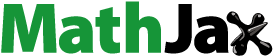
Abstract
Mesoporous titania–ceria mixed oxide (MTCMO) was synthesised in a sol–gel method using cerium chloride and titanium isopropoxide (TTIP) as CeO2 as well as TiO2 precursors and sodium dodecyl sulphate (SDS) as a structure-directing agent and its application was evaluated in the synthesis of aromatic β-phosphonomalonate derivatives via cascade Knoevenagel–phospha-Michael addition reaction. The catalyst characterised by various techniques like FT-IR spectroscopy, XRD, SEM, EDS, TEM and N2 adsorption-desorption. Partial substitution of Ti4+ cations with Ce4+ improved the BET surface area because TiO2 crystallites were suppressed to be grown. Furthermore, as a consequence of charge imbalance in the obtained Ti-O-Ce solid solution, some oxygen vacancies have generated that act as Lewis base sites while cations (Ti and Ce) around the oxygen vacancies act as Lewis acid sites. Reusability up to eight consecutive runs without significant decrease in reactivity, Mild reaction conditions, environmentally benign and compatibility with various functional groups are some of the advantages of this work.
1. Introduction
The stringent environmental regulations and economic issues compel the industry as well as academic researchers to use sustainable catalysts with high activity, compatibility with the environment, reusability and convenient separation procedure from the product. The exploration of porous materials serves great opportunities in the heterogeneous catalysis thanks to their intrinsic features especially the presence of active sites on their surface. Based on the pore size, International Union of Pure and Applied Chemistry (IUPAC) has classified the porous solid materials into three categories of microporous (<2 nm), mesoporous (2–50 nm) and macroporous (>50 nm) [Citation1]. Although many methods are available for the preparation of the mesoporous materials, soft-templating method is the most important method in which the organic surfactant molecules act as template or structure-directing agent to generate porosity in the synthesis of siliceous (eg MCM-41, MCM-48, SBA-15, etc.) and non-siliceous mesoporous material (eg metal oxides, mixed metal oxides, etc.) [Citation2].
Mesoporous materials with high BET surface area have been investigated in various applications such as adsorption [Citation3], removal of pollutant ions [Citation4], electronics [Citation5], photocatalysis [Citation6], energy storage [Citation7] and energy conversion [Citation8]. Furthermore, mesoporous materials are used in a broad spectrum of organic transformations such as oxidation reactions [Citation9–12], hydrogenation reactions [Citation13], ring-opening [Citation14], coupling reactions [Citation15–18], acid–base reactions such as Friedel–Crafts reaction [Citation19], esterification [Citation20] and transesterification reactions [Citation21]. Mesoporous materials are also used as catalyst in the other reactions [Citation22, Citation23].
Multi-component reactions (MCRs) are employed to construct target compounds with developed efficiency and atom economy from simple precursors in a single step pathway [Citation24–26]. Among the organophosphorus compounds, phosphonates are highly valuable because of their broad range of applications in material chemistry [Citation27], catalysis [Citation28, Citation29], enzyme inhibitors [Citation30], peptide mimics [Citation31], metabolic probes [Citation32], antibiotics [Citation33] and pharmacologic agents [Citation34]. Several synthetic methods have been developed for the preparation of phosphonates. Of these, the phosphorus-carbon (P-C), bond formation by direct phospha-Michael addition is one of the prevalent methods [Citation35–37]. Two-step procedures are common to obtain the β-phosphonomalonates during a nucleophilic attack of phosphorus nucleophiles to α,β-unsaturated malonates [Citation38, Citation39]. Step-by-step isolations and purifications of product and catalysts restrict overall efficiency of this method. To reduce limitations and improve overall efficiency, one-pot reaction found to be an ideal substitute. One-pot synthesis of β-phosphonomalonates via tandem Knoevenagel–phospha-Michael reaction is precious in this field [Citation40–42]. Despite the efficiency of the reported methods, however, some of the disadvantages such as low yields, long reaction time, special reaction conditions, unrecyclable catalysts and use of toxic solvents are still remained. To exploit the valuable advantages of mesoporous materials, herein, we develop a mesoporous titania–ceria mixed oxide (MTCMO). The material was synthesised through soft-templating method using sodium dodecyl sulphate (SDS) a structure-directing agent. After the characterisation of material, its application was examined in the synthesis of β-phosphonomalonates through a one-pot procedure (Scheme 1).
2. Results and discussion
2.1. Characterisation of MTCMO
FT-IR spectra MTCMO, CeO2 and TiO2 nanoparticles are shown in , respectively. FTIR of MTCMO () shows two bands at 3421 and 1636 cm−1 owing to OH banding vibration and physical adsorbed water during gelation process [Citation43]. The band at 2978 cm−1 is attributed to remaining alkyl group of surfactant. The vibration band at 615 cm−1 can be attributed to the vibration absorption peak of Ti–O–Ti and Ce–O–Ce with an overlapping. The weak band at 1057 cm−1 is assigned to symmetrical stretching adsorption of S═O [Citation44]. As shown in the FTIR spectra of samples, a shift to higher wave numbers in the bands position of samples from TiO2 to MTCMO was observed. This change may be attributed to the change in the particle size or aggregation by the addition of CeO2 NPs [Citation45] which also supported by XRD data.
XRD spectra MTCMO, CeO2 and TiO2 nanoparticles are shown in , respectively. In the XRD pattern of MTCMO (), the anatase phase of TiO2 is clearly obvious. Rutile lines such as 110 are not observed. Diffraction peaks in the XRD pattern of TiO2 at 2θ = 26.1ο, 39.8ο and 62.3ο are indexed to (101), (004) and (204 planes, respectively. These peaks are attributed to body-centred tetragonal anatase TiO2 (JCPDS card no. 21-1272). The diffraction peaks appeared at 2θ = 28.3ο, 33.8ο, 47.3ο, 73.8ο and 77.8ο are attributed to (110), (200), (220), (400) and (331) planes of the face centred cubic CeO2 (JCPDS card no. 81-0792), respectively. Using the Scherrer’s equation (EquationEquation (1)(1)
(1) ), the crystallite size (D) of samples was calculated.
(1)
(1)
Where θ is Bragg’s angle, K is Scherrer constant which is 0.9, λ = 0.15406 nm and β is diffraction peak full width at half maximum (FWHM). Using Scherrer’s equation, the crystallite size (D) of TiO2 and CeO2 nanoparticles was found to be 11 and 7 nm, respectively. Additionally, the crystallite size of MTCMO was found to be 9 nm. The decrease in crystal size can be attributed to the presence of Ti–O–Ce in the Ce-doped TiO2 [Citation46].
The morphology and structure of MTCMO were studied by SEM () and TEM (). SEM image shows the ordered morphology of MTCMO with acceptable manometer particle size in the range of 60–80 nm. TEM image shows the existence of discrete pores in the structure of MTCMO.
In order for further characterisation of MTCMO, energy-dispersive X-ray spectroscopy (EDX) was carried out. The spectrum () shows the characteristic peaks for the Ti, Ce and O, confirming the formation of TiO2–CeO2 structure. The results of EDX analysis are depicted in .
Table 1. The EDX data of MTCMO.
Textural properties of MTCMO were explored by N2 adsorption–desorption analysis and pore size distribution was calculated by Barrett–Joyner–Halenda (BJH) method. The N2 adsorption–desorption isotherm of MTCMO () is classified as a type IV isotherm, representative of mesoporous materials with an H2 hysteresis loop which indicates a narrow pore-size distribution. BET surface area of MTCMO is great value of 445.73 m2.g−1 and by our knowledge no sample of TiO2–CeO2 with this surface area is reported by now. Incorporation of Ce (Ce3+ and Ce4+) into the titania lattice prevents the growth of TiO2 crystallites [Citation47], and correspondingly leads to a decrease of crystallite size and thus increasing the BET surface area [Citation48]. Pore size distribution curve shows a maximum pore width at 1.29 nm. Mean pore diameter was 8.3506 nm which clearly indicates the mesoporosity of MTCMO. The higher BET surface area and larger pore volume result in more efficient distribution and interaction of substrates and intermediates with the surface of the catalyst [Citation49]. To show the effect of surfactant as an important factor for improving the surface area and total pore volume we synthesised a sample of TiO2–CeO2 mixed oxide (TCMO) but in this case without using surfactant. BET surface area of TCMO was 88.7465 m2.g−1 that showed a decrease as compared to the BET surface area of MTCMO. In addition, total pore volume of TCMO at P/P0 =0.99 was 0.169425 cm³/g which showed a decrease as compared to total pore volume of MTCMO which was 0.9305 cm³/g at P/P0 = 0.99. Higher surface area provides more available active sites in the catalyst and therefore we expect that MTCMO should be more active relative to TCMO.
2.2. Catalytic studies
In the next step, to find the effect of nature of catalyst on the promotion of β-phosphonomalonate synthesis, we carried out a set of investigations on a model reaction between benzaldehyde, malonitrile and triethylphosphite to find the most effective catalyst. The reactions were performed with TiO2, CeO2, TCMO and MTCMO. For the synthesis of TCMO no surfactant was used, while the other samples synthesised using SDS as a structure-directing and pore-forming agent. All the reactions were performed using 2 mol% of each catalyst under the solvent-free conditions at 50 οC for 2 h. The reaction under the catalyst-free condition afforded the desired product in the low yield (, entry 1). When TiO2 or CeO2 nanoparticles or TiO2+CeO2 mixture (, entries 2–4) were used, the desired product obtained with moderate yields. The synergic effect of CeO2 and TiO2 improved the yield of product (, entry 4). From the obtained results (, entry 6), MTCMO was found to be the most effective catalyst. Interestingly, with TCMO as catalyst (, entry 5), the desired product obtained only with 35% yield that highlights the effect of surface area provided by availability of more active sites and pores that generated when SDS was used. Further studies showed that, in addition to surface area, MTCMO is superior catalyst over TCMO in the case of catalyst loading as the desired product obtained with 75% yield when 4 mol% of TCMO was used in the ethanol as solvent and 80 οC after 2 h. CeO2 nanoparticles mainly contain a mixture of Ce3+ and Ce4+ ions on their surface [Citation50]. Ce3+ ions are larger than Ce4+ ions and a result of oxygen vacancies. The higher ratio of Ce3+/Ce4+ improves the catalytic activity of CeO2 owing to the fact that Ce3+ ions generate more strain on the lattice of CeO2 [Citation51]. The ionic radius of Ce4+ (1.01 Å) is larger than Ti4+ (0.68 Å). Therefore, the incorporation of Ce4+ cations into the crystalline structure of TiO2 leads to the substitution of some of the Ti4+ cations and expands the TiO2 lattice that consequently decreases the symmetry and makes disorder in the titanium sites [Citation52].
Table 2. Screening of different catalysts for a model reaction between benzaldehyde, malonitrile and triethylphosphite.Table Footnotea
Additionally, the physicochemical properties and catalytic behaviour of the obtained solid solution Ti–O–Ce are different from their individual components [Citation53, Citation54]. The larger electronegativity of Ti as compared to the electronegativity of Ce results in a charge imbalance at Ti–O–Ce bond and induces more defects like unsaturated chemical bonds [Citation55]. Furthermore, the electron charge density of Ti4+ is increased in the Ti–O–Ce bond, making Ti4+ the electronegative center and Ce4+ as the electropositive center because Ti4+ strongly pulls the electrons of oxygen [Citation56]. The increased electron charge density around the Ti4+ gives rise to a bond shortening as reported by Romero-Galarza et al. [Citation57] and as a result of bond shortening, some structural defects such as oxygen vacancies and reduction of Ti4+ ions are observed [Citation48, Citation58]. By removing oxygen from the surface of a metal oxide an oxygen vacancy is generated in which two electrons are remained. The unpaired electrons left behind after removing the oxygen atom act as Lewis base sites and cations around the oxygen vacancies act as Lewis acid sites [Citation59, Citation62].The reactions catalyzed by defective surface sites profit from lower energy barriers relative to the similar reactions catalyzed by non-defective surfaces [Citation63–65]. Therefore, by incorporation of Ce4+ ions into the structure of TiO2 the BET surface area improved, structural defects generated, and consequently a better interaction between the surface of the catalyst and substrates lowered the energy barrier of reaction.
In the next step, the effect of solvent, temperature and amount of catalyst was studied (). First, equivalent reactions were performed with 2 mol% of the catalyst in the various solvents as well as solvent-free conditions at 50 °C. As shown, the reaction proceeded with better yield in ethanol as compared to the other solvents (, entries 1–6). By increasing the amount of the catalyst to 3 mol% an improvement in the yield of product was observed (, entry 7), and by increasing the temperature to 60 °C the best result was obtained (, entry 8). Therefore, the optimum condition was determined as a reaction with 3 mol% of MTCMO, 1 mmol of aldehyde, 1 mmol of malonitrile and 1 mmol of triethylphosphite in the 5 mL of ethanol at 60 °C.
Table 3. Optimisation of the reaction conditions.Table Footnotea
Under the optimised reaction conditions, we explored the scope and efficiency of MTCMO for the preparation of β-phosphonomalonates from aromatic and heterocyclic aldehydes bearing electron-donating and electron-withdrawing groups (). Aromatic aldehydes with electron-withdrawing groups such as NO2 and –Cl, on the benzene ring gave shorter reaction times and yields as compared with the aldehydes with electron-donating groups like –Me, –OMe and –OH. Heteroaromatic aldehydes, such as thiophene-2-carbaldehyde and furfuraldehyde were also effective substrates to accomplish the tandem Knoevenagel–phospha-Michael (, entries 9 and 10). Electron-withdrawing groups showed more reactivity and afforded higher yields in position 4 of benzene.
Table 4. One-pot synthesis of β-phosphonomalonates catalysed by MTCMO.Table Footnotea
A comparison of the efficiency and applicability of our method with some of the recently reported methods is collected in . As it can be seen, our catalyst system shows better catalytic efficiency in terms of time, yield and catalyst loading. The results are collected for diethyl [2,2-dicyano-1-(4-methoxyphenyl)-ethyl]phosphonate as a model substrate (, entry 6).
Table 5. Comparison of catalytic activity of the MTCMO with some of the recently reported systems.
Furthermore, a comparison between the BET surface area of MTCMO with some similar oxides and mixed metal oxides is collected in . Obviously, the MTCMO (TiO2–CeO2) shows higher BET surface area relative to TiO2 and CeO2. The MTCMO also describes higher BET surface area as compared to TiO2–Fe2O3, TiO2–ZrO2 (95:5 mol%), CeO2–ZrO2 (50:50 mol%), ZrO2 and TiO2.
Table 6. Comparison of the BET surface area between MTCMO and some of similar oxides and mixed oxides.
2.3. Recyclability study of MTCMO
A simple separation procedure from the reaction mixture and efficiency after several cycles are two important factors needed for a heterogeneous catalyst. Recyclability of MTCMO was investigated in a model reaction using benzaldehyde, malonitrile and triethylposphite. After completion of the reaction, the reaction mixture was diluted with ethanol and heated at 80 °C for 10 min. Then, the catalyst was separated by filtration and washed three times with acetone (3 × 10 mL) and EtOAc. Finally, dried in an oven at 80 °C for 3 h. The catalyst was reused up to eight consecutive runs with slight decrease in the efficiency ().
2.4. Possible reaction mechanism over MTCMO
Plausible reaction mechanism for the synthesis of β-phosphonomalonates is depicted in Scheme 2. The reaction profits from the presence of both Lewis acid and Lewis base sites and high BET surface area in the MTCMO catalyst. The aromatic aldehyde is first activated by the coordination of oxygen atom on the Lewis acid sites of MTCMO, followed by nucleophilic attack of activated malonitrile. A Knoevenagel condensation between the aldehyde and malonitrile produces α,β-unsaturated malonates 1. Next, the cyano group of 1 is activated by MTCMO to give the corresponding β-phosphonomalonates through a phospha-Michael addition reaction.
3. Experimental
3.1. General
All commercially available chemicals were purchased from Merck (Kenilworth, NJ) or Aldrich (St. Louis, MO) company and used without further purifications. The products were characterised by comparison of their spectral data and physical properties with those reported in the literature. The progress of the reaction was followed by TLC using Merck 0.2 mm silica gel 60 F-254 Al-plates. Melting points were determined in open capillaries with a stuart scientific SMP2 melting point apparatus and are uncorrected. FT-IR spectra were determined as KBr pellets on a Perkin-Elmer 683 instrument (Perkin-Elmer, Waltham, MA). The crystal structure of the catalyst was analysed on a X’Pert MPD Philips diffractometer with Cu radiation source (λ = 1.54050 Å) at 40 kV voltage and 30 mA current. Field emission scanning electron microscopy measurements (FESEM) were performed on a VEGA TESCAN microscope and TEM micrographs were recorded on a CM120 1998. BET analysis was carried out on a BELOPSOR mini Π Czech 1999. The sample was degassed for 4 h at 423 K before the measurements. EDX was performed on a Philips XL‐300 instrument.
3.2. Preparation of MTCMO
MTCMO was synthesised by following procedure. First, SDS (0.82 g) was dissolved in 5 mL water, then, stirred for 30 min at room temperature to obtain a clear micellar solution. Afterwards, a solution of CeCl3 (1.23 g) dissolved in distilled water (5 mL) was added slowly to the first solution. The resulting mixture was stirred for 30 min. Titanium (iv) isopropoxide (1.42 g) dissolved in 2.5 mL isopropyl alcohol was then added to the premixed solution. The pH of the solution was maintained about 11 by adding 35% NH3.H2O. The resulting mixture was stirred for 3 h and kept on freezing conditions (4 °C) for 48 h. After that, the solid was collected by centrifugation and dried in an oven at 75 °C. The synthesised solid was extracted with ethanol and 2 M HCl. Finally, the solid washed with water and dried at 200 °C to produce mesoporous TiO2–CeO2 mixed oxide.
3.3. Synthesis of TCMO
A solution of CeCl3 (1.23 g) dissolved in distilled water (5 mL) was first prepared. To this solution, a solution of titanium (iv) isopropoxide (1.42 g) dissolved in 2.5 mL isopropyl alcohol was then added and the resulting mixture was stirred for 30 min. Then, the pH of solution was adjusted about 11 by adding 35% NH3.H2O. After stirring for 3 h, the mixture was kept on freezing conditions (4 °C) for 48 h. The solid was then collected by centrifugation and dried in an oven at 75 °C. Finally, the solid washed with water and dried at 200 °C to produce TCMO.
3.4. Synthesis of CeO2 nanoparticles
To synthesise CeO2 nanoparticles, following procedure was used: SDS (0.82 g) was dissolved in 5 mL water and stirred for 30 min at room temperature to obtain a clear micellar solution. To this solution, a solution of CeCl3 (1.23 g) dissolved in distilled water (5 mL) was added slowly and the resulting mixture was stirred for 30 min. By dropwise addition of 35% NH3.H2O, the pH of solution was maintained about 11. The resulting mixture was stirred for 3 h and then the resulted solid was collected by centrifugation. After drying in an oven at 75 °C, the synthesised solid was extracted with ethanol and 2 M HCl. Finally, washed with water and ethanol and dried at 200 °C to produce CeO2 nanoparticles.
3.5. Synthesis of TiO2 nanoparticles
TiO2 nanoparticles were synthesised using this procedure: SDS (0.82 g) was dissolved in 5 mL water and stirred for 30 min at room temperature to obtain a clear micellar solution. To this solution, a solution of titanium (iv) isopropoxide (1.42 g) dissolved in 2.5 mL isopropyl alcohol was then added and the resulting mixture was stirred for 30 min. By dropwise addition of 35% NH3.H2O, the pH of solution was maintained about 11. The resulting mixture was stirred for 3 h and then the solid was collected by centrifugation. After the solid was dried in an oven at 75 °C, it was extracted with ethanol and 2 M HCl. Finally, washed with water and ethanol and dried at 200 °C to produce TiO2 nanoparticles.
3.6. General procedure for the synthesis of β-phosphonomalonates
In a round-bottomed flask, aryl aldehyde (1 mmol), malononitrile (1 mmol, 0.066 g) and triethylphosphite (1 mmol, 0.277 mL) were mixed with MTCMO (0.015 g, 3 mol%) in ethanol (6 mL) and stirred at 60 °C for the appropriate time as shown in . The progress of the reaction was monitored by TLC (n-hexane/ethyl acetate 7:3). After completion of the reaction, ethanol (10 mL) was added to the reaction mixture, and the catalyst was filtered. Then, ethanol was evaporated under reduced pressure, and the crude product was recrystallised from n-hexane and ethyl acetate to obtain the pure product in the excellent yield.
4. Conclusion
In summary, MTCMO was prepared using SDS as a structure-directing agent, cerium chloride as the precursor of CeO2 and titanium isopropoxide (TTIP) as the precursor of TiO2. During the substitution of Ti4+ cations with Ce4+, the BET surface area increased because the TiO2 crystallites were suppressed to be grown. In addition, high surface area and presence of defective sites (reduced cations and oxygen vacancies) improved the reactivity of MTCMO. Furthermore, the catalyst demonstrated both Lewis acid and Lewis base character. The described catalyst was highly efficient for the synthesis of β-phosphonomalonates via cascade Knoevenagel–phospha-Michael addition reaction from triethylphosphite, malonitrile, and various aromatic aldehydes under the mild reaction conditions.
Acknowledgements
The authors acknowledge the Razi University Research Council for support of this work.
Disclosure statement
No potential conflict of interest was reported by the authors.
Additional information
Funding
Notes on contributors
Mohammed Salim Mohammed
Mohammed Salim Mohammed and Mohsen Bakhtiarian are M.Sc. students of organic chemistry under the guidance of Dr. Kiumars Bahrami at faculty of chemistry, Razi university, Kermanshah, Iran. Their interests cover the application of nanocatalysts in organic reactions.
Mohsen Bakhtiarian
Mohammed Salim Mohammed and Mohsen Bakhtiarian are M.Sc. students of organic chemistry under the guidance of Dr. Kiumars Bahrami at faculty of chemistry, Razi university, Kermanshah, Iran. Their interests cover the application of nanocatalysts in organic reactions.
Kiumars Bahrami
Dr. Kiumars Bahrami is working as a Professor of organic chemistry in faculty of chemistry, Razi university, Kermanshah, Iran. He has published many papers in national and international journals. He has also participated and presented several papers at national and international conferences. To date, he has supervised 33 M.Sc. and 12 Ph.D. students. He is developing research programs in the areas of nanostructured materials in organic syntheses.
References
- Rouquerol J, Avnir D, Fairbridge CW, et al. Recommendations for the characterization of porous solids (technical report). Pure Appl Chem. 1994;66(8):1739–1758.
- Pal N, Bhaumik A. Soft templating strategies for the synthesis of mesoporous materials: inorganic, organic–inorganic hybrid and purely organic solids. Adv Colloid Interface Sci. 2013;189:21–41.
- Mironyuk I, Tatarchuk T, Naushad M, et al. Highly efficient adsorption of strontium ions by carbonated mesoporous TiO2. J Mol Liq. 2019;285:742–753.
- Rizzi V, Prasetyanto EA, Chen P, et al. Amino grafted MCM-41 as highly efficient and reversible ecofriendly adsorbent material for the direct blue removal from wastewater. J Mol Liq. 2019;273:435–446.
- Maluta JR, Machado SA, Chaudhary U, et al. Development of a semigraphitic sulfur-doped ordered mesoporous carbon material for electroanalytical applications. Sens Actuator B-Chem. 2018;257:347–353.
- Chen J, Zhan J, Lu E, et al. Facile template-free fabrication of mesoporous ZnCo2O4 fibers with enhanced photocatalytic activity under visible-light irradiation. Mater Lett. 2018;220:66–69.
- Liu M, Wang X, Zhu D, et al. Encapsulation of NiO nanoparticles in mesoporous carbon nanospheres for advanced energy storage. Chem Eng J. 2017;308:240–247.
- Li W, Liu J, Zhao D. Mesoporous materials for energy conversion and storage devices. Nat Rev Mater. 2016;1(6):16023.
- Modak A, Nandi M, Bhaumik A. Titanium containing periodic mesoporous organosilica as an efficient catalyst for the epoxidation of alkenes. Catal Today. 2012;198(1):45–51.
- Geng L, Zhang X, Zhang W, et al. Highly dispersed iron oxides on mesoporous carbon for selective oxidation of benzyl alcohol with molecular oxygen. Chem Commun. 2014;50(22):2965–2967.
- Ayala V, Corma A, Iglesias M, et al. Mesoporous MCM-41-heterogenised (salen) Mn and Cu complexes as effective catalysts for oxidation of sulfides to sulfoxides: isolation of a stable supported Mn(V)O complex, responsible of the catalytic activity. J Mol Catal A Chem. 2004;221:201–208.
- Borah P, Ma X, Nguyen KT, et al. A vanadyl complex grafted to periodic mesoporous organosilica: a green catalyst for selective hydroxylation of benzene to phenol. Angew Chem. 2012;124(31):7876–7881.
- Rakitin MY, Doluda VY, Tyanina A, et al. Ruthenium-catalyzed hydrogenation of nitrobenzene in a supercritical carbon dioxide medium. Russ J Phys Chem B. 2017;11:1113–1116.
- Lee SU, Lee YJ, Kim JR, et al. Selective ring opening of phenanthrene over NiW-supported mesoporous HY zeolite catalyst depending on their mesoporosity. Mater Res Bull. 2017;96:149–154.
- Mondal P, Bhanja P, Khatun R, et al. Palladium nanoparticles embedded on mesoporous TiO2 material (Pd@ MTiO2) as an efficient heterogeneous catalyst for Suzuki-Coupling reactions in water medium. J Colloid Interface Sci. 2017;508:378–386.
- Huang SH, Liu CH, Yang CM. Efficient ligand-free Hiyama cross-coupling reaction catalyzed by functionalized SBA-15-supported Pd nanoparticles. Green Chem. 2014;16(5):2706–2712.
- Karimi B, Esfahani FK. Unexpected golden Ullmann reaction catalyzed by Au nanoparticles supported on periodic mesoporous organosilica (PMO). Chem Commun. 2011;47(37):10452–10454.
- Yang YS, Shen ZL, Loh TP. Zn/InCl3-mediated pinacol cross-coupling reactions of aldehydes with α, β-Unsaturated ketones in aqueous media. Org Lett. 2009;11(10):2213–2215.
- Silva AR, Carneiro L, Carvalho AP, et al. Asymmetric benzoylation of hydrobenzoin by copper (II) bis (oxazoline) anchored onto ordered mesoporous silicas and their carbon replicas. Catal Sci Technol. 2013;3(9):2415–2424.
- Sakthivel A, Komura K, Sugi Y. MCM-48 supported tungstophosphoric acid: an efficient catalyst for the esterification of long-chain fatty acids and alcohols in supercritical carbon dioxide. Ind Eng Chem Res. 2008;47(8):2538–2544.
- Pramanik M, Nandi M, Uyama H, et al. Organic–inorganic hybrid porous sulfonated zinc phosphonate material: efficient catalyst for biodiesel synthesis at room temperature. Green Chem. 2012;14(8):2273–2281.
- Bahrami K, Karami Z. Core/shell structured ZnO@SiO2-TTIP composite nanoparticles as an effective catalyst for the synthesis of 2-substituted benzimidazoles and benzothiazoles. J Exp Nanosci. 2018;13(1):272–283.
- Bahrami K, Bakhtiarian M. Mesoporous titania‐alumina mixed oxide: a heterogeneous nanocatalyst for the synthesis of 2‐substituted benzimidazoles, benzothiazoles and benzoxazoles. Chem Select. 2018;3(39):10875–10880.
- Rotstein BH, Zaretsky S, Rai V, et al. Small heterocycles in multicomponent reactions. Chem Rev. 2014;114(16):8323–8359.
- Zarganes‐Tzitzikas T, Chandgude AL, Dömling A. Multicomponent reactions, union of MCRs and beyond. Chem Rec. 2015;15:981–996.
- Biggs-Houck JE, Younai A, Shaw JT. Recent advances in multicomponent reactions for diversity-oriented synthesis. Curr Opin Chem Biol. 2010;14(3):371–382.
- Onouchi H, Miyagawa T, Furuko A, et al. Enantioselective esterification of prochiral phosphonate pendants of a polyphenylacetylene assisted by macromolecular helicity: storage of a dynamic macromolecular helicity memory. J Am Chem Soc. 2005;127(9):2960–2965.
- Surry DS, Buchwald SL. Biaryl phosphane ligands in palladium‐catalyzed amination. Angew Chem Int Ed. 2008;47(34):6338–6361.
- Li Y, Lu LQ, Das S, et al. Highly chemoselective metal-free reduction of phosphine oxides to phosphines. J Am Chem Soc. 2012;134(44):18325–18329.
- Peyman A, Budt KH, Spanig J, et al. C2-symmetric phosphinic acid inhibitors of HIV protease. Tetrahedron Lett. 1992;33(32):4549–4552.
- Kafarski P, Lejczak B. Phosphorus, biological activity of aminophosphonic acids. Sulfur Silicon Relat Elem. 1991;63(1–2):193–215.
- Allen MC, Fuhrer W, Tuck B, et al. Renin inhibitors. Synthesis of transition-state analog inhibitors containing phosphorus acid derivatives at the scissile bond. J Med Chem. 1989;32(7):1652–1661.
- Baylis EK, Campbell CD, Dingwall JG. 1-Aminoalkylphosphonous acids. Part 1. Isosteres of the protein amino acids. J Chem Soc, Perkin Trans 1. 1984;1:2845–2853.
- Maryanoff BE, Reitz AB. The Wittig olefination reaction and modifications involving phosphoryl-stabilized carbanions. Stereochemistry, mechanism, and selected synthetic aspects. Chem Rev. 1989;89(4):863–927.
- Enders D, Saint‐Dizier A, Lannou MI, et al. The Phospha‐Michael addition in organic synthesis. Eur J Org Chem. 2006;2006(1):29–49.
- Miller RC, Bradley JS, Hamilton LA. Disubstituted phosphine oxides. III. Addition to α, β-unsaturated nitriles and carbonyl compounds1. J Am Chem Soc. 1956;78(20):5299–5303.
- Jiang Z, Zhang Y, Ye W, et al. C Bond formation via direct and three-component conjugate addition catalyzed by 1,5,7-triazabicyclo [4.4.0] dec-5-ene (TBD. Tetrahedron Lett. 2007;48(1):51–54.
- Sobhani S, Rezazadeh S. HClO4-SiO2 as a novel and recyclable catalyst for the phospha-michael addition of phosphorous nucleophiles to α, β-unsaturated malonates. Synlett. 2010;2010(10):1485–1488.
- Sobhani S, Parizi ZP, Rezazadeh S. Phospha-Michael addition of phosphorus nucleophiles to α, β-unsaturated malonates using 3-aminopropylated silica gel as an efficient and recyclable catalyst. J Organomet Chem. 2011;696(3):813–817.
- Sobhani S, Zarifi F. Pyridine-grafted graphene oxide: a reusable acid–base bifunctional catalyst for the one-pot synthesis of β-phosphonomalonates via a cascade Knoevenagel–phospha Michael addition reaction in water. RSC Adv. 2015;5(117):96532–96538.
- Yu YQ, Xu DZ. A quaternary ammonium salt [H-dabco][AcO]: as a recyclable and highly efficient catalyst for the one-pot synthesis of β-phosphonomalonates. RSC Adv. 2015;5(37):28857–28863.
- Kalla RMN, Kim I. Tris (hydroxymethyl) aminomethane as an efficient organobase catalyst for the synthesis of β-phosphonomalonates. Tetrahedron Lett. 2017;58(5):410–414.
- Munoz E, Boldú JL, Andrade E, et al. Intrinsically formed trivalent titanium ions in sol–gel titania. J Am Ceram Soc. 2001;84:392–398.
- Zhao L, Gao L. Coating multi-walled carbon nanotubes with zinc sulfide. J Mater Chem. 2004;14(6):1001–1004.
- Wandre T, Gaikwad P, Tapase A, et al. Sol–gel synthesized TiO2–CeO2 nanocomposite: an efficient photocatalyst for degradation of methyl orange under sunlight. J Mater Sci Mater Electron. 2016;27:825–833.
- Li G, Zhang D, Jimmy CY. Thermally stable ordered mesoporous CeO2/TiO2 visible-light photocatalysts. Phys Chem Chem Phys. 2009;11(19):3775–3782.
- Liu C, Tang X, Mo C, et al. Characterization and activity of visible-light-driven TiO2 photocatalyst codoped with nitrogen and cerium. J Solid State Chem. 2008;181(4):913–919.
- Watanabe S, Ma X, Song C. Characterization of structural and surface properties of nanocrystalline TiO2−CeO2 mixed oxides by XRD, XPS, TPR, and TPD. J Phys Chem C. 2009;113(32):14249–14257.
- Guan B, Lin H, Zhu L, et al. Selective catalytic reduction of NOx with NH3 over Mn, Ce substitution Ti0.9V0.1O2−δ nanocomposites catalysts prepared by self-propagating high-temperature synthesis method. J Phys Chem C. 2011;115(26):12850–12863.
- Hoecke KV, Quik JT, Mankiewicz-Boczek J, et al. Fate and effects of CeO2 nanoparticles in aquatic ecotoxicity tests. Environ Sci Technol. 2009;43(12):4537–4546.
- Ma X, Wang Q, Rossi L, et al. Cerium oxide nanoparticles and bulk cerium oxide leading to different physiological and biochemical responses in Brassica rapa. Environ Sci Technol. 2016;50(13):6793–6802.
- Dutta G, Waghmare UV, Baidya T, et al. Origin of enhanced reducibility/oxygen storage capacity of Ce1-xTixO2 compared to CeO2 or TiO2. Chem Mater. 2006;18(14):3249–3256.
- Rynkowski J, Farbotko J, Touroude R, et al. Redox behaviour of ceria–titania mixed oxides. Appl Catal A. 2000;203(2):335–348.
- Yuan Q, Li LL, Lu SL, et al. Facile synthesis of Zr-based functional materials with highly ordered mesoporous structures. J Phys Chem C. 2009;113(10):4117–4124.
- Liu J, Li X, Zhao Q, et al. The selective catalytic reduction of NO with propene over Cu-supported Ti–Ce mixed oxide catalysts: promotional effect of ceria. J Mol Catal A Chem. 2013;378:115–123.
- Pérez-Hernández R, Mendoza-Anaya D, Fernández M, et al. Synthesis of mixed ZrO2–TiO2 oxides by sol–gel: microstructural characterization and infrared spectroscopy studies of NOx. J Mol Catal A Chem. 2008;281(1–2):200–206.
- Romero-Galarza A, Dahlberg KA, Chen X, et al. Crystalline structure refinements and properties of Ni/TiO2 and Ni/TiO2-Ce catalysts and application to catalytic reaction of “CO+. Appl Catal A. 2014;478:21–29.
- Henrich VE, Dresselhaus G, Zeiger H. Observation of two-dimensional phases associated with defect states on the surface of TiO2. Phys Rev Lett. 1976;36(22):1335–1339.
- Vannice MA. An analysis of the Mars–van Krevelen rate expression. Catal Today. 2007;123(1–4):18–22.
- Metiu H, Chrétien S, Hu Z, et al. Chemistry of Lewis acid–base pairs on oxide surfaces. J Phys Chem C. 2012;116(19):10439–10450.
- Land P. Defect equilibria for extended point defects, with application to nonstoichiometric ceria. J Phys Chem Solids. 1973;34(11):1839–1845.
- Lawrence NJ, Brewer JR, Wang L, et al. Defect engineering in cubic cerium oxide nanostructures for catalytic oxidation. Nano Lett. 2011;11(7):2666–2671.
- Hou J, Li Y, Liu L, et al. Effect of giant oxygen vacancy defects on the catalytic oxidation of OMS-2 nanorods. J Mater Chem A. 2013;1(23):6736–6741.
- Jung J, Shin HJ, Kim Y, et al. Activation of ultrathin oxide films for chemical reaction by interface defects. J Am Chem Soc. 2011;133(16):6142–6145.
- Gong XQ, Selloni A. Role of steps in the reactivity of the anatase TiO2 (101) surface. J Catal. 2007;249(2):134–139.
- Sharghi H, Ebrahimpourmoghaddam S, Doroodmand MM. Iron-doped single walled carbon nanotubes as an efficient and reusable heterogeneous catalyst for the synthesis of organophosphorus compounds under solvent-free conditions. Tetrahedron. 2013;69(23):4708–4724.
- Yu YQ, Xu DZ. Polystyrene-supported DABCO as a highly efficient and recyclable heterogeneous catalyst for the one-pot synthesis of β-phosphonomalonates. Tetrahedron. 2015;71(19):2853–2857.
- Kour P, Kumar A, Rai VK. Aqueous microwave-assisted DMAP catalyzed synthesis of β-phosphonomalonates and 2-amino-4H-chromen-4-ylphosphonates via a domino Knoevenagel-phospha-Michael reaction. C R Chim. 2017;20(2):140–145.
- Sobhani S, Honarmand M. Silica-bonded 2-hydroxyethylammonium acetate as an efficient and recyclable catalyst for the synthesis of 2-amino-4H-chromen-4-yl phosphonates and β-phosphonomalonates. Catal Lett. 2013;143(5):476–485.
- Sobhani S, Pakdin-Parizi Z. Lanthanum (iii) triflate supported on nanomagnetic γ-Fe2O3: a new magnetically recyclable heterogeneous Lewis acid for the one-pot synthesis of β-phosphonomalonates. RSC Adv. 2014;4(25):13071–13077.
- Roy S, Banerjee B, Salam N, et al. Mesoporous titania‐iron (III) Oxide with nanoscale porosity and high catalytic activity for the synthesis of β‐amino alcohols and benzimidazole derivatives. ChemCatChem 2015;7(17):2689–2697.
- Kitiyanan A, Ngamsinlapasathian S, Pavasupree S, et al. The preparation and characterization of nanostructured TiO2–ZrO2 mixed oxide electrode for efficient dye-sensitized solar cells. J Solid State Chem. 2005;178(4):1044–1048.
- Kapoor M, Raj A, Matsumura Y. Methanol decomposition over palladium supported mesoporous CeO2–ZrO2 mixed oxides. Microporous Mesoporous Mater. 2001;44:565–572.
- Onsuratoom S, Chavadej S, Sreethawong T. Hydrogen production from water splitting under UV light irradiation over Ag-loaded mesoporous-assembled TiO2–ZrO2 mixed oxide nanocrystal photocatalysts. Int J Hydrog Energy. 2011;36(9):5246–5261.
- Shelkar R, Sarode S, Nagarkar J. Nano ceria catalyzed synthesis of substituted benzimidazole, benzothiazole, and benzoxazole in aqueous media. Tetrahedron Lett. 2013;54(51):6986–6990.