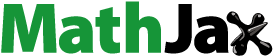
Abstract
In this work, a new ternary Ag/AgI/Ag3BiO3 photocatalyst was fabricated by an in-situ precipitation method, where Ag0 nanoparticles, serving as a mediator, implanted on the surface of glossy AgI, quantum efficiency being improved attributed to the surface plasmon resonance (SPR) effect. The composites showed superior photocatalytic performance to methylene blue (MB) degradation under visible illumination (λ > 420 nm). The Kobs values of Ag/AgI/Ag3BiO3-3 were 7.4 and 3.6 times compared with that of pristine Ag3BiO3 and AgI, respectively. Based on the series of experimental results, a Z-scheme heterojunction has formed in preparations. The electron-hole pairs maintained a transferable cycle, which efficiently inhibited the recombination of electron-hole pairs. According to active species analysis, holes were determined the predominant work-function in photocatalytic degradation system. The feasible mechanism has proposed to illustrate the photocatalytic decomposition of MB on Ag/AgI/Ag3BiO3. Our work has important implication for the management and practical application of catalysts.
1. Introduction
For decades, photocatalyst has always being exploited as a promising tool to alleviate increasing environment crisis. Including TiO2, ZnO, CdS, Fe2O3, Bi2O3 and AgX (X = Cl, Br, I) were studied by researchers as semiconductor photocatalyst [Citation1–6]. AgX that is a photosensitive material has always caught many eyes in photocatalytic field [Citation7–9]. The defect electronic structure of semiconductor results in highly recombination of photo-stimulated carriers, limiting the practical applications, but adulteration is greatly beneficial to the transmission of carriers, which may lead to a heterojunction formation [Citation10–12]. Using polyvinylpyrrolidone (PVP) as a surfactant, Zhang and colleagues [Citation13] controlled AgI/g-C3N4 heterojunction photocatalysts by a deposition-precipitation method, and the photocatalysts exhibited higher oxidation than initial AgI and g-C3N4. Parallelly, due to the powerful separation and migration of interfacial charge carries, AgX (X = I, Br)/CuBi2O4 heterojunction composites exhibited excellent photocatalytic performance [Citation14]. Fang et al. [Citation15] developed a WO3/Ag/AgCl film on glass by an impregnating–precipitation–photoreduction method, and Z-scheme was proposed to explain its excellent photocatalytic activity. A group [Citation16] constructed AgI nanoparticle-functionalized reduced graphene oxide aerogels, which not only exhibited better photocatalytic activity and stability, but were active catalysts for the synthesis of bis(indolyl)methane under solvent-free conditions. Studies on AgX are sufficient to demonstrate the importance of it in the field of photocatalysis.
Recently, Bi-based photocatalyst is a rising star at photocatalytic materials, and it has attracted extensive attention. For instance, (BiO)2CO3 [Citation17], Bi2MoO6 [Citation18] and BiVO4 [Citation19] etc. were found extensive applications in photocatalytic oxidations and reductions. Li et al. [Citation20] designed double-shell Bi2O3/Bi2WO6 hollow microspheres to rhodamine B degradation under visible-light irradiation. Li et al. [Citation21] prepared Z-scheme ternary g-C3N4/Bi2O3/BiPO4 heterostructure which extremely restrained the recombination of photo-generate electron and hole pairs, thus exhibiting outstanding oxidation and reduction abilities for methyl orange. As we all know, ABO3 is a perovskite oxide structure compound, and it can adopt majority of metallic ions. Gong and coworkers [Citation22] synthesized the AgBiO3 NPs and found that it could be rejected for mineralization including phenols and rhodamine B owe to AgBiO3 NPs self-produced amount of the main reactive oxygen species (ROS). M. Rurtz and M. Jansen [Citation23] fabricated Ag3BiO3 inorganic compound for the first time and proposed its structural model, considering Ag3BiO3 comprises of Ag2O and Bi2O3. To our best knowledges, there are hardly any reports about the optical properties of Ag3BiO3 up to now.
It is well-known that noble metal loading can improve quantum efficiency resulting from the observably surface plasmon resonance (SPR) effect under visible-light illumination [Citation24,Citation25]. Sellappan et al. [Citation26] designed, fabricated, and examined Ag/TiO2 and Au/TiO2 nanocomposites, and studied that interfacial charge transfer is the crucial cause of the activity enhancement where particles are in direct to contact with TiO2. Besides, since the SPR effect of noble mental loading, the absorption edge of composites can be enlarged in visible-light region [Citation27,Citation28].
Herein, we prepared a new ternary Ag/AgI/Ag3BiO3 photocatalyst via an in-situ precipitation method and investigated the activity of ternary composites on photocatalytic degradation of MB. The new composites exhibited higher quantum efficiency and outstanding photocatalytic performance under visible-light illumination. The stability of the composites was also investigated. Finally, the feasible mechanism was determined through various technical characterization and analysis.
2. Experimental
2.1. Materials
Bi(NO3)3·5H2O, NaOH, NH3·H2O, KI, concentrated nitric acid and absolute ethyl alcohol were purchased from Beijing Chemistry Company, China. AgNO3 was purchased from Shanghai chemical reagent Co. Ltd. Melamine was obtained from Sinopharm chemical reagent Co. Ltd. The deionized water (resistivity > 18.0 MΩ cm) was used throughout the experiments. All the reagents were bought from commercial sources of AR range and all of this without further purification.
2.2. Preparation of Ag3BiO3, AgI and Ag/AgI/Ag3BiO3 samples
The Ag3BiO3 nanoparticles were prepared as follows. Firstly, 3 mmol of AgNO3 was dissolved in 30 mL of deionized water forming colorless solution. Secondly, 1 mmol of Bi(NO3)3·5H2O reagent was dissolved in 10 mL of deionized water containing 2 mL of concentrated nitric acid. Then, the two solutions were mixed, and then 10 mL of 5 M NaOH solution was added slowly drop by drop in the mixing solution at the same time. Next, the suspensions were transferred into a Teflon-lined stainless steel autoclave (filled 70percent) of 100 mL capacity and heated at 453 K for 12 h, cooled to room temperature naturally and collected all depositions. Finally, Ag3BiO3 powder was obtained after several washings with distilled water and absolute ethanol and drying at 333 K for 12 h.
Ag/AgI/Ag3BiO3 was prepared by an in-situ precipitation method. Initially, in order to form silver ammonia solution, a set amount of AgNO3 solution was introduced moderate ammonia. Secondly, as-obtained Ag3BiO3 were added into abovementioned silver ammonia solution with magnetic stirring. Then, ratable AgI solution was instilled the abovementioned suspension and kept stirring for another 0.5 h. Finally, all sediments (Ag/AgI/ABO-X) were desiccated at 333 K for 12 h. These Ag/AgI/ABO-X (X = 1, 2, 3, 4, 5, the molar ratios of AgI to Ag3BiO3, Ag is trace) were collected for further use. For comparation, pure AgI was prepared under similar experimental conditions.
2.3. Apparatus
The crystalline structure of as-obtained composites was characterized by an X-ray diffractometer (2θ ranged from 10° to 80°). The morphological features of composites were characterized by a scanning electron microscope (SEM). An X-ray photoelectron spectroscopy (XPS) was also employed to detect the elements of compositions and its chemical state on a KRATOA XSAM800 with Mg Kα irradiation. UV–vis diffuse reflectance spectra (UV-DRS) was carried out on a UV2401 spectrophotometer to analyze the light absorption performance of samples. The photoluminescence (PL) spectra result of samples were performed with the excitation wavelength of 225 nm by a Varian Cary Eclipse spectrometer. The electrochemical impedance spectra (EIS) of all of samples were carried out on a Zahner electrochemical workstation. 300 W Xe arc lamp was occupied in the simulated solar-light.
2.4. Photocatalytic experiments
In typically, the photocatalytic activities of as-obtained samples were evaluated through removing MB dye under visible-light illumination. One 300 W Xe arc lamp acts as the illuminant to offer energies. The illuminated area is about 33.18 cm2, and the distance between the liquid level and light source is around 10 cm. In this work, 0.05 g of as-prepared samples and 100 mL MB solution (pristine absorbance is 2.50) were added into a glass reactor which kept room temperature inside. The suspensions were continuously stirred for 0.5 h absenting illumination before embarking on photocatalytic indeed works to get the adsorption or desorption equilibrium of MB dye on catalyst’s surface. Then, 3 mL of suspension was collected from reaction system at the given time interval. All collections were separated together via centrifugation at 5,000 rpm for 10 min. After that, UV-vis spectrophotometer (UV-1600) was employed to analyze these supernatants. For comparation, the original MB solution (not participate in any manage) also was judged with the same parameters. Degradation efficiency could be calculated by the way [Citation29]:
(1)
(1)
Where η is the degradation efficiency of catalysts toward MB dye. C and C0 are the concentrations while A and A0 donate the absorbance at irradiation time t and 0, respectively.
3. Results and discussion
3.1. Characterization
First, all as-obtained were analyzed by a powder X-ray diffraction (XRD). Attested in , it turns out that all the samples AgI, Ag3BiO3 and Ag/AgI/ABO were synthesized successfully already. The intense diffraction peaks of AgI match with JCPDS card no. 09-0374 and align well with previous reports [Citation30,Citation31]. Located at 28.4°, 33.1°, 33.6°, 34.8° and 53.4° of 2θ degree, the characteristic peaks agree with (312), (431), (332), (422) and (372) planes, respectively, of Ag3BiO3(JCPDS card no. 49-0140). Remarkably, as the increasing contents of AgI, the height of major diffraction peaks of Ag3BiO3 were increasingly lower in the Ag/AgI/ABO composites, indicating the size or clusters of Ag3BiO3 were greatly affected by the AgI contents. Gone were the (101) and (103) peaks of Ag/AgI/ABO, may revealing traces of sliver ions from AgI were reduced to metallic sliver because it is a sensitizer [Citation32].
Second, in order to further prove the existence of metallic sliver and to analyze the elemental compositions and chemical status, Ag/AgI/ABO-3 composite was characterized by XPS. The full-range XPS spectrum (See ) confirms only I, Ag, O, Bi and a carbon (indicator) peak existence, revealing that the chemical states of all elements are involved. From the high-resolution XPS spectra of O 1s in , it is resolved into a couple of peaks located at 532.6, 531.0 and 529.4 eV. The higher banding energy of 532.6 eV ascribed to oxygen complexes absorbed surface such as OH, H2O, and O2 and the peaks at 531.0 and 529.4 eV respectively stemmed from Ag-O and Bi-O bonds in Ag3BiO3 [Citation33,Citation34]. The high-resolution XPS spectrum (see ) for I 3d3/2 and 3d5/2 are observed at 631.0 and 619.9 eV respectively, ascribed to the I− in AgI [Citation35,Citation36]. The XPS spectrum of Bi 4f, shows that the binding energies of Bi 4f5/2 and 4f7/2 are distinctly observed at 164.0 and 158.7 eV respectively, ascribed to the Bi3+ in Ag3BiO3. As is shown in , the peaks centered about at 374.8 and 368.7 eV are respectively coincident with the Ag 3d3/2 and 3d5/2, which are ascribed to the Ag+ [Citation37]. Moreover, the Ag 3d5/2 high-resolution XPS spectra is also resolved a slight peak located at 367.9 eV, revealing a bit Ag0 have yielded in Ag/AgI/Ag3BiO3 [Citation38,Citation39] and that is corresponded to the XRD result.
3.2. Morphology analysis
displays the microcosmic morphology features of Ag3BiO3, AgI and Ag/AgI/ABO-3 composites. As is shown in , Ag3BiO3 with aggregation is a nanoparticle ranged from 200 to 500 nm. exhibits that the morphology of pristine AgI is a glossy irregular particle. The formed heterojunction can be found to be comprised by metallic Ag, Ag3BiO3 and AgI (See ). The morphologies of AgI particles obviously change after imbedding Ag3BiO3 nanoparticles over it. On the one hand, the AgI is surrounded by a mount of nanoparticles and the quantity of its crystallographic planes has increased. On the other hand, there are trace of fine nanoparticles, metallic Ag, implanted on the surface of AgI, which is wonderful to consistent with XRD and XPS. It proves a fact that a heterojunction in Ag/AgI/ABO can promote the separation and transmission efficiency of electron-holes, thereby enhancing the photocatalytic performance.
3.3. UV–vis diffuse analysis
In order to obtain the band gap energies of photocatalysts, optical absorption studies in the UV–vis region was carried out, shown in . AgI holds a sharp adsorption edge with wavelengths presumably 450 nm, which is in accordance with report [Citation40]. For Ag3BiO3 nanoparticles, its absorption band edge onset about at 600 nm, displays stronger absorption than AgI in visible region. Obviously, compared to intrinsic absorption intensity of AgI, the Ag/AgI/ABO-3 heterojunction shows stronger absorbance in visible region, which coincident with the spread of absorption in visible-light region. Therefore, it features the great enhancement of photocatalytic performance, attributed to the strong light harvesting property of Ag3BiO3. The valence band and conduction band both of Ag3BiO3 and AgI are estimated by Butler and Ginley model using formula (2) and (3) [Citation41]:
(2)
(2)
(3)
(3)
of which Ec, χ, E0, Eg and Ev are conduction band energy, pearson absolute electronegativity of samples, the reduction potential of water, the band energy of as-obtained sample and valence band energy, respectively. Here, χ values are 5.615 eV and 5.479 eV for Ag3BiO3 and AgI, respectively. According to the above rules, the available Eg of AgI is 2.74 eV, Ec and Ev of Ag3BiO3 are 0.19 eV, 2.05 eV, respectively.
3.4. Photocatalytic activity of photocatalyst
At this work, MB dye as an objective contaminant was used for evaluating the as-obtained photocatalytic performance. Control experiments were carried out and it implied that MB dye couldn’t be mineralized in the absence of the as-obtained samples. From the it can be seen, every doping sample’s photocatalytic activities for MB dye outperform its individual counterparts. In contrast, Ag/AgI/ABO-3 photocatalyst is the best one to degrade MB dye at all samples exposing visible illumination. For Ag/AgI/ABO-3, it exhibits highest photocatalytic performance rate of 0.02723 min−1 with 80.5 percent degradation, which much exceled that of bare AgI and Ag3BiO3 (See ). Accordingly, locating at 664 nm is the characteristic absorbance peak of MB dye [Citation42]. describes the time-dependent UV-Vis absorption spectra evolution curves of MB dye in the degradation system that Ag/AgI/ABO-3 involves. It could be seen that slight hypsochromic effects of MB occurred as MB was gradually demethylated [Citation43,Citation44]. The SEM image of Ag/AgI/ABO (See ) offers an indication that a heterojunction accelerates the separation of electron hole pairs, thus improving the photocatalytic activities of dopants. Moreover, the mineralization level of the Ag/AgI/ABO-3 photocatalyst to MO was investigated to determine the mineralization ratio of the degraded MB [Citation45]. As total organic carbon (TOC) analysis pattern shows in , the TOC value fell by 16.48 percent after an hour visible light irradiation. This result indicates that Ag/AgI/ABO-3 photocatalyst was easy to mineralize MB dye solution.
Figure 5. Photocatalytic activities of different samples for MB under visible-light irradiation (a), time-dependent UV-Vis absorption spectra for the degradations of MB over Ag/AgI/Ag3BiO3-3 catalyst (b), first-order rate constant of different as-obtained samples (c) and Change of TOC with irradiation time (d).
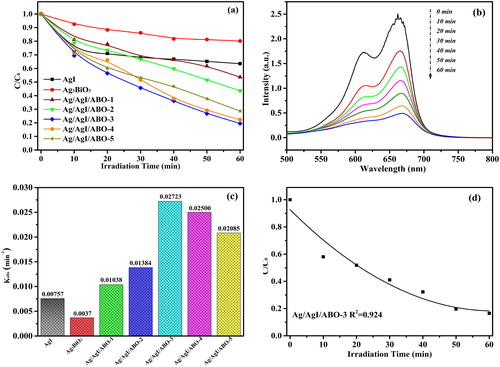
3.5. Active species analysis
Generally, the reactive species on the surface of as-prepared photocatalysts play an active role in photocatalytic process. Therefore, different scavengers were brought in photocatalytic reaction system to affirm which kinds of species at work. Popularly, isopropanol (IPA), ammonium oxalate (AO) and benzoquinone (BQ) were used for capturing hydroxyl (•OH), holes (h+) and superoxide (•O2-), respectively. As is shown in , the photocatalytic performance is slightly reduced when IPA and BQ are introduced into photocatalytic system, which indicates •OH and •O2- made segmental influence on photodecomposition process. On the other hand, the photocatalytic performance is obviously declined when AO is supplied for photodecomposition process, validating that holes are the predominant work-functional species. Therefore, a feasible photocatalytic mechanism can be proposed for the Ag/AgI/ABO photocatalytic reactions.
3.6. Reaction mechanism
In light of the discussion above, schematically shows the feasible photocatalytic mechanism to illustrate the superior photocatalytic performance of MB dye over Ag/AgI/ABO material. Nevertheless, photogenic electrons and holes are effortless to recombination due to the narrow band gap level of Ag3BiO3 material. A type-I heterojunction is firstly employed to explain the photocatalytic mechanism on Ag/AgI/ABO. According to the alignment of both Ag3BiO3 and AgI electronic structures, they can be excited synchronously by solar light (λ > 420 nm). As is shown in , photons are absorbed by Ag3BiO3 and AgI under visible-light illumination. Simultaneously, the electron-hole pairs yield on both Ag3BiO3 and AgI interface. In addition, because of Ag0 SPR, electrons on the CB of Ag can migrate to that of AgI. Since the CB of Ag3BiO3 is more positive than that of AgI, the number of electrons transfers to the CB of Ag3BiO3. Unluckily, it is hardly for Ag3BiO3 to reduce O2 into •O2− as the potential level of it is less negative than that of •O2−/O2 (-0.33 eV vs. NHE) [Citation46,Citation47], which against the consequence of trapping experiments. Besides, holes on the VB of AgI can move to that of Ag3BiO3, posed the recombination of electrons and holes, which is not beneficial for photocatalytic reaction. The conditions are similar with the report [Citation48]. Consequently, it is most possible that Z-Scheme heterojunction plays a significant role in photocatalytic process. As is shown in , the electrons on the CB of Ag3BiO3 migrate to the VB of Ag. Under visible illumination, the electrons on the VB of excited Ag can be transferred to CB of AgI in the help of Ag0 SPR. It is well-known that electron possesses powerful reduction ability. The absorbed O2 is reduced into •O2− by electrons on the CB of AgI. In line with active species trapping experiments, both •O2− and •OH are considered as secondary radical species, and holes are the dominant active species in photocatalytic process. As we can see, the photo-generated holes of Ag3BiO3 transfer to AgI on the VB. The separation of electron-hole pairs maintains a transferable cycle, which effectively inhibits the recombination of electron-hole pairs.
In order to investigate the separation efficiency and transport properties of charge carries, the PL emission spectra shows in . A weaker PL intensity reflects a higher lifetime of photo-excited charge-carrier [Citation49,Citation50]. It can be seen clearly that the PL peak intensity of Ag3BiO3 is the strongest, followed by AgI, which indicates that the photogenic electron-hole pairs are easy to recombine. However, the PL peak of Ag/AgI/ABO-3 is significantly weakened, indicating the recombination of photogenic electron-hole pairs over Ag/AgI/ABO-3 can be inhibited validly. In addition, as illustrated in , the EIS Nyquist plot of samples are semicircles and the radius value of Ag3BiO3 is the biggest, followed by AgI, indicating their impedance and capacitive reactance bigger than the composites. Namely, the efficient charges separation both of Ag3BiO3 and AgI are poor compared to Ag/AgI/ABO, which conforms to the photocatalytic performance results. It's worth noting that the radius of Ag/AgI/ABO-3 is the smallest among all samples, revealing that there emerges a significantly efficient charges separation, which attributes to the function of Z-scheme heterojunction. Moreover, moderate amount of Ag0 nanoparticles anchored on AgI surfaces serve as charge carriers separation centers, facilitating the surface electron excitation and interfacial transfer with the effect of SPR, also presented by PL [Citation51].
In a word, the Ag/AgI/ABO photocatalyst has formed Z-scheme heterojunction which advanced the electron and hole pairs’ transport property, along with the enhancement of the photocatalytic performance. The feasible mechanism was demonstrated by PL and EIS.
4. Conclusions
The Ag/AgI/ABO photocatalyst was fabricated by an in-situ precipitation method for the first time. Ag0 nanoparticles yielded during synthetic process. These appreciate metallic Ag0 nanoparticles acted as charge carrier separation center over SPR which accelerated the interfacial electrons transfer to other electron acceptors. The Z-scheme heterojunction has formed in Ag/AgI/ABO where the electron-hole pairs were able to be separated and migrated efficiently. Thus, the as-prepared exhibited superior photocatalytic performance to the MB dye degradation over Ag/AgI/ABO. Holes that were dominant radical species played an important role in the process degradation of MB dye. Enhancing the stability of the photocatalyst is a huge challenge. In shortly, the Ag/AgI/ABO photocatalyst is a utility material to curb environmental issue.
Conflicts of interest
The authors declare no conflict of interest.
Acknowledgments
This work was financially supported by Natural Science Foundation of Changchun Normal University ([2009]005, [2010]009).
References
- Arabzadeh A, Salimi A. One dimensional CdS nanowire@TiO2 nanoparticles core-shell as high performance photocatalyst for fast degradation of dye pollutants under visible and sunlight irradiation. J Colloid Interface Sci. 2016;479:43–54.
- Chao J, Liu G, Zu L, et al. Preparation of Ag@Ag3PO4@ZnO ternary heterostructures for photocatalytic studies. J Colloid Interface Sci. 2015;453:36–41.
- Kun R, Balázs M, Dékány I, et al. Photooxidation of organic dye molecules on TiO2 and zinc–aluminum layered double hydroxide ultrathin multilayers. Colloid Surface A: Physicochem Eng Aspects. 2005;265:155–162.
- Yu Z, Kumar R, Chu Y, et al. Photocatalytic decomposition of RhB by newly designed and highly effective CF@ZnO/CdS hierarchical heterostructures. ACS Sustain Chem Eng. 2018;6:155–164.
- Chen S, Hu Y, Ji L, et al. Preparation and characterization of direct Z-scheme photocatalyst Bi2O3/NaNbO3 and its reaction mechanism. Appl Surf Sci. 2014;292:357–366.
- Cao S, Zhu Y. Hierarchically nanostructured r-Fe2O3 hollow spheres: preparation, growth mechanism, photocatalytic property, and application in water treatment. J Phys Chem C. 2008;112:6253–6257.
- Cao J, Xu B, Luo B, et al. Preparation, characterization and visible-light photocatalytic activity of AgI/AgCl/TiO2. Appl Surf Sci. 2011;257:7083–7089.
- Liang W, Tang G, Zhang H, et al. Core–shell structured AgBr incorporated g-C3N4 nanocomposites with enhanced photocatalytic activity and stability. Mater Technol. 2017;32:675–685.
- Ghanbari M, Soofivand F, Salavati-Niasar M. Simple synthesis and characterization of Ag2CdI4/AgI nanocomposite as an effective photocatalyst by co-precipitation method. J Mol Liq. 2016;223:21–28.
- Shinger MI, Idris AM, Devaramani S, et al. In situ fabrication of graphene-based Ag3PO4@AgBr composite with enhanced photocatalytic activity under simulated sunlight. J Environ Chem Eng. 2017;5:1526–1535.
- Xu F, Xiao W, Cheng B, et al. Direct Z-scheme anatase/rutile bi-phase nanocomposite TiO2 nanofiber photocatalyst with enhanced photocatalytic H2-production activity. Int J Hydrogen Energy. 2014;39:15394–15402.
- Song Z, He Y. Novel AgCl/Ag/AgFeO2 Z-scheme heterostructure photocatalyst with enhanced photocatalytic and stability under visible light. Appl Surf Sci. 2017;420:911–918.
- Zhang W, Zhou L, Shi J, et al. Fabrication of novel visible-light-driven AgI/g-C3N4 composites with enhanced visible-light photocatalytic activity for diclofenac degradation. J Colloid Interface Sci. 2017;496:167–176.
- Guo F, Shi W, Wang H, et al. Study on highly enhanced photocatalytic tetracycline degradation of type II AgI/CuBi2O4 and Z-scheme AgBr/CuBi2O4 heterojunction photocatalysts. J Hazard Mater. 2018;349:111–118.
- Fang H, Gao X, Yu J, et al. Preparation of the all-solid-state Z-scheme WO3/Ag/AgCl film on glass accelerating the photodegradation of pollutants under visible light. J Mater Sci. 2019;54:286–301.
- Reddy D, Choi J, Lee S, et al. Green synthesis of AgI nanoparticle-functionalized reduced graphene oxide aerogels with enhanced catalytic performance and facile recycling. RSC Adv. 2015;5:67394–67404.
- Dong F, Li Q, Zhou Y, et al. In situ decoration of plasmonic Ag nanocrystals on the surface of (BiO)2CO3 hierarchical microspheres for enhanced visible light photocatalysis. RSC Dalton Trans. 2014;43:9468–9480.
- Du X, Wan J, Jia J, et al. Photocatalytic degradation of RhB over highly visible-light active Ag3PO4-Bi2MoO6 heterojunction using H2O2 electron capturer. Mater Design. 2017;119:113–123.
- Kim TW, Choi KS. Nanoporous BiVO4 photoanodes with Dual-Layer oxygen evolution catalysts for solar water splitting. Science. 2014;343:990–994.
- Li X, Huang R, Hu Y, et al. A templated method to Bi2WO6 hollow microspheres and their conversion to double-shell Bi2O3/Bi2WO6 hollow microspheres with improved photocatalytic performance. ACS Inorg Chem. 2012;51:6245–6250.
- Li J, Yuan H, Zhu Z, et al. Improved photoelectrochemical performance of Z-schemeg-C3N4/Bi2O3/BiPO4 heterostructure and degradation property. Appl Surf Sci. 2016;385:34–41.
- Gong JY, Lee CS Kim EJ, et al. Self-generation of reactive oxygen species on crystalline AgBiO3 for the oxidative remediation of organic pollutants. ACS Appl Mater Interfaces. 2017;9:28426–28432.
- Rurtz M, Jansen M. Ag3BiO3, und Ag5BiO4, die ersten Siiberoxobismutate(III). Z anorg allg Chem. 1993;619:1446–1454.
- Li C, Zhang A, Zhang L, et al. Enhanced photocatalytic activity and characterization of magnetic Ag/BiOI/ZnFe2O4 composites for Hg0 removal under fluorescent light irradiation. Appl Surf Sci. 2018;433:914–926.
- Ge M, Cao C, Li S, et al. In situ plasmonic Ag nanoparticles anchored TiO2 nanotube arrays as visible-light-driven photocatalysts for enhanced water splitting. RSC Nanoscale. 2016;8:5226–5234.
- Sellappan R, Nielsen MG, Posada FG, et al. Effects of plasmon excitation on photocatalytic activity of Ag/TiO2 and Au/TiO2 nanocomposites. J Catal. 2013;307:214–221.
- Liu S, Yu J, Wang T, et al. A multifunctional Ag/PAOCG reusable substrate for p-nitrophenol reduction and SERS applications. J Mater Sci. 2017;52:13748–13763.
- Song B, Tang Q, Wu W, et al. A novel in-situ synthesis and enhanced photocatalytic performance of Z-scheme Ag/AgI/AgBr/sulfonated polystyrene heterostructure photocatalyst. J Inorg Organomet P. 2018;28:805–811.
- Chen F, Yang Q, Sun J, et al. Enhanced photocatalytic degradation of tetracycline by AgI/BiVO4 heterojunction under visible-light irradiation: mineralization efficiency and mechanism. ACS Appl Mater Interfaces. 2016;8:32887–32900.
- Zhang A, Zhang L, Chen X, et al. Photocatalytic oxidation removal of Hg0 using ternary Ag/AgI-Ag2CO3 hybrids in wet scrubbing process under fluorescent light. Appl Surf Sci. 2017;392:1107–1116.
- Cui M, Yu J, Lin H, et al. In-situ preparation of Z-scheme AgI/Bi5O7I hybrid and its excellent photocatalytic activity. Appl Surf Sci. 2016;387:912–920.
- Yang N, Lv X, Zhong S, et al. Preparation of Z-scheme AgI/Bi5O7I plate with high visible light photocatalytic performance by phase transition and morphological transformation of BiOI microspheres at room temperature. RSC Dalton Trans. 2018;47:11420–11428.
- Dai K, Lu L, Liang C, et al. A high efficient graphitic-C3N4/BiOI/graphene oxide ternary nanocomposite heterostructured photocatalyst with graphene oxide as electron transport buffer material. Dalton T. 2015;44:7903–7910.
- Islam M, Reddy D, Ma R, et al. Reduced-graphene-oxide-wrapped BiOI-AgI heterostructured nanocomposite as a high-performance photocatalyst for dye degradation under solar light irradiation. Solid State Sci. 2016;61:32–39.
- Xu H, Yan J, Xu Y, et al. Novel visible-light-driven AgX/graphite-like C3N4 (X = Br, I) hybrid materials with synergistic photocatalytic activity. Appl Catal B-Environ. 2013;129:182–193.
- Wang P, Huang B, Zhang Q, et al. Highly efficient visible light plasmonic photocatalyst Ag@Ag(Br,I). Chem Eur J. 2010;16:10042–10047.
- Reddy D, Ma R. Choi M, et al. Reduced graphene oxide wrapped ZnS–Ag2S ternary composites synthesized via hydrothermal method: Applications in photocatalyst degradation of organic pollutants. Appl Surf Sci. 2015;324:725–735.
- Li T, Lu G, Hu X, et al. Ag/Ag3PO4/BiPO4 nanocomposites with high photocatalytic degradation activity for 2,4-dichlorophenol. Mater Lett. 2017;188:392–395.
- Wang Z, Zhang J, Lv J, et al. Plasmonic Ag2MoO4/AgBr/Ag composite: Excellent photocatalytic performance and possible photocatalytic mechanism. Appl Surf Sci. 2017;396:791–798.
- Liu L, Qi Y, Yang J, et al. An AgI@g-C3N4 hybrid core@shell structure: Stable and enhanced photocatalytic degradation. Appl Surf Sci. 2015;358:319–327.
- Di L, Yang H, Xian T, et al. Photocatalytic and photo-fenton catalytic degradation activities of Z-scheme Ag2S/BiFeO3 heterojunction composites under visible-light irradiation. Nanomaterials-Basel. 2019;9:399.
- Kumar S, Khanchandani S, Thirumal M, et al. Achieving enhanced visible-light-driven photocatalysis using type-II NaNbO3/CdS core/shell heterostructures. ACS Appl Mater Interfaces. 2014;6:13221–13233.
- Zhang T, Oyama T, Horikoshi S, et al. Photocatalyzed N-demethylation and degradation of methylene blue in titania dispersions exposed to concentrated sunlight. Sol Energy Mat Sol C. 2002;73:287–303.
- Choi J, Reddy D, Kim T. Enhanced photocatalytic activity and anti-photocorrosion of AgI nanostructures by coupling with graphene-analogue boron nitride nanosheets. Ceram Int. 2015;41:13793–13803.
- Reddy D, Lee S, Choi J, et al. Green synthesis of AgI-reduced graphene oxide nanocomposites:Toward enhanced visible-light photocatalytic activity for organicdye removal. Appl Surf Sci. 2015;341:175–184.
- Yangjeh AH, Akhundi A. Novel ternary g-C3N4/Fe3O4/Ag2CrO4 nanocomposites: magneticallyseparable and visible-light-driven photocatalysts for degradation of water pollutants. J Mol Catal A-Chem. 2016;415:122–130.
- Zhu B, Xia P, Li Y, et al. Fabrication and photocatalytic activity enhanced mechanism of direct Z-scheme g-C3N4/Ag2WO4 photocatalyst. Appl Surf Sci. 2017;391:175–183.
- Islam M, Reddy A, Choi J, et al. Surface oxygen vacancy assisted electron transfer and shuttling for enhanced photocatalytic activity of a Z-scheme CeO2–AgI nanocomposite. RSC Adv. 2016;6:19341–19350.
- Reddy D, Choi J, Lee S, et al. Controlled synthesis of heterostructured Ag@AgI/ZnS microspheres with enhanced photocatalytic activity and selective separation of methylene blue from mixture dyes. J Taiwan Inst Chem E. 2016;66:200–209.
- Lee S, Reddy A, Kim T. Well-wrapped reduced graphene oxide nanosheets on Nb3O7(OH) nanostructures as good electron collectors and transporters for efficient photocatalytic degradation of rhodamine B and phenol. RSC Adv. 2016;6:37180–37188.
- Liu H, Cao W, Su Y, et al. Synthesis, characterization and photocatalytic performance of novel visible-light-induced Ag/BiOI. Appl Catal B-Environ. 2012;111-112:271–279.