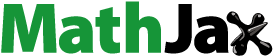
Abstract
Green synthesis of silver nanoparticles (AgNPs) has attracted substantial interest and achievement due to their environmental friendliness, ease of manipulation, and potential for large-scale production. By using aqueous Camellia sinensis leaf extract, suspensions of AgNPs, which possess photocatalytic and biological activity, were successfully synthesized in this study. The reduction of silver ions to AgNPs was visually inspected via the colour of the reaction mixture, which was altered from pale yellow to reddish brown. The methods of characterization of the as-synthesized AgNPs were performed using Ultraviolet-Visible spectroscopy (UV-Vis), dynamic light scattering technique (DLS), and X-ray diffraction analysis (XRD). The surface plasmon resonance bands of AgNPs at 420-430 nm in UV-Vis spectra indicated the presence of silver particles at the nanoscale. The size of AgNPs was determined by DLS, ranging from 25 nm to 40 nm. XRD analysis showed that AgNPs were highly pure and crystalline. The antibacterial activity of AgNPs against Gram-positive and Gram-negative bacterial strains, including Staphylococcus aureus and Escherichia coli, respectively, was demonstrated by the disc diffusion method. The examination of the photocatalytic activity of AgNPs was conducted through methylene blue (MB) degrading experiments, and the MB degrading performance was approximately 95% within 72 h under sunlight.
1. Introduction
According to a report generated from a market study, “Silver nanoparticles are emerging as one of the fastest growing product categories in the nanotechnology industry” [Citation1]. By engineering metallic silver into ultrafine particles, which have one of three dimensions less than 100 nm, these particles are considered silver nanoparticles (AgNPs) [Citation2]. By virtue of their size and surface effect, AgNPs reveal exceptional physical, chemical, and biological properties. The “quantum size effect” is accountable to the size-dependent properties of AgNPs. In comparison with bulk metallic silver, clusters of atoms in AgNPs are not subjected to the average of all quantum forces, thererby displaying idiosyncratic properties, such as catalytic and antimicrobial activity, cancer therapy, and sensing.
A plethora of approaches to synthesizing AgNPs can be classified into two basic pathways, namely top-down and bottom-up methods [Citation3]. Depending on the nature of synthetic processes, synthesis techniques can be categorized into physical, chemical, and biological methods. In reality, most of the physical synthetic methods have been unequivocally based on the top-down concept, which utilizes physical agents to “break off” bulk material into nanoparticles, such as mechanical milling, arc discharge, laser ablation, thermal evaporation/condensation, etc [Citation3, Citation4]. Meanwhile, most of the bottom-up synthetic routes involve chemical and biological methods. In respect of chemical synthesis, the conversion of precursors of ionic silver to Ag0 atoms is conducted via chemical reduction, then clusters of Ag0 atoms are formed at the nanoscale thanks to capping/stabilizing agents. Four types of chemical reactions that have been exploited to synthesize AgNPs include borohydride reduction [Citation5], citrate reduction (Turkevich method) [Citation6], and Tollens (silver-mirror) reaction [Citation7]. Due to the disadvantages of physical and chemical synthetic pathways such as high energy consumption, costliness, and the use of potentially toxic chemical reagents, green synthesis of AgNPs has been gaining momentum, which exploits naturally biological sources, including bacteria, fungi [Citation8], yeasts [Citation9], algae [Citation10], plants [Citation3], and natural biopolymers. Some superior advantages of biological synthetic routes consist of cost-effectiveness, environmental and biological compatibility, rapid synthesis rate, scalability, simplicity, and sustainability.
Among the aforementioned biological agents, plants have received enormous attention due to the availability of diverse biomolecules responsible for AgNP synthesis such as flavonoids [Citation11], terpenoids [Citation12], alkaloids, polyphenols, alcohol, phenolic acid, flavones, antioxidants, vitamins, polysaccharides. One of the early and impacted reports on the synthesis of AgNPs by using leaf extract derived from Geranium (Pelargonium graveolens) was published by Shankar et al. [Citation13]. Upon mixing geranium leaf extract with AgNO3 solution, the authors observed a quick formation of highly stable, crystalline AgNPs with particle sizes ranging from 16 to 40 nm. Also, the authors confirmed the rate of AgNPs synthesis using geranium leaf extract was faster than that mediated by a fungus (Fusarium oxysporum). Kalaichelvan, P.T. et al. used Acalypha indica leaf extract to optimize AgNP biosynthesis of AgNPs and evaluate their inhibitory efficacy against phytopathogenic fungi [Citation14]. In a study by Niraimathi, K. L. et al. Alternanthera sessilis (Linn.) leaf extract was used to synthesize AgNPs endowed with antimicrobial and antioxidant activities [Citation15]. SEM images revealed the AgNPs size of 30 nm and FTIR spectra suggested that the protein coating was responsible for stabilizing AgNPs. Interestingly, a venomous plant extract (Cleistanthus collinus) was of interest to Kanipandian, N. et al. in the green synthesis of AgNPs [Citation16]. The results AgNPs have a spherical shape, and a size ranging between 20 and 40 nm. Furthermore, as-synthesized AgPNs did not pose any poisonous threat to normal cell HBL100 and mice and exhibited a significant scavenging effect of free radicals, as well as anticancerous activity. Ahmed K.B.A. et al. first reported the sunlight-mediated synthesis of AgNPs using a redox protein system of ferredoxin-NADP+ and ferredoxin derived from spinach leaf extract [Citation17]. The as-synthesized AgNPs possessed catalytic activity, antibacterial activity against gram-positive and gram-negative bacteria, and Hg2+ ion detection. Roasted arabica coffee extract was an apt choice in a study by Dhand, V. et al. [Citation18] for the synthesis of AgNPs with spherical and ellipsoidal shapes. The resultant AgNPs displayed antibacterial activity as potently as the standard drug ampicillin did. Tulsi leaf extract [Citation19], Prosopis farcta seed aqueous extract [Citation20], Tropaeolum majus L. leaf extract [Citation21], Moringa oleifera flower extract [Citation22], Allium ampeloprasum leaf extract [Citation23], Tamarindus indica leaf extract [Citation24] have recently been selected as green sources for the synthesis of AgNPs with enhanced antimicrobial activities. In terms of plasmonic applications, the bioinspired nanoengineering of gold nanoparticles (AuNPs), AgNPs, and heterometallic (AgAu) nanohybrids have been lately of utmost interest in fabricating fluorescence-based detectors. Nature-derived materials such as silkworm and sericin proteins were employed to synthesize AuNPs, AgNPs, and AgAu nanohybrids with striking and tunable optoelectronic properties that can be applied to mobile phone-aided attomolar sensing of zinc ions and mefenamic acid in environmental and biological samples [Citation25, Citation26]. Particularly, the importance of incorporating the principles of green chemistry and nanotechnology into synthetic approaches of nanomaterials has been highlighted, thereby eradicating the toxic chemicals in the development of nanomaterials and promoting the use of renewable raw materials [Citation27].
Camellia sinensis leaf aqueous extract has been of interest in many reports relevant to the synthesis of metallic nanoparticles [Citation28, Citation29]. With an ample amount of catechins, which is a subgroup of polyphenols belonging to the flavonoids class, tea leaf extracts have become an efficient natural source of reducing and stabilizing agents. The mixture of catechins, including (-)-Epicatechin (EC), (-)-Epicatechin gallate (ECG), (-)-Epigallocatechin (EGC), and (-)-Epigallocatechin gallate (EGCG), is involved in the synthesis of AgNPs and imparts the biocompatibility [Citation30], antibacterial activity [Citation31] to resultant AgNPs. Tea has been one of the most widely consumed beverages throughout the world. There are four main types of tea produced from the Camellia sinensis plant: white, green, Oolong, and black tea [Citation32]. The classification is based on the Camellia sinensis leaf processing methods. Of these types of tea, green tea has been found to contain a large number of catechins, which are linked to its antioxidant effects and health-beneficial properties [Citation33]. Nevertheless, none of the studies has been focused on dyes treatment in wastewater using green tea extract synthesized AgNPs until now, given that numerous publications related to nature-derived AgNPs have investigated their application in eliminating dyes in water bodies [Citation34]. This study attempts to bridge this gap. One of the popular contaminants in water sources is dyes, which have been commonly used in food, pharmaceutical, cosmetics, paper, textile, plastics, ink, and paint industries. It has been demonstrated that plant-mediated AgNPs can eliminate dyes in water through adsorption, photocatalytic degradation, or synergistic effect of both [Citation35–39]. Recently, the multifunctional property of green-synthesized nanomaterials has stimulated novel discoveries, creating AgNPs-based nanohybrid with versatile properties and applications such as biosensing, dye degradation [Citation40], bactericidal, and anticancer activities [Citation41].
The dual functionality of plant-inspired AgNPs for antibacterial and photocatalytic properties has garnered considerable attention [Citation42–44] thanks to their nano-size, spherical shape, high dispersion, and colloidal stability [Citation45, Citation46]. In light of the previous studies associated with tea extract-derived AgNPs and their antimicrobial evaluation, this study was focused on the optimization of the synthesis of AgNPs using the aqueous extract of green tea leaf in Vietnam, the examination of the antibacterial activity against bacterial strains, encompassing gram-negative Escherichia coli (E. coli) and gram-positive Staphylococcus aureus (S. aureus), and the photocatalytic degradation of methylene blue of as-synthesized AgNPs. Furthermore, to the best of our knowledge, tea extract-synthesized AgNPs were first employed in dye treatment in this study.
2. Materials and methods
2.1. Materials
Dried green tea leaf product was purchased from Dai Nguyen Tea Company, Vietnam. Silver nitrate (AgNO3) was procured from China. Analytical grade NaOH and HCl were used as procured.
2.2. Methods
2.2.1. Preparation of tea leaf extract
3 g of dried green tea leaf product was brewed in 150 mL of double distilled water at 80 °C for 5 mins. After that, the tea leaf extract was filtered twice until there is no residue in the extract.
2.2.2. Synthesis of tea leaf extract-mediated AgNPs
The preparation of an aqueous solution of AgNO3 at the concentration of 2.5 mmol/L was conducted, then 10 mL of the as-prepared tea leaf extract was added to 10 mL of the 2.5 mmol/L AgNO3 solution. While agitated, the pH of the mixture was adjusted to a predetermined pH range. The colour change of the mixture from pale yellow to orangish brown is indicative of the formation of AgNPs. The effect of the pH of the mixture on the synthesis of AgNPs was investigated in the range from 7 to 12. Six levels of concentration (1 mM, 3 mM, 5 mM, 7 mM, 9 mM, 12 mM) were selected for the synthesis of AgNPs. The reaction time was varied from 5 to 120 mins.
2.2.3. Characterization of tea leaf extract-mediated AgNPs
2.2.3.1. UV-Vis spectroscopic analysis
The UV-Vis method can assist in monitoring the attributes of AgNPs because their optical properties based on the surface resonance phenomenon depend on their shape, size, and degree of polydispersity. Thanks to a Shimadzu UV-1800 UV-Vis spectrophotometer (Japan), the spectra of AgNPs suspensions were obtained.
2.2.3.2. Dynamic light scattering
The size distribution of AgNPs was measured using a dynamic light scattering analyzer (DLS, Jinan Winner Particle Instrument Stock Co., Ltd). The average hydrodynamic size, histogram of size distribution, and polydispersity index (PDI) were elucidated.
2.2.3.3. Transmission electron microscopy
The confirmation of the morphology of AgNPs (size and shape) was conducted using the transmission electron microscope (TEM). The TEM images of AgNPs were recorded on an instrument of JEOL JEM-Plus-1400, Tokyo, Japan.
2.2.3.4. X-ray diffraction analysis
The crystalline nature and purification were revealed by the X-ray Diffraction analytical method. The D8 Advance Eco diffraction system (Bruker) was employed to obtain the diffractogram of AgNPs. The measuring parameters included an operating voltage of 30 kV, a current of 10 mA, and X-ray radiation of CuKα with a wavelength of 1.54184 A°. The scanning was performed in the range between 5° to 80°. The average grain size of AgNPs can be inferred from the diffraction pattern using the Scherrer’s formula as follows:
Where Dp denotes average crystallite size (nm), K is the Scherrer constant (K = 0.94 for spherical crystallites with cubic symmetry), λ is X-ray wavelength (1.54184 A° for CuKα radiation), β represents FWHM (Full Width at Half Maximum, and θ is the Bragg angle.
2.2.4. Photocatalytic degradation of methylene blue using tea leaf extract mediated AgNPs
10-mL aliquots of an aqueous solution of MB at a concentration of 10 mg/L were prepared by diluting an appropriate amount of stock solution (1000 mg/L). Varying volumes of the tea leaf-mediated AgNPs suspension were added to the as-prepared MB solutions. A control sample was prepared without adding AgNPs. The elimination of MB using the tea leaf-mediated AgNPs was investigated as a function of pH conditions, the dose of AgNPs suspension, contact time, and sunlight exposure. The MB removal of AgNPs was evaluated by determining the remaining concentration of MB via absorbances at 664 nm and recording UV-Vis spectra using a spectrophotometer (Shimadzu UV-1800, Japan). The following equations were used to estimate the percentage of photocatalytic degradation (%PD) of MB:
or
where A0 and C0 denote the initial absorbance and concentration of the MB solution respectively and A and C represent the absorbance and concentration of the MB solution after the photocatalytic process respectively.
2.2.5. Antibacterial testing of AgNPs
The evaluation of the antibacterial activity of the tea leaf extract-mediated AgNPs was performed based on the growth inhibition of gram-positive S. aureus and gram-negative E. coli bacterial strains. Bacterial broth suspensions with suitable bacterial concentrations of about 1.5x108 CFU/mL for each bacteria were prepared. For the disc diffusion method, bacterial cultures, using the as-prepared broth, were uniformly spread over the Petri dishes of tryptic soy agar. Sterile paper circular discs freshly soaked in the AgNPs suspension and control solutions were put on the surface of agar plates. After the as-prepared plates were incubated at 37 °C for 24 h, the inhibition zones (ZoI), which result from the bacterial growth inhibition induced by antibacterial samples, were recorded.
Furthermore, the inhibition zones were analyzed according to the protocol of Serrano-Aroca et al. [Citation47], in which the diameters of the inhibition zones (diz) and sample discs (d) are measured respectively and the normalized width (nw) of each of the zones of inhibition is calculated as follows:
3. Results and discussions
3.1. Visual observation of AgNPs formation and UV-Vis spectral examination
It has been confirmed that the plant extract derived formation of AgNPs can impart yellowish brown or reddish brown to the resulting solutions [Citation48, Citation49]. After the addition of tea leaf extract to the aqueous solution of AgNO3 at appropriate pH levels, the colour of the reaction mixture changed from colourless to orangish brown (), which is in accord with previous studies [Citation50, Citation51]. Since AgNPs can display the localized surface plasmon resonance (SPR) band, the UV-Vis spectra could be employed to detect the formation of AgNPs and their size, shape, and concentration. The increased generation of AgNPs could be characterized by increased absorbance [Citation52, Citation53]. The degree of the sharpness of SPR peaks, which is assessed via the Full-Width Half Maximum (FWHM) value, provided the information pertinent to AgNPs size distribution (monodisperse, polydisperse, agglomerated) [Citation54]. Moreover, the size and stability of AgNPs could be deduced from the position of the SPR peak and its shift to either the longer or shorter wavelengths (red shift or blue shift) [Citation55, Citation56]. exemplified a spectrum of the tea leaf extract-mediated AgNPs, which showed a typical SPR band peaking at 412 nm. The noise observed between 500-550 nm may result from the agglomeration of as-synthesized AgNPs [Citation57]. The plausible mechanism of the formation of AgNPs synthesized from tea leaf extract has been suggested, in which tea catechins reduce Ag+ to Ag0, while catechins are oxidized to quinone [Citation51].
3.2. Influence of pH on the synthesis of tea leaf extract mediated AgNPs suspensions
The effect of pH is of crucial importance in the synthesis and manipulation of the size and shape of AgNPs, which has been demonstrated in relevant studies [Citation58]. As displayed in , UV-Vis spectra of AgNPs suspensions synthesized at different pH levels showed absorption peaks in the wavelength range of 400-420 nm, which correspond to the typical SPR bands of AgNPs. The trend of “red-shifting” of SPR peaks occurred as the pH of the mixture was increased from pH 7 to 12 and peak intensity decreased significantly at pH 12, signifying the aggregation of AgNPs. As the pH of the reaction mixture is more basic, the functional groups of flavonoids, terpenoids, and phenolic compounds present in the tea extract are ionized, increasing their reducing capacity; in turn, the speed of the reaction is improved [Citation59]. In addition, the basic pH levels possibly increase the conversion of Ag+ into AgOH [Citation36]. Therefore, pH conditions between 8.5 and 10 of reaction mixtures facilitated the synthesis of AgNPs.
Figure 3. UV-Vis results illustrative of the effect of pH conditions on the synthesis of AgNPs using tea leaf extract.
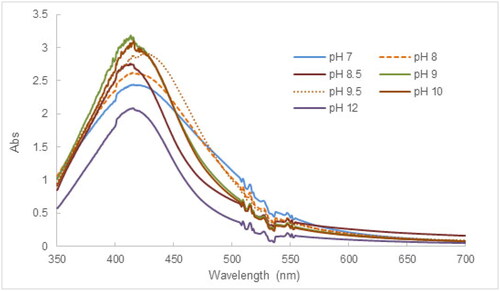
The results of particle size measurement by the DLS method revealed that the AgNPs size obtained the lowest value in the range of pH 8.5-10 (28-34 nm). At pH conditions beyond 10, the AgNPs size increased significantly (330 nm at pH 12). These results are consistent with UV-Vis spectra of AgNPs suspensions at different pH values. Furthermore, the spherical shape was primarily observed in the TEM image of AgNPs (Figure S1, SI) and their average particle size was approximately 41 nm, which is in agreement with the size values obtained by DLS. It was also found that biomolecules were attached to the surface of AgNPs, which was demonstrated by the presence of organic layers between AgNPs. Owing to this coating, agglomeration, and precipitation of AgNPs were prevented, which renders AgNPs dispersed in suspensions [Citation57].
3.3. Influence of reaction time on the synthesis of tea leaf extract mediated AgNPs suspensions
The formation and characteristics of resultant AgNPs could be impacted by the competition between nucleation and growth processes, which occur during the synthesis of AgNPs [Citation48]. In , as the reaction time was extended, the absorbance of the SPR peak increased gradually, corresponding to the much darker AgNPs suspensions visually. After 60 min, no significant change was noticed, which indicated the termination of the reaction. Prolonging the reaction time induces more biomolecules in the tea extract to reduce the Ag+; however, a very quick reaction is more likely to cause the aggregation of AgNPs [Citation46]. Therefore, the reaction time of 30 min at pH 8.5-10 was selected as the optimized parameter taking into account the completion of the reaction, and the limitation of aggregation.
3.4. Influence of AgNO3 concentration on the synthesis of tea leaf extract mediated AgNPs suspensions
The AgNPs suspensions displayed reddish-brown colours that gradually darkened at elevated AgNO3 concentrations (). When the AgNO3 concentrations were gradually elevated, the absorbances of SPR peaks of AgNPs suspensions in UV-Vis spectra were higher, signifying the increased content of AgNPs. In addition, an interesting observation was highlighted that the SPR peaks were shifted to the long wavelength region, indicating that the AgNPs size became gradually larger and more polydispersed (Figure S2, SI). This is attributed to the fact that the reduction rate of AgNO3 is accelerated at higher AgNO3 concentrations. Nevertheless, the agglomeration of AgNPs might happen as a result of weak Van der Waals attraction. The acquired results are consistent with those of previous reports [Citation50, Citation60].
3.5. Effect of tea leaf extract concentration on the AgNPs synthesis
The investigation into the effect of the concentration of tea leaf extract was carried out by mixing 10 mL of 5 mM AgNO3 in the tea leaf extract whose volume was varied from 6 to 10 mL. The pH level of 9 of reaction mixtures and the reaction time of 30 min were maintained respectively. The results in showed that the absorbance of the SPR peak decreased as the volume of tea extract increased, and the SPR peak was shifted to longer wavelengths (from 415 to 430 nm), which were consistent to the literature [Citation19]. This tendency could be attributed to the “valence effects” proposed in a previous study [Citation55] that there exists an optimal zone of the phytoconstituents concentration, in which silver ions would adhere efficiently to biomolecules, then they would be converted to silver atoms and AgNPs. Above the optimal concentration range, silver ions and atoms would be diluted in a large number of biomolecules, thereby causing the formation (conversion, nucleation) to be less advantageous.
3.6. Characterization of AgNPs using XRD analysis
XRD has been a key analytical method in many studies related to nanoparticles to confirm the crystal nature and assess the crystalline purity of biosynthesized AgNPs. Moreover, the AgNPs size can be estimated based on the Debye-Scherrer equation, in which the broadening of diffraction peaks was induced by the decrease in particle size. As displayed in , the XRD pattern exhibited typical diffraction peaks of silver at 38.3°, 44.1°, 64.6°, and 77.5°, which are associated with (111), (200), (220), and (311) respectively. According to the reflection rules, the crystal structure of AgNPs was found to be face-centered cubic (fcc). By comparison with the standard XRD patterns of Ag2O and AgCl, it was demonstrated that metallic silver was the only species in the tea leaf extract-mediated AgNPs. Based on the Scherrer’s formula, the average crystallite size was estimated to be 9.54 nm. The discrepancy in AgNPs size between the DLS measurement and the XRD estimation results from the fact that XRD determines the primary or pristine size of particles, while DLS measures the secondary or hydrodynamic size of particles, referring to the size of particles with surrounding layers that are moving in a liquid. Generally, the determination of particle size using the DLS technique can be overestimated and affected by medium factors such as polarity, refractive index, viscosity, and so on [Citation48].
3.7. Evaluation of antibacterial activity
It has been found that phytochemicals in tea possess antimicrobial activities due to the ability of catechin gallate to intercalate into bacterial membrane bilayers [Citation51]. Also, the antibacterial activities of AgNPs have been widely recognized in multiple studies pertinent to the green synthesis of AgNPs as opposed to concerns related to the toxicity of AgNPs. Cytotoxicity effects of green-synthesized AgNPs on different cell lines have been evaluated, showing that antiproliferative activities are potent against cancerous cells lines, but low against the normal fibroblast cell [Citation23] or the human epidermal keratinocytes cell lines [Citation51].
In this study, the antibacterial activity of the tea leaf extract-mediated AgNPs was examined against two bacterial strains, including Staphylococcus aureus (S. aureus) gram-positive infection-inducing pathogen, and Escherichia coli (E. coli) gram-negative food-poisoning pathogen. The results presented that the AgNPs suspension exhibited antibacterial activities against the tested bacterial strains. The AgNPs suspensions synthesized from different initial Ag+ concentrations possessed different antibacterial activities (). Particularly, the tea leaf extract-mediated AgNPs expressed higher antibacterial activities against S. aureus compared to E. coli (Figure S3, SI), which is in agreement with relevant studies [Citation61, Citation62]. Moreover, the elevated concentrations of AgNPs enhance the inhibition zones, emphasizing the antibacterial activities of AgNPs against both of the tested bacterial strains.
Figure 8. Widths of normalized inhibition zones of tea leaf extract, AgNPs suspensions synthesized respectively from 3, 5, and 9 mM AgNO3 solutions against E. coli and S. aureus.
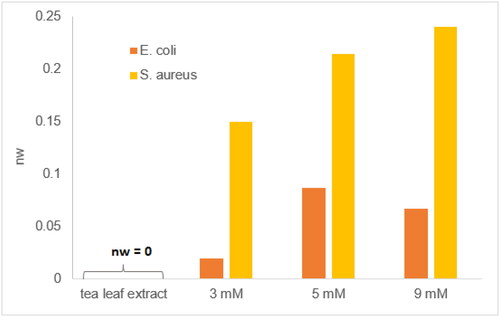
The causality of the antibacterial selectivity of AgNPs against gram-negative or gram-positive bacteria has not had an unambiguous clarification [Citation21, Citation63], which may depend on the charge’s attributes of AgNPs surface [Citation5], the surface chemistry of AgNPs [Citation51], and the composition of bacterial cell walls [Citation64]. Notwithstanding a huge effort to investigate the antimicrobial activity of plant-mediated AgNPs that many scientific publications have devoted, the accurate mechanism has not been comprehensively explored yet. There are two acceptable mechanisms: (i) the attachment of AgNPs on the cell wall of bacteria, the penetration of AgNPs through the cell walls, and the damage to intracellular biomolecules and structures; (ii) the liberation of Ag+ from AgNPs, then Ag+ ions interact with sulfhydryl groups of proteins and enzymes responsible for the ATP producing process, then deactivate them [Citation23]. In addition, AgNPs and Ag+ ions can induce the generation of reactive oxygen species (ROS), which in turn can disrupt cellular functions such as the respiratory process and cell division [Citation65].
3.8. Photocatalytic degradation of Methylene blue using tea leaf extract synthesized AgNPs
Photocatalytic degradation of MB using plant-mediated AgNPs is based on the interactions between valence electrons and light radiation through the Surface Plasmon Resonance effect, leading to electron-hole generation, then the photoexcited electrons reduce MB [Citation34]. Also, photoinduced electrons can be captured by other species in aqueous solution such as oxygen (O2), and hydroxyl (OH-) to produce free radicals (O2· and OH·), which ultimately convert MB into non-toxic substances (CO2 and H2O) [Citation38, Citation39]. Additionally, the catalytic degradation of dyes using AgNPs could be perceived as an “electron shuttling” process [Citation66], wherein AgNPs play a role of an electron transferring facilitator, widely considered as “the relay effect” [Citation67]. Thermodynamically, the catalytic degradation of dyes via reduction using NaBH4 or electron donors on the AgNPs surface (capping agents) is favorable, but the process is not kinetically advantageous. In the presence of AgNPs, the degradation of dyes is accelerated due to the intermediate redox potential of AgNPs and their small size, which catalyze the reduction reaction [Citation42, Citation68].
3.8.1. Effect of pH on MB degradation
As can be demonstrated in , pH significantly affected the degradation of MB using AgNPs synthesized from the tea leaf extract. The higher pH level of the reaction mixture resulted in better efficacy of the MB degrading process. In acidic pH conditions between 2.5 and 6, the photocatalytic degradation of MB was less favorable with the percentage of photocatalytic degradation ranging from 9.8 − 48.5%. On the contrary, the %PD increased rapidly in basic pH conditions, which reached 69.5% at the pH of 7.5 and leveled off at 83.4% above the pH of 8.5. According to the literature, AgNPs derived from tea leaf have been reported to have a negatively charged surface at slightly basic pH conditions [Citation51], whereas MB is a water-soluble cationic dye so MB can be efficiently adsorbed on the surface of AgNPs via electrostatic attraction at elevated pHs, which assists in the photocatalytic degradation. Furthermore, the basic pHs are conducive to the production of free radicals, especially the formation of hydroxyl radicals, which act as a strong oxidant to degrade effectively MB.
3.8.2. Effect of AgNPs dose on MB degradation
The influence of AgNPs dose on the performance of the MB photocatalytic degradation was investigated by varying the volume of the 2.5 mM AgNPs suspension in the mixture of 10 mg/L MB and AgNPs. The results in showed that the typical absorbance at 664 nm became higher at elevated amounts of the 2.5 mM AgNPs suspension, which demonstrated that the increasing amounts of AgNPs in the reaction mixture decreased the effectiveness of MB degradation. At sufficient amounts of AgNPs, the high dispersion and availability of active sites of AgNPs guarantee the performance of the MB photocatalytic degradation. In contrast, an excess of AgNPs in the reaction medium results in the agglomeration of AgNPs in contact with MB, thereby reducing the photocatalytic degradation [Citation38]. According to our results, the volume of the 2.5 mM AgNPs suspension should not exceed 200 μL.
3.8.3. Effect of contact time on MB degradation
In this experiment, the photocatalytic degradation of MB using the tea leaf extract-mediated AgNPs was investigated as a function of the contact time. The UV-Vis spectra of the reaction mixture at different time intervals exhibited two characteristics which were a decrease in absorbance at 664 nm, and a decline in absorbance at 425.5 nm. These findings could result from the synergy between photocatalytic activity and adsorbing ability of AgNPs [Citation34]. The %PD rose to 81.6% after 24 h and slightly increased to 85.3% after 72 h (), which accords with the relevant studies [Citation35, Citation69] ().
Figure 11. UV-Vis spectra illustrative of the effect of the contact time on the MB degradation (initial MB concentration: 10 mg/L, contact time: 24 h, pH: 8.5).
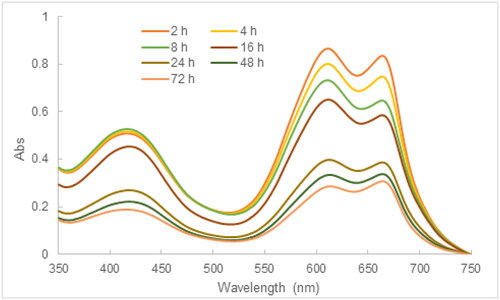
Table 1. Applicative performance of the tea-leaf extract synthesized AgNPs in this study and some recently reported biosynthesized AgNPs.
3.8.4. Effect of sunlight exposure on MB degradation
Numerous publications related to the photocatalytic activity of plant extract synthesized AgNPs have performed the photocatalytic degradation of dyes employing sunlight irradiation [Citation35, Citation37, Citation39, Citation70]. In this experiment, the effect of sunlight exposure was evaluated by mixing together 200 μL of the 2.5 AgNPs suspension and 10 mL of 10 mg/L MB at the pH of 8.5. The reaction suspension was exposed to the sunlight from sunrise to sunset within 72 h. The improvement in the MB photocatalytic degradation was observed in , wherein displayed a significant decline in the absorbance at 664 nm corresponding to the monomer MB and the absorbance at 617 nm assigned to dimer (MB)2 [Citation71]. The %PD was found to be 95% after 72 h of solar irradiation. Due to sunlight exposure, the photons interact with AgNPs, and the electrons are excited from the valence band to the conduction band, creating electron-hole pairs. The photoinduced electrons are captured by atmospheric oxygen to form oxygen anion radicals, while photogenerated holes are provided with electrons from hydroxyl anions to generate hydroxyl radicals. The radicals could decompose MB into carbon dioxide, water, and simpler organic molecules or ions [Citation72].
4. Conclusion
In this study, the effects of important parameters such as the pH condition, contact time, the AgNO3 concentration, and the tea leaf extract concentration on the tea leaf extract-mediated synthesis of AgNPs have been demonstrated. Methods of characterization were used, including UV-Vis spectroscopy, DLS, and XRD, which assisted in the confirmation of the success of AgNPs synthesis using the tea leaf extract. The SPR bands occurred between 420-430 nm in UV-Vis spectra of the AgNPs suspensions synthesized using the optimized conditions such as the pH of 8.5-9, the AgNO3/tea extract volume ratio of 1:1, and the reaction time of 30 mins. The average AgNPs size determined by DLS was in the range of 28-34 nm. The XRD spectrum of as-synthesized AgNPs unequivocally demonstrated the pure crystallinity of the tea leaf extract-mediated AgNPs. The significant antibacterial activity of the tea leaf extract mediated AgNPs against the Gram-positive S. aureus, and Gram-negative E. coli bacterial strains assisted in their promising applications in the pharmaceutical industry, food technology, cosmetics, and agriculture. In terms of the environmental application, the tea leaf extract-mediated AgNPs showed a remarkable photocatalytic degradation, recommending their applicable potential for dye treatment in wastewater.
CRediT authorship contribution statement
Chau Thi Bich Le: Investigation, Data curation, Formal Analysis, Visualization, Writing - original draft. Cuong Minh Pham: Investigation, Data curation, Formal Analysis, Visualization. Trung Huu Bui: Methodology, Validation, Formal Analysis. Quoc-An Trieu: Conceptualization, Methodology, Investigation, Data curation, Resources, Writing - review & editing, Supervision.
Supplemental Material
Download MS Word (749 KB)Disclosure statement
No potential conflict of interest was reported by the author(s).
Data availability statement
The authors confirm that the data supporting the findings of this study are available within the article and its supplementary materials. These data are available from the corresponding author upon request.
Additional information
Funding
References
- Chen X, Schluesener HJ. Nanosilver: a nanoproduct in medical application. Toxicol Lett. 2008;176(1):1–18. doi: 10.1016/j.toxlet.2007.10.004.
- Potočnik J. Commision Recommendation of 18 October 2011 on the definition of nanomaterial. The European Commission; 2011;38–40.
- Rana A, Yadav K, Jagadevan S. A comprehensive review on green synthesis of nature-inspired metal nanoparticles: mechanism, application and toxicity. J Clean Prod. 2020;272:122880. doi: 10.1016/j.jclepro.2020.122880.
- Jadoun S, Arif R, Jangid NK, et al. Green synthesis of nanoparticles using plant extracts: a review. Environ Chem Lett. 2020;19(1):355–374. doi:10.1007/s10311-020-01074-x.
- Abbaszadegan A, Ghahramani Y, Gholami A, et al. The effect of charge at the surface of silver nanoparticles on antimicrobial activity against gram-positive and gram-negative bacteria: a preliminary study. J Nanomater. 2015;2015:1–8. doi: 10.1155/2015/720654.
- Turkevich J, Stevenson PC, Hillier J. A study of the nucleation and growth processes in the synthesis of colloidal gold [10..1039/DF9511100055]. Discuss Faraday Soc. 1951;11:55–75. doi: 10.1039/df9511100055.
- Sharma VK, Yngard RA, Lin Y. Silver nanoparticles: green synthesis and their antimicrobial activities. Adv Colloid Interface Sci. 2009;145(1–2):83–96. doi: 10.1016/j.cis.2008.09.002.
- Jain N, Bhargava A, Majumdar S, et al. Extracellular biosynthesis and characterization of silver nanoparticles using Aspergillus flavusNJP08: a mechanism perspective [10..1039/C0NR00656D]. Nanoscale. 2011;3(2):635–641. doi: 10.1039/c0nr00656d.
- Waghmare SR, Mulla MN, Marathe SR, et al. Ecofriendly production of silver nanoparticles using Candida utilis and its mechanistic action against pathogenic microorganisms. 3 Biotech. 2015;5(1):33–38. doi: 10.1007/s13205-014-0196-y.
- Kathiraven T, Sundaramanickam A, Shanmugam N, et al. Green synthesis of silver nanoparticles using marine algae caulerpa racemosa and their antibacterial activity against some human pathogens. Appl Nanosci. 2015;5(4):499–504. doi: 10.1007/s13204-014-0341-2.
- Bose D, Chatterjee S. Biogenic synthesis of silver nanoparticles using guava (Psidium guajava) leaf extract and its antibacterial activity against Pseudomonas aeruginosa. Appl Nanosci. 2016;6(6):895–901. doi: 10.1007/s13204-015-0496-5.
- Mashwani Z-U-R, Khan MA, Khan T, et al. Applications of plant terpenoids in the synthesis of colloidal silver nanoparticles. Adv Colloid Interface Sci. 2016;234:132–141. doi: 10.1016/j.cis.2016.04.008.
- Shankar SS, Ahmad A, Sastry M. Geranium leaf assisted biosynthesis of silver nanoparticles. Biotechnol Prog. 2003;19(6):1627–1631. doi: 10.1021/bp034070w.
- Krishnaraj C, Ramachandran R, Mohan K, et al. Optimization for rapid synthesis of silver nanoparticles and its effect on phytopathogenic fungi. Spectrochim Acta A Mol Biomol Spectrosc. 2012;93:95–99. doi: 10.1016/j.saa.2012.03.002.
- Niraimathi KL, Sudha V, Lavanya R, et al. Biosynthesis of silver nanoparticles using Alternanthera sessilis (Linn.) extract and their antimicrobial, antioxidant activities. Colloids Surf B Biointerfaces. 2013;102:288–291. doi: 10.1016/j.colsurfb.2012.08.041.
- Kanipandian N, Kannan S, Ramesh R, et al. Characterization, antioxidant and cytotoxicity evaluation of green synthesized silver nanoparticles using cleistanthus collinus extract as surface modifier. Mater Res Bull. 2014;49:494–502. doi: 10.1016/j.materresbull.2013.09.016.
- Ahmed KBA, Senthilnathan R, Megarajan S, et al. Sunlight mediated synthesis of silver nanoparticles using redox phytoprotein and their application in catalysis and colorimetric mercury sensing. J Photochem Photobiol B. 2015;151:39–45. doi: 10.1016/j.jphotobiol.2015.07.003.
- Dhand V, Soumya L, Bharadwaj S, et al. Green synthesis of silver nanoparticles using Coffea arabica seed extract and its antibacterial activity. Mater Sci Eng C Mater Biol Appl. 2016;58:36–43. doi: 10.1016/j.msec.2015.08.018.
- Jain S, Mehata MS. Medicinal plant leaf extract and pure flavonoid mediated green synthesis of silver nanoparticles and their enhanced antibacterial property. Sci Rep. 2017;7(1):15867. doi: 10.1038/s41598-017-15724-8.
- Khatami M, Varma RS, Zafarnia N, et al. Applications of green synthesized Ag, ZnO and Ag/ZnO nanoparticles for making clinical antimicrobial wound-healing bandages. Sustainable Chem Pharm. 2018;10:9–15. doi: 10.1016/j.scp.2018.08.001.
- Valsalam S, Agastian P, Arasu MV, et al. Rapid biosynthesis and characterization of silver nanoparticles from the leaf extract of Tropaeolum majus L. and its enhanced in-vitro antibacterial, antifungal, antioxidant and anticancer properties. J Photochem Photobiol B. 2019;191:65–74. doi: 10.1016/j.jphotobiol.2018.12.010.
- Bindhu MR, Umadevi M, Esmail GA, et al. Green synthesis and characterization of silver nanoparticles from Moringa oleifera flower and assessment of antimicrobial and sensing properties. J Photochem Photobiol B. 2020;205:111836. doi: 10.1016/j.jphotobiol.2020.111836.
- Jalilian F, Chahardoli A, Sadrjavadi K, et al. Green synthesized silver nanoparticle from allium ampeloprasum aqueous extract: characterization, antioxidant activities, antibacterial and cytotoxicity effects. Adv Powder Technol. 2020;31(3):1323–1332. doi: 10.1016/j.apt.2020.01.011.
- Le NTT, Trinh BTD, Nguyen DH, et al. The physicochemical and antifungal properties of eco-friendly silver nanoparticles synthesized by psidium guajava leaf extract in the comparison with Tamarindus indica. J Clust Sci. 2021;32(3):601–611. doi: 10.1007/s10876-020-01823-6.
- Rai A, Bhaskar S, Reddy N, et al. Cellphone-aided attomolar zinc ion detection using silkworm protein-based nanointerface engineering in a plasmon-coupled dequenched emission platform. ACS Sustainable Chem Eng. 2021;9(44):14959–14974. doi: 10.1021/acssuschemeng.1c05437.
- Bhaskar S, Rai A, Ganesh KM, et al. Sericin-based bio-inspired nano-engineering of heterometallic AgAu nanocubes for attomolar mefenamic acid sensing in the mobile phone-based surface plasmon-coupled interface. Langmuir. 2022;38(39):12035–12049. doi: 10.1021/acs.langmuir.2c01894.
- Anastas P, Eghbali N. Green chemistry: principles and practice. Chem Soc Rev. 2010;39(1):301–312. doi: 10.1039/b918763b.
- Loo YY, Chieng BW, Nishibuchi M, et al. Synthesis of silver nanoparticles by using tea leaf extract from Camellia sinensis. Int J Nanomed. 2012;7:4263–4267.
- Nadagouda MN, Varma RS. Green synthesis of silver and palladium nanoparticles at room temperature using coffee and tea extract. Green Chem. 2008;10(8):859–862. doi: 10.1039/b804703k.
- Moulton MC, Braydich-Stolle LK, Nadagouda MN, et al. Synthesis, characterization and biocompatibility of “green” synthesized silver nanoparticles using tea polyphenols. Nanoscale. 2010;2(5):763–770. doi: 10.1039/c0nr00046a.
- Onitsuka S, Hamada T, Okamura H. Preparation of antimicrobial gold and silver nanoparticles from tea leaf extracts. Colloids Surf B Biointerfaces. 2019;173:242–248. doi: 10.1016/j.colsurfb.2018.09.055.
- Reygaert WC. Green tea catechins: their use in treating and preventing infectious diseases. Biomed Res Int. 2018;2018:9105261. doi: 10.1155/2018/9105261.
- Musial C, Kuban-Jankowska A, Gorska-Ponikowska M. Beneficial properties of green tea catechins. IJMS. 2020;21(5):1744. doi: 10.3390/ijms21051744.
- Marimuthu S, Antonisamy AJ, Malayandi S, et al. Silver nanoparticles in dye effluent treatment: a review on synthesis, treatment methods, mechanisms, photocatalytic degradation, toxic effects and mitigation of toxicity. J Photochem Photobiol B. 2020;205:111823. doi: 10.1016/j.jphotobiol.2020.111823.
- Vanaja M, Paulkumar K, Baburaja M, et al. Degradation of methylene blue using biologically synthesized silver nanoparticles. Bioinorg Chem Appl. 2014;2014:742346. doi: 10.1155/2014/742346.
- Edison TJI, Sethuraman MG. Instant green synthesis of silver nanoparticles using Terminalia chebula fruit extract and evaluation of their catalytic activity on reduction of methylene blue. Process Biochem. 2012;47(9):1351–1357. doi: 10.1016/j.procbio.2012.04.025.
- Kumar P, Govindaraju M, Senthamilselvi S, et al. Photocatalytic degradation of methyl orange dye using silver (Ag) nanoparticles synthesized from Ulva lactuca. Colloids Surf B Biointerfaces. 2013;103:658–661. doi: 10.1016/j.colsurfb.2012.11.022.
- Tahir K, Nazir S, Li B, et al. An efficient photo catalytic activity of green synthesized silver nanoparticles using Salvadora persica stem extract. Sep Purif Technol. 2015;150:316–324. doi: 10.1016/j.seppur.2015.07.012.
- Roy K, Sarkar CK, Ghosh CK. Photocatalytic activity of biogenic silver nanoparticles synthesized using yeast (Saccharomyces cerevisiae) extract. Appl Nanosci. 2015; 5(8):953–959. doi: 10.1007/s13204-014-0392-4.
- Bhaskar S, Jha P, Subramaniam C, et al. Multifunctional hybrid soret nanoarchitectures for mobile phone-based picomolar Cu2+ ion sensing and dye degradation applications. Physica E. 2021;132:114764. doi: 10.1016/j.physe.2021.114764.
- Bhaskar S, Srinivasan V, Ramamurthy SS. Nd2O3-Ag nanostructures for plasmonic biosensing, antimicrobial, and anticancer applications. ACS Appl Nano Mater. 2023;6(2):1129–1145. doi: 10.1021/acsanm.2c04643.
- Vilas V, Philip D, Mathew J. Catalytically and biologically active silver nanoparticles synthesized using essential oil. Spectrochim Acta A Mol Biomol Spectrosc. 2014;132:743–750. doi: 10.1016/j.saa.2014.05.046.
- Gavade NL, Kadam AN, Suwarnkar MB, et al. Biogenic synthesis of multi-applicative silver nanoparticles by using Ziziphus Jujuba leaf extract. Spectrochim Acta A Mol Biomol Spectrosc. 2015;136:953–960. doi: 10.1016/j.saa.2014.09.118.
- Rafique M, Sadaf I, Tahir MB, et al. Novel and facile synthesis of silver nanoparticles using Albizia procera leaf extract for dye degradation and antibacterial applications. Mater Sci Eng C Mater Biol Appl. 2019;99:1313–1324. doi: 10.1016/j.msec.2019.02.059.
- Khan AU, Yuan Q, Wei Y, et al. Ultra-efficient photocatalytic deprivation of methylene blue and biological activities of biogenic silver nanoparticles. J Photochem Photobiol B. 2016;159:49–58. doi: 10.1016/j.jphotobiol.2016.03.017.
- Nouri A, Tavakkoli Yaraki M, Lajevardi A, et al. Ultrasonic-assisted green synthesis of silver nanoparticles using Mentha aquatica leaf extract for enhanced antibacterial properties and catalytic activity. Colloids Interface Sci Commun. 2020;35:100252. doi: 10.1016/j.colcom.2020.100252.
- Martí M, Frígols B, Serrano-Aroca A. Antimicrobial characterization of advanced materials for bioengineering applications. J Vis Exp. 2018;(138):e57710. doi:10.3791/57710.
- Pryshchepa O, Pomastowski P, Buszewski B. Silver nanoparticles: synthesis, investigation techniques, and properties. Adv Colloid Interface Sci. 2020;284:102246. doi: 10.1016/j.cis.2020.102246.
- Tarannum N, Divya, Gautam YK. Facile green synthesis and applications of silver nanoparticles: a state-of-the-art review. RSC Adv. 2019;9(60):34926–34948. doi: 10.1039/c9ra04164h.
- Chandra A, Bhattarai A, Yadav AK, et al. Green synthesis of silver nanoparticles using tea leaves from three different elevations. ChemistrySelect. 2020;5(14):4239–4246. doi: 10.1002/slct.201904826.
- Rolim WR, Pelegrino MT, de Araújo Lima B, et al. Green tea extract mediated biogenic synthesis of silver nanoparticles: characterization, cytotoxicity evaluation and antibacterial activity. Appl Surf Sci. 2019;463:66–74. doi: 10.1016/j.apsusc.2018.08.203.
- Prathna TC, Chandrasekaran N, Raichur AM, et al. Biomimetic synthesis of silver nanoparticles by citrus Limon (lemon) aqueous extract and theoretical prediction of particle size. Colloids Surf B Biointerfaces. 2011;82(1):152–159. doi: 10.1016/j.colsurfb.2010.08.036.
- Bindhu MR, Umadevi M. Synthesis of monodispersed silver nanoparticles using Hibiscus cannabinus leaf extract and its antimicrobial activity. Spectrochim Acta A Mol Biomol Spectrosc. 2013;101:184–190. doi: 10.1016/j.saa.2012.09.031.
- Jadhav K, Dhamecha D, Bhattacharya D, et al. Green and ecofriendly synthesis of silver nanoparticles: characterization, biocompatibility studies and gel formulation for treatment of infections in burns. J Photochem Photobiol B. 2016;155:109–115. doi: 10.1016/j.jphotobiol.2016.01.002.
- Mishra A, Kaushik NK, Sardar M, et al. Evaluation of antiplasmodial activity of green synthesized silver nanoparticles. Colloids Surf B Biointerfaces. 2013;111:713–718. doi: 10.1016/j.colsurfb.2013.06.036.
- Sudhakar C, Selvam K, Govarthanan M, et al. Acorus calamus rhizome extract mediated biosynthesis of silver nanoparticles and their bactericidal activity against human pathogens. J Genet Eng Biotechnol. 2015;13(2):93–99. doi: 10.1016/j.jgeb.2015.10.003.
- Wan Mat Khalir WKA, Shameli K, Jazayeri SD, et al. Biosynthesized silver nanoparticles by aqueous stem extract of Entada spiralis and screening of their biomedical activity. Front Chem. 2020;8:620. doi: 10.3389/fchem.2020.00620.
- Veerasamy R, Xin TZ, Gunasagaran S, et al. Biosynthesis of silver nanoparticles using mangosteen leaf extract and evaluation of their antimicrobial activities. J Saudi Chem Soc. 2011;15(2):113–120. doi: 10.1016/j.jscs.2010.06.004.
- Martínez-Castañón GA, Niño-Martínez N, Martínez-Gutierrez F, et al. Synthesis and antibacterial activity of silver nanoparticles with different sizes. J Nanopart Res. 2008;10(8):1343–1348. doi: 10.1007/s11051-008-9428-6.
- Rajkumar T, Sapi A, Das G, et al. Biosynthesis of silver nanoparticle using extract of Zea mays (corn flour) and investigation of its cytotoxicity effect and radical scavenging potential. J Photochem Photobiol B. 2019;193:1–7. doi: 10.1016/j.jphotobiol.2019.01.008.
- Sun Q, Cai X, Li J, et al. Green synthesis of silver nanoparticles using tea leaf extract and evaluation of their stability and antibacterial activity. Colloids Surf, A. 2014;444:226–231. doi: 10.1016/j.colsurfa.2013.12.065.
- Widatalla HA, Yassin LF, Alrasheid AA, et al. Green synthesis of silver nanoparticles using green tea leaf extract, characterization and evaluation of antimicrobial activity. Nanoscale Adv. 2022;4(3):911–915. doi: 10.1039/d1na00509j.
- Alfuraydi AA, Devanesan S, Al-Ansari M, et al. Eco-friendly green synthesis of silver nanoparticles from the sesame oil cake and its potential anticancer and antimicrobial activities. J Photochem Photobiol B. 2019;192:83–89. doi: 10.1016/j.jphotobiol.2019.01.011.
- Hemlata, Meena PR, Singh AP, et al. Biosynthesis of silver nanoparticles using cucumis prophetarum aqueous leaf extract and their antibacterial and antiproliferative activity against cancer cell lines. ACS Omega. 2020;5(10):5520–5528. doi: 10.1021/acsomega.0c00155.
- Tang S, Zheng J. Antibacterial activity of silver nanoparticles: structural effects. Adv Healthcare Mater. 2018;7(13):1701503. doi: 10.1002/adhm.201701503.
- Varadavenkatesan T, Selvaraj R, Vinayagam R. Phyto-synthesis of silver nanoparticles from mussaenda erythrophylla leaf extract and their application in catalytic degradation of methyl orange dye. J Mol Liq. 2016;221:1063–1070. doi: 10.1016/j.molliq.2016.06.064.
- Mallick K, Witcomb M, Scurrell M. Silver nanoparticle catalysed redox reaction: an electron relay effect. Mater Chem Phys. 2006;97(2–3):283–287. doi: 10.1016/j.matchemphys.2005.08.011.
- Vidhu VK, Philip D. Catalytic degradation of organic dyes using biosynthesized silver nanoparticles. Micron. 2014;56:54–62. doi: 10.1016/j.micron.2013.10.006.
- Al-Zaban MI, Mahmoud MA, AlHarbi MA. Catalytic degradation of methylene blue using silver nanoparticles synthesized by honey. Saudi J Biol Sci. 2021;28(3):2007–2013. doi: 10.1016/j.sjbs.2021.01.003.
- Raina S, Roy A, Bharadvaja N. Degradation of dyes using biologically synthesized silver and copper nanoparticles. Environ Nanotechnol Monit Manag. 2020;13:100278. doi: 10.1016/j.enmm.2019.100278.
- Azad UP, Ganesan V, Pal M. Catalytic reduction of organic dyes at gold nanoparticles impregnated silica materials: influence of functional groups and surfactants. J Nanopart Res. 2011;13(9):3951–3959. doi: 10.1007/s11051-011-0317-z.
- Jose PPA, Kala MS, Kalarikkal N, et al. Silver-attached reduced graphene oxide nanocomposite as an eco-friendly photocatalyst for organic dye degradation. Res Chem Intermed. 2018;44(9):5597–5621. doi: 10.1007/s11164-018-3443-8.
- Mortazavi-Derazkola S, Ebrahimzadeh MA, Amiri O, et al. Facile green synthesis and characterization of Crataegus microphylla extract-capped silver nanoparticles (CME@Ag-NPs) and its potential antibacterial and anticancer activities against AGS and MCF-7 human cancer cells. J Alloys Compd. 2020;820:153186. doi: 10.1016/j.jallcom.2019.153186.
- Maghimaa M, Alharbi SA. Green synthesis of silver nanoparticles from Curcuma longa L. and coating on the cotton fabrics for antimicrobial applications and wound healing activity. J Photochem Photobiol B. 2020;204:111806. doi: 10.1016/j.jphotobiol.2020.111806.