Abstract
Hydrogel, with excellent hydrophilicity biocompatibility and stimuli responsive, has been widely used in biomedical and artificial organs fields. However, synthetic hydrogel usually displays poor gel strength, poor toughness and slow speed of absorbing water. Therefore, extensive exploratory research is still required to develop new composite materials for the application of bionic viscera. In this work, we constructed a new kind of composite hydrogel by a facile wet chemistry method to cross-link three kinds of polymer resin, polyvinyl alcohol, carboxymethyl cellulose, and carboxymethyl starch. The conductivity, moisture content and elastic modulus of hydrogel can be controlled by the dosage of potassium salt, sodium salt, water and glycerol. After molding and testing, the electrical conductivity, elastic modulus, and moisture content of the artificial liver and kidney organs were similar to those of the corresponding pig viscera, which is superior to most of the current research on hydrogels. This work provides a facile approach to synthesize highly efficient hydrogel, which accelerates the application of hydrogel in bionic organs.
1. Introduction
Hydrogel is a new kind of functional polymer material with three-dimensional network structure formed by moderate cross-linking [Citation1]. The polymer network of hydrogel shows solid-like properties, and the aqueous phase of hydrogel enables the carrier to quickly diffuse, which indicates that hydrogel displays the transport characteristics similar to liquid. Due to these favorable properties, many hydrogel materials are biocompatible and flexible [Citation2], making them ideal candidates for many biocompatible materials [Citation3, Citation4], drug delivery carrier [Citation5], energy saving [Citation6], medical wound dressings [Citation7–9] and tissue engineering [Citation10–12].
The hydrogel is insoluble in water, but it can swell significantly in water with excellent water absorption and water retention capacity. Based on this, Wang et al. proposed a vertical array of graphene hydrogel composite adsorption material (LiCl@rGO-SA) [Citation13]. They designed and constructed a new type of air water intake device that can realize rapid and continuous circulation. Through adsorption materials and water intake devices, the innovative breakthrough achieved solar-powered ultra-high air water withdrawal. In addition, conductive hydrogel has attracted extensive attention in the field of biology because of its conductivity and flexibility. For example, Jiao and his group prepared a novel transparent and highly ionic conductive hydrogel by using biological mineral calcium to regulate the polyacrylamide-sodium carboxymethyl cellulose (PAM-CMC) hydrogel crosslinked network structure [Citation14]. The hydrogel-based sensor shows highly sensitive to changes of strain and stress in a wide sensing range due to the high strength, high stretchability, high conductivity, and strong self-adhesion of hydrogel, which can be used to detect physiological signals of human movement and complex gesture language.
Macroscopically, the hydrogels, with the solid viscoelasticity, deform under the action of external force, and can return to its original state after the external force is removed. Microscopically, the water in hydrogels can freely diffuse in the three-dimensional network structure, and the diffusion coefficient is similar to that in aqueous solution, showing the properties of liquid [Citation15]. Therefore, hydrogel is a kind of special material between solid and liquid, which shows the properties both of solid and liquid. In addition, some of the hydrogels (intelligent response hydrogels) can remarkably respond to external stimuli such as temperature, pH, light, magnetic field and electric field through volume changes, making it a promising application in tissue engineering [Citation16], drug controlled release, sensor separation of materials [Citation17]. What’s more, hydrogels exhibit similar structures with many tissues in organisms, such as muscle, cartilage, cornea, and skin, and show excellent biocompatibility. Therefore, hydrogels, closer to living tissue than any other artificial material, are ideal materials for artificial replacement of organs [Citation18]. However, the poor mechanical strength and strain of hydrogels severely limit their potential applications [Citation19]. In recent years, toughened hydrogels have been prepared to improve the tensile and toughness of hydrogels by adding nanoparticles and double networks [Citation20–26], but the improvement of mechanical properties of toughened hydrogels is still too low. Therefore, it is necessary to further improve the mechanical performance and maintain a high electrical conductivity, realizing the hydrogels and biological tissue (such as muscle and skin) work together. PVA As a common polymer, PVA is widely used in biological materials because of its good biocompatibility, non-toxicity and excellent friction properties. The proximity of PVA molecular chains to each other can form crystallization and hydrogen bonding reactions enable them to physically cross-link to form hydrogels [Citation27–31]. However, pure PVA hydrogels are biologically inert hydrogels, which are difficult for cells to adhere to, so additives are required for regulation. As a polysaccharide with excellent physical and chemical properties, sustainably regenerated cellulose can be used in a wide variety of materials [Citation32–36]. Derivatives of cellulose are a series of compounds in which the hydroxyl groups of cellulose are partially or completely esterified or etherified [Citation37–39]. The graft-modified cellulose has different functional groups, which greatly expand the application of cellulose materials. In this paper, carboxymethyl cellulose is used as a regulator.
Herein, in order to promote the application of hydrogels in bionic organs, hydrogels were fabricated in this work by crosslinking and interpenetration of polyvinyl alcohol (PVA), carboxymethyl cellulose (CMC) and carboxymethyl starch (CMS), and adding a small amount of vapor silica as toughening agent. From the experimental results, we found that the conductivity of hydrogels can be effectively adjusted by adding and changing the dosage of the electrolytes such as sodium chloride and potassium chloride. What’s more, water and glycerin were used as plasticizer to control the water content and mechanical properties of hydrogels. Finally, the conductive hydrogels that meet the needs of biomimetic liver and kidney organs were successfully synthesized. This work provides an effective preparation method for controlled synthesis of hydrogels and promotes the practical application of hydrogels in bionic organs.
2. Synthesis method
2. 1. Synthesis of the hydrogel
Polyvinyl alcohol (PVA), carboxymethyl cellulose (CMC), carboxymethyl starch (CMS) and deionized water were added into a beaker at a mass ratio of 1.2:0.14:0.07:5.7 and stirring the solution for 30 min until uniform. Then ferric chloride was added to the mixture as a catalyst and a small amount of gaseous silica was added as the toughening agent for swelling. After the swelling, the mixed solution was heated to 85 °C for dissolution. PVA can tangle with the cellulose and CMS, with intermolecular and intramolecular hydrogen bond generating, thus forming the three-dimensional hydrogel. Then, the polymers were cross-linked and interpenetrated by stirring at 85 °C for 12 h. Next, it was cooled at room temperature to form gel. Finally, the formed gel was dried and dehydrated at 100 °C in the air-blast drying oven to form powder, which can be used according to different requirements of toughness and elasticity or stored as a solid.
3. Forming method
Water, sodium chloride, potassium chloride and glycerin were added to the mixed ligand formed by the above formula according to the requirements of different organs' toughness and elasticity, water content and conductivity (conductivity is adjusted by sodium chloride and potassium chloride aqueous solution, glycerin as plasticizer), and stirring for 30 min until evenly dispersed. Then, the color was adjusted by natural pigment, and the solution was injected into the silastic mold. Finally, the mold was placed in a thermostat at −20 °C for low temperature cross-linking for 12 h, and opening the mold and thawing the hydrogel at room temperature for 2 h to obtain hydrogel products. Reagents and the related information are shown in Table S1. All reagents are purchased with the same purity and have not been purified.
3.1. Characterization of the hydrogel
Powder X-ray diffraction (XRD) data were measured on a Rigaku D/Max 2550 diffractometer with Cu Kα radiation (λ = 1.5418 Å). Scanning electron microscope (SEM) characterization was carried out by a HITACHI S-4800 at an accelerating voltage of 20 kV. X-ray photoelectron spectroscopy (XPS) data was obtained by a ThermoFisher-ESCALab250 with Al-Kα X-ray radiation (1486.6.6 eV). Transmission electron microscope (TEM), energy dispersive X-ray spectroscopy (EDX), high-resolution TEM (HRTEM) and high-angle annular dark-field scanning TEM (HAADF-STEM) images were performed by Tecnai G2 F20 S-Twin HRTEM with an accelerating voltage of 200 kV. Fourier transform infrared (FT-IR) spectroscopy was obtained on a Thermo Scientific Nicolet iS5. Static contact angle of the hydrogel powder material was recorded on a JC2000D1 contact Angle measuring instrument. The final powder material for characterization is obtained by drying the hydrogel after adjusting and molding. The materials used for different artificial organs are the same in composition, only the content of metal salts, glycerol, etc. is different. Therefore, the characterization and analysis of only one kind of powder material is needed to reveal the composition and structure of the material.
The moisture content of the sample was obtained by weighing the quality difference before and after drying. Physical dehydration, namely vacuum drying, was mainly used to dry samples for 12 h under the condition of 50 °C. The elasticity modulus test was carried out in the Dma device with the dimensions of 4 cm long, 1 cm wide and 0.3 cm thick of samples. Electrical conductivity was performed on a TH2683B conductivity tester at the frequency of 3 GHz. A small piece of the sample was put into a specific mold (height 50 mm, diameter 10 mm) at a pressure of 1 MPa to measure the resistance value. The conductivity of the samples, which is the reciprocal of the volume resistivity, is then calculated according to the mold size. Simples including the pig viscera and the artificial bionic organs are shown in .
4. Results and discussion
4.1. Morphology and composition of the hydrogel powder
Through the experiment of the above steps, a hydrogel with adjustable flexibility, water content and electrical conductivity has been successfully prepared. In order to determine the composition of the hydrogel and explore whether it meets the requirements of different bionic organs, the morphology and composition of the catalysts were characterized by SEM, TEM, XRD and XPS, and a series of tests was applied to characterize the hydrogel properties. In order to facilitate characterization and testing, the hydrogel was dried, dehydrated and ground into powder. In addition, the powder can be melted into water, stirred and cooled to form the hydrogel directly, which is easy to transport and store.
The surface morphology of the catalyst was observed by SEM and TEM to further understand the particle size, morphology and distribution of the catalyst. shows the surface topography of powder at different magnifications under SEM. As shown in these figures, the porous resin carbon material on the prepared hydrogel powder is uniformly loaded with small particles of uniform size. Through the measurement of the proportion, the particle size of the resin is between 20-300 μm, and the size of small particles on porous resin is about 200 nm, showing irregular shape. What’s more, the surface is distributed with mesoporous and macropores, which can ensure the water absorption of the composite resin. Besides, the macropores and the mesopores can also cover the small particles of conductive electrolyte well, so that it is evenly distributed and ensure its conductivity. To explore the hydrophilicity of the sample, we tested the static contact angle of the material with water. The contact angle between the material and water is 38.5° (), indicating that the material has good hydrophilicity, corresponding to the above analysis.
The XRD pattern obtained by X-ray diffraction instrument can be used for qualitative determination of the crystal plane. The X-ray diffraction pattern of conductive hydrogel powder is shown in , with the characteristic peak labeled. According to the XRD pattern, the larger carbon background peak at 2θ = 19.5° indicates that the material is loaded on the amorphous carbon material, which should be a dried hydrophobic resin. Meanwhile, the two diffraction peaks of the hydrogel powder at 2θ = 28.4° and 40.6° correspond to the (200) (220) crystal plane of KCl (JCPDS No. 41-1476), and the five diffraction peaks at 2θ = 25.6°, 30.4°, 34.4°, 39.1°, and 44.6° represent the (210), (111), (121) (-221) and (320) crystal planes of NaHCO3 (JCPDS No. 15-0700), respectively. XRD pattern shows that we have successfully prepared the composite superabsorbent hydrogel material with strong electrolyte KCl and NaHCO3, in which the addition of KCl and NaHCO3 can greatly promote the conductivity of the material. In addition, chemical composition and weight of hydrogel powder were tested by EDX, as shown in . Non-metallic components C and O show strong peak values, which proves that most of hydrogel powder is resin carbon material carrier, and metal components Na and K only account for a small part in the material, which can indirectly support the XRD analysis results. Besides, FT-IR spectrum was carried out to further explore the composition. As shown in , the peaks at 1650 cm−1, 3355 cm−1, 2924 cm−1, and 1373 cm−1 corresponds to the stretching vibrations of the carbonyl, the N-H bond of amino, the C-H bonds from methyl and methylene, respectively, which are derived from CMS and CMC. The strong peak of C-O bond appears at the wavelength of 1026 cm−1, corresponding to the ether group formed by the polymer crosslinking. The C-O bond appears at 578 cm−1 due to the carboxyl group in the hydrogel. Based on the above analysis, it can be concluded that the hydrogel powder consists of a few small granular KCl and NaHCO3 crystals loaded on the porous composite superabsorbent resin carbon material.
In order to further explore the elemental composition and electronic structure of the material, XPS test was conducted on hydrogel powder, as shown in . shows the full spectrum of XPS with some key elements annotated. According to the peak area and intensity, it can be clearly seen that the material is mainly composed of C and O, with relatively few elements such as Na, K and Cl. Meanwhile, according to the fine spectra of each element (), it can be concluded that Na [Citation40], K [Citation41] are in +1 valence and Cl is in −1 valence state [Citation42]. O mainly exists in C-O bonds, presumably corresponding to the composition of the resin material. In addition to the standard carbon represented by 284.8 eV, the binding energy peak of C represents the C-O bond in the resin material at 286.3 eV, and the binding energy of the C-bonded part in Na salt at 287.2 eV. Thus, XPS can further prove the XRD analysis results that Na, K, Cl formed KCl and NaHCO3 crystals supported on the resin material respectively.
Figure 4. a) XPS survey spectra of the hydrogel powder. b–f) High-resolution XPS spectra of C 1 s, Cl 1 s, K 2p, Na 1 s and O 1 s, respectively.
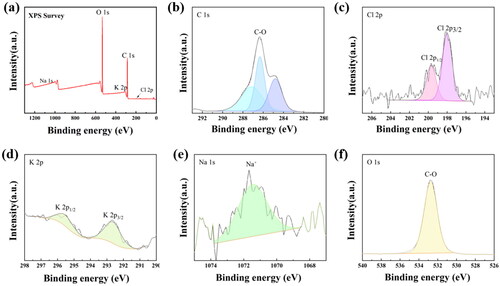
As shown in , the structure and morphology of hydrogel powder particles were observed. It could be clearly seen that ionic crystals with conductive ability were distributed near the resin carbon material from TME images. shows the electrolyte crystal (particles on the left) and the carbon material carrier (right). are high magnification microscope images of magnified in a red frame. It can be seen from that the particle size of electrolyte is about 100 nm and the measured lattices spacing are 0.239 nm and 0.237 nm respectively, corresponding to the (211) and (031) crystal planes of NaHCO3, respectively (), which laterally verifies the XRD characterization results. As shown in , the resin carbon material shows no lattice stripes, indicating it is amorphous carbon material. shows the HAADF-STEM image and corresponding element distribution of hydrogel powder particles. As shown in these figures, the distribution of elements well proves the above analysis results that the electrolyte particles are loaded on the resin carbon material.
5. Performance analysis of bionic liver and kidney organs
Through a series of analysis of the hydrogel powder, it can be concluded that the gel material is composed of composite porous absorbent resin material with sodium salt and sylvite supported. Theoretically, due to the load of the electrolyte material and the porous coating, the gel displays relatively stable conductivity. Thus, the conductivity, mechanical properties and water content of the hydrogel can be adjusted controllably by changing the contents of sodium chloride, water, and glycerin. In order to synthesize artificial liver and kidney hydrogels that meet the requirements of bionic organs, a series of tests were carried out to evaluate the hydrogel materials in terms of conductivity, elastic modulus, and water content.
shows the hydrogel of artificial liver and kidney organ formed in the mold. As supporting materials for tissues and organs, biomimetic organ materials need to exhibit the indistinguishable conductivity, water content, elastic modulus and other properties with corresponding organs to ensure good biocompatibility. The conductivity of hydrogel material was adjusted by adding electrolytes such as sodium chloride and potassium chloride. The specific test method and comparison range are shown in supporting information. As shown in , the electrical conductivity of the bionic liver and kidney was 0.0874 S m−1 and 0.089 S m−1, respectively, meeting the requirements of pig viscera.
Figure 6. a) Optical photograph, b) electrical conductivity, c) moisture content, d) elastic modulus of the bionic liver and kidney, respectively.
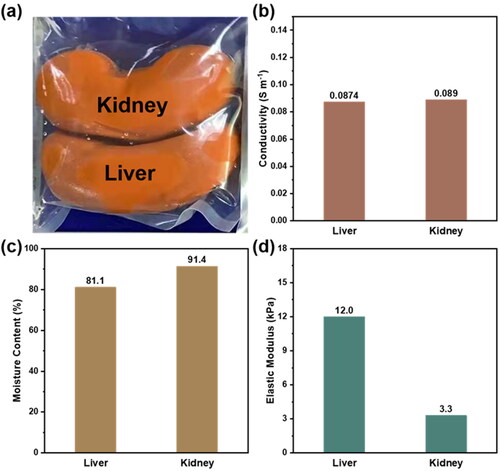
Due to the different structure and composition of tissues in the body, including differences in the proportion of protein, fat and other substances, the water content of each organ and the surrounding environment is various. However, only when the water content of the bionic organ is similar to that of the organ, can the water balance of the bionic organ material and its working environment be guaranteed, so as to ensure the structural stability of the material without damaging the working environment of the body. In this work, the water content of the hydrogel was obtained by comparing the quality difference before and after drying and dehydration by vacuum drying oven (supporting for details). As shown in , the measured moisture content of the liver and kidney of the bionic organ is 81.1% and 91.4% respectively, which also consistent with the data of corresponding pig viscera.
In addition, when hydrogel is used to prepare bionic viscera, it is necessary to show appropriate elastic modulus to ensure its structural stability as well as proper deformability. We prepared hydrogel materials suitable for different parts of liver and kidney by changing the dosage of glycerol as plasticizer. According to relevant standards, the elastic modulus of biomimetic liver and kidney hydrogels were 12.0 kPa and 3.3 kPa respectively by using Dma device for temperature changing test (), which are suitable for application17. What’s more, the artificial liver and kidney we synthesized are superior to most of the current hydrogel research for the artificial organs (Table S2).
6. Conclusions
In summary, we successfully fabricated adjustable composite polymer hydrogel materials for biomimetic liver and kidney organs through polymerization and simultaneous cross-linking method. According to a series of microstructure characterization of hydrogels powder, it can be concluded that the hydrogel powder consists of a few small granular KCl and NaHCO3 crystals loaded on porous composite superabsorbent hydrogel materials. Electrolytes, such as KCl and NaHCO3 crystal, effectively improve the conductivity of hydrogel, while porous composite resin materials ensure sufficient water absorption and stability. Finally, a series of test results, such as conductivity under high frequency, water content and elastic modulus, indicated that the synthetic hydrogel bionic organ can meet the needs of pig viscera. In this work, the electrical conductivity and elastic modulus of hydrogel were adjusted controllably, which provides a new idea for the optimization and application of hydrogel in bionic organs.
Supplemental Material
Download MS Word (701.8 KB)Disclosure statement
No potential conflict of interest was reported by the authors.
Data availability statement
The data that support the findings of this study are available from the corresponding author on reasonable request.
Additional information
Funding
References
- Liu X, Liu J, Lin S, et al. Hydrogel machines. Mater Today. 2020;36:102–124.
- Li TT, Xing MF, Gao B, et al. Multiscale synergistic toughened pluronic/PMEA/hydroxyapatite hydrogel laminated aramid soft composites: puncture resistance and self-healing properties. Composites Part B-Engineering. 2021;216:108856.
- Yin Chin S, Cheung Poh Y, Kohler AC, et al. Additive manufacturing of hydrogel-based materials for next-generation implantable medical devices. Sci Robot. 2017;2(2):eaah6451
- Xue X, Hu Y, Deng Y, et al. Recent advances in design of functional biocompatible hydrogels for bone tissue engineering. Adv Funct Mater. 2021;31(19):2009432.
- McFetridge ML, Del Borgo MP, Aguilar MI, et al. The use of hydrogels for cell-based treatment of chronic kidney disease. Clin Sci (Lond). 2018;132(17):1977–1994.
- Xu Z, Wang S, Hu X-Y, et al. Sunlight-induced photo-thermochromic supramolecular nanocomposite hydrogel film for energy-saving smart window. Sol RRL. 2018; 2(11):1800204.
- Guo B, Dong R, Liang Y, et al. Haemostatic materials for wound healing applications. Nat Rev Chem. 2021; (11):773–791.
- Kharaziha M, Baidya A, Annabi N. Rational design of immunomodulatory hydrogels for chronic wound healing. Adv Mater. 2021; 33(39):e2100176.
- Dong R, Guo B. Smart wound dressings for wound healing. Nano Today. 2021;41:101290.
- Hiraki HL, Nagao RJ, Himmelfarb J, et al. Fabricating a kidney cortex extracellular Matrix-Derived hydrogel. J Vis Exp. 2018; (140):e58314.
- Ye S, Boeter JWB, Mihajlovic M, et al. A chemically defined hydrogel for human liver organoid culture. Adv Funct Mater. 2020;30(48):2000893.
- Ye S, Boeter JWB, Penning LC, et al. Hydrogels for liver tissue engineering. Bioengineering (Basel). 2019;6(3):59.
- Xu J, Li T, Yan T, et al. Ultrahigh solar-driven atmospheric water production enabled by scalable rapid-cycling water harvester with vertically aligned nanocomposite sorbent. Energy Environ Sci. 2021;14(11):5979–5994.
- Bai J, Wang R, Wang X, et al. Biomineral calcium-ion-mediated conductive hydrogels with high stretchability and self-adhesiveness for sensitive iontronic sensors. Cell Rep Phys Sci. 2021; 2(11):100623.
- Yang C, Suo Z. Hydrogel ionotronics. Nat Rev Mater. 2018; 3(6):125–142.
- Li TT, Xing MF, Gao B, et al. Construction of synergistic toughening, self-healing puncture-resistant soft composites by using fabric-reinforced pluronic/PMEA hydrogel. Composites Part A-Applied Science and Manufacturing. 2021;145:106388.
- Correa S, Grosskopf AK, Lopez Hernandez H, et al. Translational applications of hydrogels. Chem Rev. 2021;121(18):11385–11457.
- Lei L, Danlian H, Yashi C, et al. Design of an amorphous and defect-rich CoMoOF layer as a pH-universal catalyst for the hydrogen evolution reaction. J Mater Chem A. 2021;9(13): 8730–8739.
- Han J, Wang S, Zhu S, et al. Electrospun core-shell nanofibrous membranes with nanocellulose-stabilized carbon nanotubes for use as high-performance flexible supercapacitor electrodes with enhanced water resistance, thermal stability, and mechanical toughness. ACS Appl Mater Interfaces. 2019;11(47):44624–44635.
- Raghuwanshi VS, Garnier G. Characterisation of hydrogels: linking the nano to the microscale. Adv Colloid Interface Sci. 2019; 274:102044.
- Jiao Y, Lu KY, Lu Y, et al. Highly viscoelastic, stretchable, conductive, and self-healing strain sensors based on cellulose nanofiber-reinforced polyacrylic acid hydrogel. Cellulose. 2021; 28(7):4295–4311.
- Jiao Y, Lu Y, Lu K, et al. Highly stretchable and self-healing cellulose nanofiber-mediated conductive hydrogel towards strain sensing application. J Colloid Interface Sci. 2021;597:171–181.
- Lu Y, Han JQ, Ding QQ, et al. TEMPO-oxidized cellulose nanofibers/polyacrylamide hybrid hydrogel with intrinsic self-recovery and shape memory properties. Cellulose. 2021;28(3):1469–1488.
- Lu Y, Yue Y, Ding Q, et al. Self-recovery, fatigue-resistant, and multifunctional sensor assembled by a nanocellulose/carbon nanotube nanocomplex-mediated hydrogel. ACS Appl Mater Interfaces. 2021;13(42):50281–50297.
- Zheng C, Lu K, Lu Y, et al. A stretchable, self-healing conductive hydrogels based on nanocellulose supported graphene towards wearable monitoring of human motion. Carbohydr Polym. 2020;250:116905.
- Zhu S, Sun H, Lu Y, et al. Inherently conductive poly(dimethylsiloxane) elastomers synergistically mediated by nanocellulose/carbon nanotube nanohybrids toward highly sensitive, stretchable, and durable strain sensors. ACS Appl Mater Interfaces. 2021;13(49):59142–59153.
- Ding Q, Xu X, Yue Y, et al. Nanocellulose-mediated electroconductive self-healing hydrogels with high strength, plasticity, viscoelasticity, stretchability, and biocompatibility toward multifunctional applications. ACS Appl Mater Interfaces. 2018;10(33):27987–28002.
- Han JQ, Ding QQ, Mei CT, et al. An intrinsically self-healing and biocompatible electroconductive hydrogel based on nanostructured nanocellulose-polyaniline complexes embedded in a viscoelastic polymer network towards flexible conductors and electrodes. Electrochim Acta. 2019;318:660–672.
- Shan J, Ling T, Davey K, et al. Transition-metal-doped RuIr bifunctional nanocrystals for overall water splitting in acidic environments. Adv Mater. 2019;31(17):e1900510.
- Niu ZX, Cheng WL, Cao ML, et al. Recent advances in cellulose-based flexible triboelectric nanogenerators. Nano Energy. 2021;87:106175.
- Oyeoka HC, Ewulonu CM, Nwuzor IC, et al. Packaging and degradability properties of polyvinyl alcohol/gelatin nanocomposite films filled water hyacinth cellulose nanocrystals. J Biores Bioproducts. 2021;6(2):168–185.
- Chen L, Cao S, Huang L, et al. Development of bamboo cellulose preparation and its functionalization. J For Eng. 2021;6(04):1–13.
- Huang C, You C, Xiong R, et al. Research progress of natural polysaccharide in the application of biomedical materials. J For Eng. 2021;6(03):1–8.
- Ling Z, Lai C, Huang C, et al. Research progress in variations of cellulose supramolecular structures via biomass pretreatment. J For Eng. 2021;6(04):24–34.
- Wang J, Jia L, Xu Y, et al. Effect of acetic acid-sulfite two-step pretreatment on the adsorption of poplar lignin toward cellulase. J For Eng. 2021;6(05):111–119.
- Yu Y, Li Y, Lou Y, et al. Effect of lignin condensation on cellulose enzymatic hydrolysis during deep eutectic solvent fractionation of lignocellulose. J For Eng. 2021;6(06):101–108.
- Amin KNM, Hosseinmardi A, Martin DJ, et al. A mixed acid methodology to produce thermally stable cellulose nanocrystal at high yield using phosphoric acid. J Biores Bioproducts. 2022;7(2):99–108.
- Deeksha B, Sadanand V, Hariram N, et al. Preparation and properties of cellulose nanocomposite fabrics with in situ generated silver nanoparticles by bioreduction method. J Biores Bioproducts. 2021; 6(1):75–81.
- Yang X, Biswas SK, Han J, et al. Surface and interface engineering for nanocellulosic advanced materials. Adv Mater. 2021;33(28):e2002264.
- Darwiche A, Bodenes L, Madec L, et al. Impact of the salts and solvents on the SEI formation in Sb/Na batteries: an XPS analysis. Electrochim Acta. 2016; 207:284–292.
- Cano A, Monroy I, Avila M, et al. Relevant electronic interactions related to the coordination chemistry of tetracyanometallates. An XPS study. New J Chem. 2019;43(46):18384–18393.
- Winiarski J, Tylus W, Szczygieł B. EIS and XPS investigations on the corrosion mechanism of ternary Zn–Co–Mo alloy coatings in NaCl solution. Appl Surf Sci. 2016;364:455–466.