp907559y
- Burdett , JK , Price , GL and Price , SL . 1982 . Role of the crystal field theory in determining the structure of spinels . J. Am. Chem. Soc. , 104 : 92 – 95 . doi: 10.1021/ja00365a019
- Peng , J , Hojamberdiev , M , Xu , Y , Cao , B , Wang , J and Wu , H . 2011 . Hydrothermal synthesis and magnetic properties of gadolinium-doped CoFe2O4 nanoparticles . J. Magn. Magn. Mater. , 323 : 133 – 138 . doi: 10.1016/j.jmmm.2010.08.048
- Meng , X , Li , H , Chen , J , Mei , L , Wang , K and Li , X . 2009 . Mössbauer study of cobalt ferrite nanocrystals substituted with rare-earth Y3+ ions . J. Magn. Magn. Mater. , 32 : 1155 – 1158 . doi: 10.1016/j.jmmm.2008.10.041
- Waldron , RD . 1955 . Infrared spectra of ferrites . Phys. Rev. , 99 : 1727 – 1728 . doi: 10.1103/PhysRev.99.1727
- Zhao , L , Zhang , H , Xing , Y , Song , S , Yu , S , Shi , W , Guo , X , Yang , J , Lei , Y and Cao , F . 2008 . Studies on the magnetism of cobalt ferrite nanocrystals synthesized by hrdrothermal method . J. Solid State Chem. , 181 : 245 – 252 . doi: 10.1016/j.jssc.2007.10.034
- Ramankutty , CG and Sugunam , S . 2001 . Surface properties and catalytic activity of ferrospinels of nickel, cobalt and copper prepared by soft chemical method . Appl. Catal. A , 218 : 39 – 51 . doi: 10.1016/S0926-860X(01)00610-X
- Kodama , RH , Berkowitz , AE , McNiff , EJ and Foner , S . 1996 . Surface spin disorder in NiFe2O4 nanoparticles . Phys. Rev. Lett. , 77 : 394 – 397 . doi: 10.1103/PhysRevLett.77.394
- Zhao , L , Yang , H , Yu , L , Cu , Y , Zhao , X and Feng , S . 2006 . Fabrication and magnetic properties of nanocrystalline La, Nd, Gd substituted Ni–Mn ferrite . Jpn. J. Appl. Phys. , 45, : 4030 – 4034 . doi: 10.1143/JJAP.45.4030
- Franco , A Jr and silva , FCE . 2010 . High temperature magnetic properties of cobalt ferrite nanoparticles . Appl. Phys. Lett. , 96 : 172505 – 172508 . doi: 10.1063/1.3422478
- Chiu , WS , Radiman , S , Abd-Shukor , R , Abdullah , MH and Khiew , PS . 2008 . Tunable coercivity of CoFe2O4 nanoparticles via thermal annealing treatment . J. Alloys Compd. , 459 : 291 – 297 . doi: 10.1016/j.jallcom.2007.04.215