Abstract
The design of highly active photocatalysts for degradation of phenol can be particularly challenging for solar energy utilisation. Herein, a series of mesoporousLaCo1−xCexO3catalysts have been synthesised and studied for degradation of phenol under UV irradiation. Compared with LaCoO3-SG catalyst which is formed through sol-gel method, optimised LaCo1−xCexO3-NC prepared by nanocasting method showed remarkably higher activity for the degradation of phenol due to the higher specific surface area, and the order of degradation rates is LaCo0.85Ce0.15O3-NC > LaCoO3-NC > LaCoO3-SG. Moreover, Au/LaCo1−xCexO3 catalysts were synthesized via Au deposition–precipitation method, and the catalytic activity for photodegradation of phenol increased with the loading of Au on the support.
1. Introduction
As industry wastewater entering into rivers, lakes and seas, serious water pollution is caused by organic pollutants. In particular, phenol is one of most polluted materials in the water environment owing to its stable chemical structure as well as extensive usage in the chemical industry [Citation1]. Currently, photocatalytic technology with semiconductor as catalyst is known as an effective method to treat refractory organic compounds [Citation2]. Compared with simple oxides such as TiO2, Fe2O3, ZnO, WO3, perovskite-type composite oxides (ABO3) are the most promising photocatalysts in the photodegradation process due to their flexible design, high thermal and chemical stability.
The ABO3 composite oxides have a narrow band gap and can absorb visible light and promote electron transitions, so that ABO3 composite oxides show good photocatalytic activity for photodegradation of organic compounds. Especially, the doping of different ions at A or B sites further improve the catalytic performance of the composite oxides under visible light without changing perovskite-type structures, in which the B-site ion is always the active centre plays a decisive role in the catalytic reactions. Therefore, the selection of B-site elements is very important in the design or modification of ABO3 catalyst.
It was reported that the partial replacement of Co3+ ions in LaCoO3 by Fe3+, Cu2+, Mn3+ and Ce3+ ions could improve the catalytic performance for the degradation of phenol, resulting from the formation of oxygen vacancies [Citation3–6]. However, the rate of phenol degradation using perovskite oxides obtained by traditional methods as photocatalyst is very limited owing to low surface area of the catalyst and poor electron–hole separation rate in photochemistry process. To obtain perovskite-type oxides with high specific surface area, several preparation methods were put forward [Citation7–9]. Template route method including hard and soft template is used widely. For example, Lu [Citation10] synthesized three-dimensional ordered mesoporous LaCoO3 oxide with a specific surface of 96.7 m2.g−1 using cubic ordered mesoporous vinyl silica as template. Wang [Citation11] used KIT-6 as hard template to get mesoporous LaCoO3 oxide with specific surface area of 270 m2.g−1.
The perovskites with high specific surface area and mesoporous structure could provide a large number of active sites, accelerate the diffusion of the reactants and products, shorten the length of charge carrier diffusion and effectively inhibit the electron–hole recombination in semiconductors [Citation12]. Furthermore, the deposition of Au on the perovskite could enlarge the absorption range of sunlight through surface plasmon resonance, which inhibit the recombination of electron–hole pairs and improve the catalytic activity [Citation13–15].
Motivated by the above observations, we developed a novel and promising photocatalysts with mesoporous perovskite materials and doped rare earth ion (La–Co–Ce–O3) for the degradation of phenols. The ordered mesoporous La–Co–Ce–O3-NC catalyst through nanocasting method with high surface area showed an interesting performance in the degradation reaction rather than the catalyst through sol-gel method (La–Co–O3-SG). In order to implement the degradation of phenol under visible light irradiation, Au/LaCo1−xCexO3 photocatalyst was prepared, and the photocatalytic performance can be further improved.
2. Experimental
2.1. Catalyst preparation
The mesoporous LaCo1−xCexO3 (x = 0, 0.10, 0.15, 0.20) catalysts were prepared via nanocasting method by using SBA-15 as the template. In a typical synthesis procedure, stoichiometric amounts of lanthanum nitrate hexahydrate (La(NO3)3·6H2O), cobalt nitrate hexahydrate (Co(NO3)2·6H2O), cerium nitrate (Ce(NO3)3·6H2O) and citric acid were mixed in 15 mL ethanol containing 5 mL of deionised water. The molar ratio of the citric acid to total metal ions (La3+, Co2+ and Ce4+) was 2:1. A clear solution was obtained after stirring at room temperature for 30 min. Then, 1.0 g SBA-15 was added into the complex solution with stirring at 40 °C until it became viscous, then dried at 80 °C overnight. Subsequently, the obtained solid was calcined at 300 and 700 °C for 2 h (ramp 2 °C/min) in air, respectively. Finally, 2 M NaOH aqueous solution was used to remove silica template at room temperature for three times. After the solid was washed with deionised water and ethanol, and dried at 80 °C for 12 h, black powder was obtained, which was recorded as LaCo1−xCexO3-NC, respectively.
For comparison, LaCoO3 catalyst was prepared by sol-gel method, and was recorded as LaCoO3-SG. Except without template, this method is consistent with the above procedure.
About 3% Au/LaCo1−xCexO3-NC catalyst was prepared by deposition–precipitation method. The support was added to the HAuCl4·6H2O solution, followed by adding 0.1 M NaOH solution to adjust pH to 8, after continuous stirring for 2 h at 70 °C and subsequent aging for 6 h, the precipitate was filtered and washed with deionised water until being free from C1− ion, then the catalyst was obtained after calcination at 250 °C for 3 h.
2.2. Catalyst characterisation
The composition and crystal phase of samples were characterised by powder X-ray diffraction meter with Cu Ka radiation. Nitrogen adsorption–desorption measurements were determined using an ASAP 2020 M analyser utilising the Brunauer–Emmett–Teller (BET) model and Barrett–Joyner–Halenda (BJH) method for the calculation of specific surface areas and pore size distribution. Before measurement, the samples of 0.1–0.15 g were degassed in vacuum at 100 °C for 5 h. Hydrogen temperature programmed reduction (H2-TPR) measurements were carried out on a self-assembled apparatus with thermal conductivity detector (TCD) as detector under a flow rate of 50 mL·min−1 (5% H2/Ar). Approximately 50 mg of sample was pre-treated with Ar at 100 °C for 2 h, prior to running the H2-TPR experiments, and the temperature was raised from room temperature to 800 °C at a rate of 10 °C·min−1. X-ray photoelectron spectroscopy (XPS) also has been registered using ESCALAB 250xi Electron Spectrometer.
2.3. Photocatalytic evaluation
The photocatalytic degradation of phenol reactions were carried out using a 300 W Mercury lamp (without filter) as light source. Briefly, 10 mg of catalyst was suspended in 10 mL of aqueous solution containing 20 ppm phenol. The reaction temperature was kept at 20 °C. Before irradiation, stirred for 30 min to reach an adsorption/desorption equilibrium. Samples were withdrawn from the reactor after the reaction and filtered through a 0.22 µm water filter film. Analysis of the evolved phenol was performed by high-performance liquid chromatography produced by Shimadzu Company of Japan. The dark reaction results show that the prepared catalyst has better photocatalytic effect.
3. Results and discussion
3.1. X-ray diffraction
Wide-angle X-ray diffraction (XRD) pattern of the samples is shown in . LaCoO3-SG, LaCoO3-NC and LaCo0.85Ce0.15O3-NC exhibit characteristic diffraction peaks at 2θ = 22.9°, 32.7°, 40.3°, 47.6°, 58.3°, 68.6°, 69.5° and 78.2°, respectively, and similar to characteristic peaks of the XRD standard card PDF (JCPDS 48-0123), which indicate the pure rhombohedral perovskite phase is formed by nanocasting and sol-gel method. The intensity of characteristic diffraction peaks of LaCoO3-NC is lower than that of LaCoO3-SG, indicating that the crystallinity of LaCoO3-NC is weaker. La, Co and Ce oxide phases are not observed in LaCo1−xCexO3-NC, which indicates that Ce ions occupy the lattice position of Co ions [Citation16]. Au/LaCoO3-NC and Au/LaCo0.85Ce0.15O3-NC catalysts have obvious diffraction signal at 2θ = 38.3° attributable to Au (JCPDS PDF# 04-0784), suggesting that gold is loaded on the surface of the support.
3.2. N2 adsorption–desorption
As shown in , the N2 adsorption–desorption isotherms of LaCoO3-NC and LaCo0.85Ce0.15O3-NC correspond to type-IV isotherm with type-H2 hysteresis loop appeared in the relative pressure range of 0.45–0.80, which is the feature of mesoporous materials [Citation10]. shows that the pore size distributions of the two supports are narrow. The average pore diameters are 4 and 4.6 nm, respectively, and the specific surface area is 149 and 213 m2.g−1 (). The specific surface area of LaCoO3-SG is 5 m2.g−1. Furthermore, the pore size distribution and specific surface area of the 3% Au/LaCo0.85Ce0.15O3-NC were not change significantly, indicating the mesoporous structure remained.
Table 1. N2 adsorption–desorption measurement results of the samples.
3.3. Transmission electron microscopy
The structure and size of the catalysts were characterised by transmission electron microscopy (TEM). The average particle size of the nanoparticles of LaCoO3-SG is 61 nm according to the software of particle size calculation (). The result is corresponding to XRD analysis. It can be seen from that some mesoporous structures have been obtained. At the same time, there are some large particles on the surface of the samples, which indicates that the nanopore filling is not completely. Compared with the LaCoO3-SG, the mesoporous structure can provide good mass transfer performance and easy accessibility to the active sites in the catalytic process, and finally improve the catalytic efficiency. shows that the average particle sizes of Au are 7.81 and 6.05 nm, respectively.
3.4. Hydrogen temperature programmed reduction
Two main reduction steps were observed for the LaCoO3-SG (): the reduction peaks at 466 and 658 °C were the reduction of surface Co3+ to Co2+, and Co2+ to Co0 [Citation17], respectively. The reduction peaks of LaCoO3-NC and LaCo0.85Ce0.15O3 shifted to 450 and 448 °C (). After Au loading, the reduction peaks of Au appeared at 200–300 °C for 3% Au/LaCoO3 and 3% Au/LaCo0.85Ce0.15O3 corresponding to the literature [Citation14]. The reduction peak of catalysts shifted to low temperature, indicating that the catalytic efficiency were improved, which may result from nanocasting method facilitates loading of Au.
3.5. X-ray photoelectron spectroscopy
The surface element composition and metal oxidation state of the samples were characterised by XPS. The 3D spectra of lanthanum in the catalyst were shown in .The peaks of La3+at 856.0–852.5 eV and 837.4–833.7 eV appeared at La 3d3/2 and La 3d5/2 [Citation18], respectively. The BE = 779.8 and 797.9 eV ascribe to the surface Co3+ species, BE =780.0 and 781.9 eV ascribe to Co4+ [Citation19–20] (). In order to maintain the charge balance of the sample, some Ce4+ ions were formed in the process of substituting Ce3+ for Co3+ ions at B site. It is reported that two main peaks at BE = 822.0 and 866.0 eV (Ce 3d5/2 signal), BE = 899.5 and 903.6 eV (Ce 3d3/2 signal), and BE = 917.7 eV(Ce 3d3/2 signal), attributable to the surface Ce3+ and Ce4+ species [Citation21]. This conclusion is confirmed by Ce 3D spectra (), which is helpful to improve catalytic activity.
Figure 5. XPS patterns of samples. (a) LaCoO3-SG; (b) LaCoO3-NC; (c) 3%Au/LaCoO3-NC; (d) LaCo0.85Ce0.15O3; (e) 3%Au//LaCo0.85Ce0.15O3.
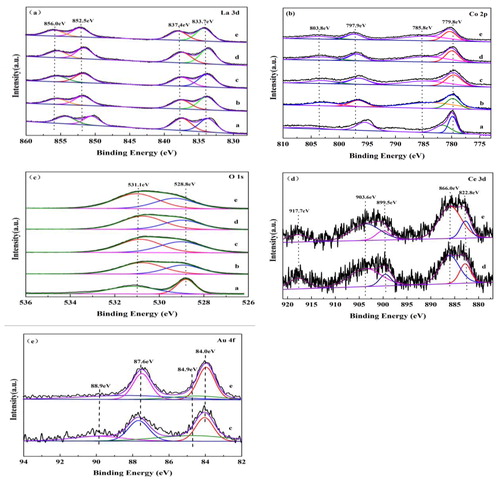
As shown in , the asymmetrical O 1 s spectrum of each sample could be decomposed into two components at BE = 532.2 and 530.6 eV, which can be assignable to the surface oxygen and lattice oxygen [Citation22], respectively. The surface oxygen content of LaCoO3-NC () and LaCo0.85Ce0.15O3-NC () is higher than LaCoO3-SG. Meanwhile, the catalytic activity of LaCo0.85Ce0.15O3-NC is higher than that of LaCoO3-NC, as the presence of Ce4+ increasing the oxygen vacancy of materials, facilitating the adsorption, activating and migration of oxygen sources, accelerating the formation of more surface oxygen [Citation9].
The Au 4f spectra of 3%Au/LaCoO3-NC and 3% Au/LaCo0.85Ce0.15O3-NC are shown in . and . Both Au0 and Auδ+(0 < δ < 3) species coexisted on the surface of the samples. The BE values correspond to 4f7/2 and 4f5/2 peaks at 84.0, 84.9, 87.6 and 88.9 eV, respectively [Citation23]. After loading Au onLaCoO3-NC and LaCo0.85Ce0.15O3-NCsupport, the surface oxygen OS content increased (), which might be attributed to the strong interaction between Au and oxygen species [Citation14].
Table 2. Au4f7/2 and O 1 s binding energies for catalysts.
Table 3. Composition of surface metal elements of catalysts determined by XPS.
3.6. Catalytic performance
The degradation rate of phenol is shown in . LaCoO3-NC demonstrated higher activity than LaCoO3-SG for the photodegradation of phenol under UV irradiation. The replacement of Co by Ce could improve the activity and reach the highest activity with 15% Ce doping. After loading Au on the LaCoO3-NC and LaCo0.85Ce0.15O3-NC support, the degradation rates of phenol were increased to 53.59 and 57.52%, respectively, indicating that gold had certain catalytic activity for phenol. The larger specific surface area of the LaCoO3-NC and LaCo0.85Ce0.15O3-NC means more opportunities to react with phenol, which may enhance the photocatalytic performance ().
Table 4. The activity of catalysts.
shows that gold modified LaCoO3-NC and LaCo0.85Ce0.15O3-NC,to some extent, have degradation effect on phenol under visible light. Degradation rate of phenol followed the order LaCo0.85Ce0.15O3-NC > LaCoO3-NC > LaCoO3-SG. This is consistent with the results of photodegradation of phenol under ultraviolet irradiation, indicating that Au modification is beneficial to the absorption of visible light.
Experimental conditions: The dosage of catalyst: 1 g·L−1, neutral pH, the concentration of phenol: 20 mg L−1, working volume of phenol: 10 mL, reaction time: 6 h, lamp source: 500 W Xenon lamp.
4. Conclusions
In summary, the ordered mesoporous catalysts with Ce-doped perovskite oxides at LaCoO3 B site were prepared successfully by nanocasting method for the first time. LaCo0.85Ce0.15O3-NC catalysts with abundant surface area achieved 49.74% photocatalytic activity for phenol degradation at 6 h of UV irradiation, which was better than other photocatalysts for phenol degradation. After loading Au on the LaCo0.85Ce0.15O3-NC support, the degradation rate of phenol (57.52%) increased. The BET and TEM characterisation results show that Au/LaCo0.85Ce0.15O3catalyst has a high specific surface area (213 m2.g−1) and small gold particles, which is conducive to improving the dispersion of active component Au. XPS analysis indicate that the presence of Ce4+ ions increased the oxygen vacancy of the material, which can be advantages to improve the catalytic performance of the catalyst. In addition, the presence of Auδ+ (0<δ<3) and more lattice oxygen on the surface of 3% Au/LaCo0.85Ce0.15O3 catalyst also contributed to the high activity of the catalyst.
Disclosure statement
No potential conflict of interest was reported by the authors.
Additional information
Funding
References
- Lang X, Zhao J, Chen X. Visible-light-induced photoredox catalysis of dye-sensitized titanium dioxide: selective aerobic oxidation of organic sulfides. Angew Chem Int Ed. 2016;55(15):4697–4700.
- Banerjee S, Pillai SC, Falaras P, et al. New insights into the mechanism of visible light photocatalysis. J Phys Chem Lett. 2014;5(15):2543–2554.
- Peng Q, Shan B, Wen Y, et al. Enhanced charge transport of LaFeO3 via transition metal (Mn, Co, Cu) doping for visible light photoelectrochemical water oxidation. Int J Hydrgen Energ. 2015;40(45):15423–15431.
- Miao J, Sunarso J, Duan X, et al. Nanostructured Co-Mn containing perovskites for degradation of pollutants: insight into the activity and stability. J Hazard Mater. 2018;349:177–185.
- Belessi VC, Ladavos AK, Pomonis PJ. Methane combustion on La–Sr–Ce–Fe–O mixed oxides: bifunctional synergistic action of SrFeO3−x and CeOxphases. Appl Catal B Environ. 2001;31(3):183–194.
- Luo J-Y, Meng M, Qian Y, et al. A mesoporous oxidation catalyst La–Co–Ce–O prepared by citric acid complexation and organic template decomposition method. Catal Lett. 2007;116(1–2):50–56.
- Levasseur B, Kaliaguine S. Methanol oxidation on LaBO3 (B = Co, Mn, Fe) perovskite-type catalysts prepared by reactive grinding. Appl Catal Gen. 2008;343(1/2):29–38.
- Royer S, Levasseur B, Alamdari H, et al. Mechanism of stearic acid oxidation over nanocrystalline La1-xAxBO3(A = Sr, Ce; B = Co, Mn): the role of oxygen mobility. Appl Catal B. 2008;80(1/2):51–61.
- Xue-Hui H, Xiao-Hui S, Peng-Ju N. Surface modification of SBA-15 and its effect on the structure and properties of mesoporous La0.8Sr0.2CoO3. Chin J Chem Phys. 2017;33(7):1462–1473.
- Wang Y, Ren J, Wang Y, et al. Nanocasted synthesis of mesoporous LaCoO3 perovskite with extremely high surface and excellent activity in methane combustion. J Phys Chem C. 2008;112(39):15293–15298.
- Wang Y, Cui X, Li Y, et al. A simple co-nanocasting method to synthesize high surface area mesoporous LaCoO3 oxides for CO and NO oxidations. Microporous Mesoporous Mater. 2013;176:8–15.
- Papadas I, Christodoulides JA, Kioseoglou G, et al. A high surface area ordered mesoporous BiFeO3 semiconductor with efficient water oxidation activity. J Mater Chem A. 2015;3(4):1587–1593.
- Zhang G, Miao H, Hu X, et al. A facile strategy to fabricate Au/TiO2 nanotubes photoelectrode with excellent photo electrocatalytic properties. Appl Surf Sci. 2017;391:345–352.
- Li X, Dai H, Deng J, et al. Au/3DOM LaCoO3: High-performance catalysts for the oxidation of carbon monoxide and toluene. Chem Eng J. 2013;228:965–975.
- Liu Y, Dai H, Deng J, et al. Au/3DOM La0.6Sr0.4MnO3: highly active nanocatalysts for the oxidation of carbon monoxide and toluene. J Catal. 2013;305:146–153.
- Souza Toniolo F, Magalhães RNSH, Perez CAC, et al. Structural investigation of LaCoO3 and LaCoCuO3 perovskite-type oxides and the effect of Cu on coke deposition in the partial oxidation of methane. Appl Catal B. 2012;117–118:156–166.
- Menggentuya J-l, Z. Effect of reparation conditions on Au/LaCoO3 Catalytic Performance for CO oxidation. J Mol Catal. 2009;23(5):443–447.
- Sato T, Watanabe R, Fukuhara C, et al. Effect of water density on heterogeneous catalytic water gas shift reaction in the presence of Ru/C, Pd/LaCoO3 and Fe3O4 in supercritical water. J Supercrit Fluids. 2015;97:211–216.
- Luo J, Zhou X, Ning X, et al. Utilization of LaCoO3 as an efficient co-catalyst to boost the visible light photocatalytic performance of g-C3N4. Sep Purif Technol. 2018;201:309–317.
- Sakthivel M, Ramaraj S, Chen S-M, et al. Synthesis of rose like structured LaCoO3 assisted functionalized carbon nanofiber nanocomposite for efficient electrochemical detection of anti-inflammatory drug 4-aminoantipyrine. Electrochim Acta. 2018;260:571–581.
- Yu H, Jiao Na Li Y, Pang J, et al. Au-CeO2 Janus-like nanoparticles fabricated by block copolymer templates and their catalytic activity in the degradation of methyl orange. Appl Surf Sci. 2018;427:771–778.
- Zhang Y, Beckers J, Bliek A. Surface properties and catalytic performance in CO oxidation of cerium substituted lanthanum–manganese oxides. Appl Catal A Gen. 2002;235(1/2):79–92.
- Meilin Li J, Zhaorigetu X, Yuenian S, et al. Activity and deactivation behavior of Au/LaMnO3 catalysts for CO oxidation. J Rare Earth. 2011;29(3):213–215.