Lung surfactant is a mixture of phospholipids and proteins that is synthesized by alveolar type 2 cells and secreted into the alveoli to reduce surface tension at the air-liquid interface. Mammalian lung surfactant consists of approximately 80% phospholipids, 10% neutral lipids and 10% proteins. The discovery of surfactant deficiency as the cause of neonatal respiratory distress syndrome (RDS) in preterm infants in 1959 by Avery and Mead [Citation1] has been a starting point for the development of synthetic lung surfactants for intratracheal instillation in preterm infants. The first generation of synthetic lung surfactants included phospholipid mixtures, such as ALEC®, a mixture of dipalmitoylphosphatidylcholine (DPPC) and egg phosphatidylglycerol (PG), and Exosurf®, a mixture of DPPC with hexadecanol and tyloxapol. However, these pure phospholipid-based preparations had a limited clinical efficacy because they lacked surfactant proteins B and C (SP-B and SP-C) that enhance the surface-active properties of surfactant phospholipids. The second generation of synthetic lung surfactants therefore contained SP-B and/or SP-C peptide mimics, such as the SP-B analog KL4 (Surfaxin®) and recombinant human surfactant protein C (rhSP-C) (Venticute®). These synthetic lung surfactants were quickly overshadowed by the introduction of natural surfactants prepared by organic solvent extraction of bovine (Survanta®, Infasurf®, Alveofact®) or porcine (Curosurf®) lungs and consisting of phospholipids and small quantities (~1 wt%) of SP-B and SP-C. Clinical surfactants have had a tremendous effect on the outcome of preterm infants with RDS. In combination with antenatal corticosteroid therapy and advanced modes of ventilatory support, intratracheal administration of animal-derived lung surfactants has boosted survival and decreased morbidity of preterm infants born at the margins of viability. Potential side-effects of clinical surfactants due to cross-species transfer of infectious agents did not materialize, but high production costs and batch-to-batch variability have led to renewed interest in synthetic lung surfactants and the creation of third generation synthetic lung surfactants, using advanced SP-B and SP-C peptide mimics.
Both SP-B and SP-C have been sequenced and genetic studies have shown that hereditary SP-B deficiency causes fatal neonatal RDS [Citation2] and that SP-C mutations can lead to childhood interstitial lung disease [Citation3]. Discovery of the structures of SP-B and SP-C 30 years ago and advances in peptide synthesis promised a quick development of functional peptide mimics and synthetic lung surfactant for clinical use. However, the complex 3-D structure of native SP-B and the propensity of SP-C for amyloid formation of its transmembrane region have been quite a challenge in designing and producing highly functional, stable peptide mimics of SP-B and SP-C.
Human SP-B is an amphipathic, 79 amino-acid (MW ~8.7 kDa) saposin-like protein, consisting of 4–5 α-helices with three intramolecular disulfide bonds (i.e. Cys-8 to Cys-77, Cys-11 to Cys-71 and Cys-35 to Cys-46). The helical bundle for SP-B is folded into two leaves and held together with disulfide bridges, with one leaf having α-helices 1 (N-terminal helix), 5 (C-terminal helix) and 4 and the second composed of α-helices 2 and 3 [Citation4,Citation5]. Using truncated synthetic peptides (e.g. SP-B1–25, ~residues 1-25; SP-B4949–66, ~residues 49-66) that encompassed α-helices predicted from 3D-saposin homology comparisons, we found that N- and C-terminal peptides not only interacted with phospholipids similarly to that of native SP-B but also partially mimicked in vitro and in vivo activities of the parent protein [Citation6]. Refinement of this approach led to the more advanced Mini-B and Super Mini-B mimics [Citation4]. For example, Mini-B is a 34-residue, ‘short-cut’ peptide that incorporates the N-terminal α-helix (~residues 8–25) and C-terminal α-helix (~residues 63–78) of native SP-B, joined with a customized turn and cross-linked with two vicinal disulfide bonds (i.e. Cys-8 to Cys-77 and Cys-11 to Cys-71) to form an α-helix hairpin. Mini-B shows high surface activity in vitro and in animal models of surfactant deficiencies, which may be due to this mimic accurately reproducing the topology of the N- and C-terminal domains in the native SP-B [Citation5]. Adding the hydrophobic N-terminal insertion sequence of SP-B (i.e. residues 1–7) to Mini-B led to ‘Super Mini-B’ [Citation7]. Super Mini-B demonstrates excellent in vitro and in vivo surface activity after formulation with phospholipids [Citation8], which may be a consequence of both the cross-linked α-helix hairpin and deeper insertion of the peptide into the lipid bilayer ().
Figure 1. Molecular illustration of a phospholipid bilayer cross section with Super Mini B (SMB) peptide interacting with the synthetic surfactant lipid bilayer. SMB, an amphipathic helix hairpin peptide mimic of full-length SP-B, is shown with the N-terminal helix highlighted in red, the bend connecting the N and C terminal domains in orange and the C-terminal helix in blue. The cystine disulfide linkages are highlighted in yellow, while the N-terminal phenylalanine is in magenta. The synthetic surfactant lipid mixture consists of DPPC, POPC and POPG in the mole ratio of 5:3:2. The acyl chains are rendered in green while phospholipid head group atoms are nitrogen in blue and phosphate atoms in orange. The graphic image is based on the homology templated structure of SMB using iTasser that was inserted into a bilayer of synthetic surfactant lipids with the Charmm graphical user interface. This peptide-bilayer lipid ensemble was then refined by molecular dynamics using the Gromacs force field and Charmm36 m parameter set for one microsecond to reach an equilibrium state. Water molecules used in the simulation have been deleted from the illustration for clarity.
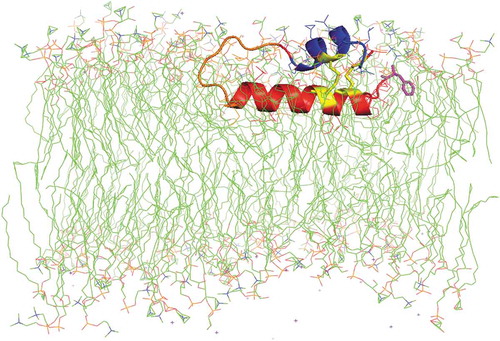
Revak and Cochrane imitated the α-helical structure of SP-B with a 21-residue peptide alternating one lysine residue with a sequence of 4 leucine residues (KL4) [Citation9]. KL4-surfactant (Surfaxin®) received FDA approval for the prevention of RDS in 2012, but production was discontinued in 2015. Except that it was not approved for treatment of preterm infants with neonatal RDS, Surfaxin® had a practical problem because it does not associate with phospholipids as readily as SP-B, forms a gel in its storage form and must be heated to 44°C and shaken before administration, which complicates its ease of clinical use. In our hands, KL4-based surfactant was inferior to peptide constructs based on the amino-acid sequence and the 3D-saposin motif of SP-B.
SP-C is an extremely hydrophobic 35 amino-acid (MW ~4.2 kDa) protein with two palmitoyl chains bound to cysteines in positions 4 and 5 via labile thioester bonds and an α-helix formed by amino acid residues 9–34. The first functional SP-C analog was developed by the respiratory pharmacology group of Häfner et al. with recombinant DNA technology [Citation10]. This recombinant human SP-C analog (rhSP-C) had a 34 amino-acid sequence with phenylalanine instead of cysteine in positions 4 and 5 (SP-Cff) and isoleucine instead of methionine in position 32. However, palmitoylation of SP-C proved to be essential to sustain the proper dynamics of lung surfactant and the results of several large multicenter studies using rhSP-C-based surfactant (Venticute®) as treatment for the acute respiratory distress syndrome gave disappointing results. This problem was first tackled by Johansson et al. [Citation11], who replaced the palmitoylated cysteines in the N-terminal of full-length SP-C with serine and retained the transmembrane helical domain by substituting leucines for valines (SP-C33). SP-C33 has shown to have good in vitro and in vivo surface activity, especially in combination with an SP-B peptide mimic [Citation12]. Our group took a different approach by replacing the cysteine-palmitoyl moieties in the N-terminal of SP-C with serines and maintaining the α-helix with intrapeptide salt-bridges (aka, ‘ion-locks’) that penetrate deeply into the surfactant lipid bilayer [Citation13], thereby creating a stable SP-C peptide mimic with elevated in vitro and in vivo activities (). The group of Barron explored the use of helical poly-N-substituted glycines (peptoids) that imitate the surface activity of SP-B and SP-C and are resistant to protease degradation [Citation14]. Peptoids are non-natural compounds with a polypeptide backbone and the side chains are appended to the backbone nitrogens rather than the α-carbons as in peptide mimics. A recent study suggests reasonable in vivo functionality of peptoids in surfactant-deficient rats [Citation14], but data on clearance and metabolism of these peptoids in the lung are not yet available.
Figure 2. Molecular illustration of a phospholipid bilayer cross section with SP-C ion lock-1 protein interacting with the synthetic surfactant lipid bilayer. SP-C ion lock-1 is a transbilayer helical protein mimic of full-length SP-C. The protein is shown with the transmembrane helix highlighted in red, the N-terminal surface domain in blue and the C-terminal segment in orange. The synthetic surfactant lipid mixture consists of DPPC:POPC:POPG in the mole ratio of 5:3:2. The acyl chains are rendered in green while phospholipid head group atoms are nitrogen in blue and phosphate atoms in orange. The protein structure is based on the homology templated structure of SP-C ion lock-1 using iTasser that was inserted into a bilayer of synthetic surfactant lipids with the Charmm graphical user interface. This peptide-bilayer lipid ensemble was then refined by molecular dynamics using the Gromacs force field and Charmm36 m parameter set for 0.5 microseconds to reach an equilibrium state. Water molecules used in the simulation have been deleted from the illustration for clarity.
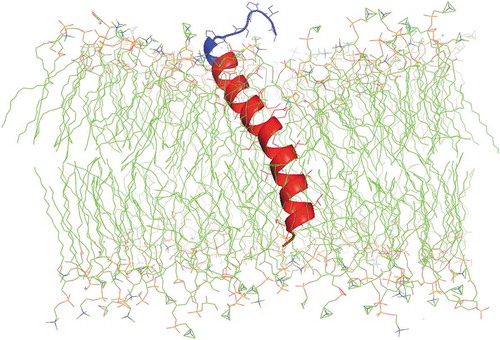
Availability of highly functional SP-B and SP-C analogs has led to the development of third generation synthetic lung surfactants consisting of a combination of 0.2% Mini-B and 1.5% SP-C33 in a 1:1 DPPC:palmitoyl-oleoyl-phosphoglycerol (POPG) mixture (CHF5633, Chiesi Farmaceutici, Parma, Italy) [Citation12] and 3% Super Mini-B in DPPC:POPC:POPG 5:3:2 (mole:mole) surfactant lipids (MiniSurf®, Molecular Express Inc., Rancho Dominguez, CA). CHF5633 has recently shown clinical efficacy in the treatment of preterm infants with neonatal RDS [Citation15], whereas MiniSurf® is at a preclinical stage. In contrast with previous synthetic lung surfactants, these preparations demonstrate in vitro and in vivo surface activity that equals that of clinical surfactants.
After 30 years two advanced synthetic lung surfactant preparations may in the foreseeable future reach the neonatal intensive care nursery for treatment of preterm infants with RDS. Both preparations are in liquid form for intratracheal instillation via an endotracheal tube during mechanical ventilation or through a thin catheter in the trachea (less invasive surfactant administration, LISA [Citation16]) in spontaneously breathing infants supported with nasal continuous airway pressure (nCPAP) or non-invasive positive pressure ventilation (NIPPV). These surfactants will undoubtedly be embraced by neonatal clinicians in case their introduction increases availability and decreases the costs of surfactant treatment. However, critical neonatal trials are required to demonstrate the efficacy of these surfactants in improving outcome. One of the curses of mechanical ventilation in preterm infants is the development of chronic lung disease (bronchopulmonary dysplasia, BPD) that may affect lung function on a permanent basis. Moving away from routine intubation and mechanical ventilation at birth to continuous use of nCPAP in order to try to reduce the incidence and severity of BPD has led to dramatic changes in ventilatory management of even the youngest preterm infants [Citation17]. Up so far, the concept has only been partially successful as the incidence of BPD among large cohorts of extremely preterm infants remains unacceptably high despite the introduction of noninvasive ventilation. Although the LISA methodology enables surfactant administration without interruption of noninvasive respiratory support during spontaneous breathing, aerosol delivery of synthetic lung surfactant may prove to be an important step forward to phase out intratracheal placement of a tube or catheter for surfactant treatment. Recent animal studies with aerosol delivery of liquid [Citation18] and dry powder [Citation19] synthetic lung surfactant support the potential clinical applicability of aerosol delivery of synthetic lung surfactant. We may not have found the holy grail of neonatal pulmonology yet, but the last 30 years have taught us how to optimize respiratory support for preterm infants with RDS using animal-derived clinical lung surfactant and put us on track with an equivalent synthetic lung surfactant product.
Declaration of interest
The authors hold patents in a series of synthetic surfactant peptides, including SP-B1-25, Mini-B, Super Mini-B, and SP-C ion lock-1. The first author has been a scientific consultant for Chiesi Farmaceutici SpA and Molecular Express Inc.
Reviewers disclosure
A reviewer on this manuscript has disclosed being a scientific consultant for Chiesi Farmaceutici SPA. Peer reviewers on this manuscript have no other relevant financial relationships or otherwise to disclose.
Additional information
Funding
References
- Avery ME, Mead J. Surface properties in relation to atelectasis and hyaline membrane disease. AMA J Dis Child. 1959;97(5,Part 1):517–523.
- Nogee LM, Wert SE, Proffit SA, et al. Allelic heterogeneity in hereditary surfactant protein B (SP-B) deficiency. Am J Respir Crit Care Med. 2000;161:973–981.
- Griese M, Lorenz E, Hengst M, et al. Surfactant proteins in pediatric interstitial lung disease. Pediatr Res. 2016;79(1–1):34–41.
- Waring A, Taeusch W, Bruni R, et al. Synthetic amphipathic sequences of surfactant protein-B mimic several physicochemical and in vivo properties of native pulmonary surfactant proteins. Pept Res. 1989;2(5):308–313.
- Walther FJ, Gordon LM, Waring AJ. Design of surfactant protein B peptide mimics based on the saposin fold for synthetic lung surfactants. Biomed Hub. 2016;1(3).
- Waring AJ, Walther FJ, Gordon LM, et al. The role of charged amphipathic helices in the structure and function of surfactant protein B. J Pept Res. 2005;66(6):364–374.
- Walther FJ, Waring AJ, Hernandez-Juviel JM, et al. Critical structural and functional roles for the N-terminal insertion sequence in surfactant protein B analogs. PLoS One. 2010;5(1):e8672.
- Waring AJ, Gupta M, Gordon LM, et al. Stability of an amphipathic helix-hairpin surfactant peptide in liposomes. Biochim Biophys Acta. 2016;1858(12):3113–3119.
- Revak SD, Merritt TA, Hallman M, et al. The use of synthetic peptides in the formation of biophysically and biologically active pulmonary surfactants. Pediatr Res. 1991;29(5):460–465.
- Davis AJ, Jobe AH, Häfner D, et al. Lung function in premature lambs and rabbits treated with a recombinant SP-C surfactant. Am J Respir Crit Care Med. 1998;157(2):553–559.
- Johansson J, Some M, Linderholm BM, et al. A synthetic surfactant based on a poly-Leu SP-C analog and phospholipids: effects on tidal volumes and lung gas volumes in ventilated immature newborn rabbits. J Appl Physiol (1985). 2003;95(5):2055–2063.
- Ricci F, Murgia X, Razzetti R, et al. In vitro and in vivo comparison between poractant alfa and the new generation synthetic surfactant CHF5633. Pediatr Res. 2017;81(2):369–375.
- Walther FJ, Waring AJ, Hernández-Juviel JM, et al. Surfactant protein C peptides with salt-bridges (“ion-locks”) promote high surfactant activities by mimicking the α-helix and membrane topography of the native protein. PeerJ. 2014;2:e485.
- Czyzewski AM, McCaig LM, Dohm MT, et al. Effective in vivo treatment of acute lung injury with helical, amphipathic peptoid mimics of pulmonary surfactant proteins. Sci Rep. 2018;8(1):6795.
- Sweet DG, Turner MA, Straňák Z, et al. A first-in-human clinical study of a new SP-B and SP-C enriched synthetic surfactant (CHF5633) in preterm babies with respiratory distress syndrome. Arch Dis Child Fetal Neonatal Ed. 2017;102(6):F497–F503.
- Härtel C, Paul P, Hanke K, et al. Less invasive surfactant administration and complications of preterm birth. Sci Rep. 2018 May 29;8(1):8333.
- Mulder EE, Lopriore E, Rijken M, et al. Changes in respiratory support of preterm infants in the last decade: are we improving? Neonatology. 2012;101(4):247–253.
- Walther FJ, Hernández-Juviel JM, Waring AJ. Aerosol delivery of synthetic lung surfactant. PeerJ. 2014;2:e403.
- Walther FJ, Gupta M, Lipp MM, et al. Aerosol delivery of dry powder synthetic lung surfactant to surfactant-deficient rabbits and preterm lambs on non-invasive respiratory support. Gates Open Res. 2019;3:6.