Abstract
A mild and efficient synthetic method has been developed for the preparation of biologically important quinoxalines in excellent yield from relatively safe precursor α-bromoketones and 1,2-diamines using catalytic amount of micellar sodium dodecyl sulfate in water at ambient temperature. The method is also found effective for the introduction of quinoxaline moiety into the ring A of pentacyclic triterpenoid, friedelin. Ambient reaction conditions, renewable catalytic condition, inherently safer chemistry, excellent product yields, and water as a reaction medium display both economic and environmental advantages.
Introduction
Quinoxalines are ubiquitous heterocyclic units in pharmaceuticals and bioactive natural products Citation1–4. They are used as pharmaceuticals and antibiotics such as echinomycin, levomycin, and actinoleutin which are known to inhibit the growth of Gram-positive bacteria and are also active against various transplantable tumors Citation1–3. Antitumoral properties of quinoxaline compounds have also been investigated Citation4. Beside these, they are well known for their application in dyes Citation5 as an efficient electroluminescent materials Citation6 in organic semiconductors Citation7 as building blocks for the synthesis of anion receptors Citation8 as cavitands Citation9 Citation10, dehydroannulenes Citation11, and DNA cleaving agents Citation12,Citation13. Conventionally, quinoxalines are synthesized by a double condensation reaction involving a dicarbonyl precursor and o-phenylenediamine Citation14,Citation15. Due to the highly reactive nature of the dicarbonyls, alternative routes have been proposed recently Citation16. Antoniotti and Donach Citation16 have reported one of these methods to synthesize quinoxalines from epoxides and ene-1,2-diamines. Active manganese oxide and molecular sieves in combination or manganese oxides in combination with microwaves have also been used in producing quinoxalines Citation17,Citation18. These processes, however, require excessive amounts of corrosive manganese oxide as stoichiometric oxidants and scaling them up for industrial processes can lead to the formation of large amounts of toxic waste leading to environmental issues. In additional studies, Robinson and Taylor reported a homogeneous catalytic process utilizing Pd(OAc)2, RuCl2 (PPh3)2 to synthesize quinoxalines from hydroxy ketones Citation19 and recently a copper catalyzed oxidative cyclization process has been reported Citation20. An improved ruthenium catalyzed using direct approach to synthesize quinoxalines from diols and o-diamines have also been reported Citation21. These processes suffer from the major drawback that the catalysts are expensive, toxic, and cannot be recovered and reused. In addition to the above catalytic methods, synthesis of quinoxalines using zeolites Citation22–25 microwave Citation26,Citation27 and solid supports Citation28–30 has also been reported. Nevertheless, these methods suffer from unsatisfactory product yields, critical product isolation procedures, expensive, and detrimental metal precursors and harsh reaction conditions, which limit their use as environmentally friendly protocol. In addition most of the reported methods are not recommended as a clean protocol.
Although very few of the recent reports have claimed α-bromoketones as an equivalent safe chemical precursor of α-hydroxyketones, epoxides, or dicarbonyls as reaction partners of o-phenylenediamine to prepare quinoxalines Citation31,Citation32 they involved the use of either HClO4-SiO2 or TMSCl as catalyst. Although useful, HClO4 has huge hazardous nature than its potential usefulness, whereas those catalyzed by TMSCl needs higher temperature, with lower yield of the desired products not satisfying the principles of green chemistry protocol in contemporary science as well as their acceptance for industrial applications.
In this context the development of an alternative route to quinoxaline from less reactive α-bromoketones in aqueous medium was felt necessary not only due to the increased regulatory pressure focusing on organic solvents, but also because of the emphasis given toward the development of green protocol for organic synthesis nowadays.
The use of water as a medium for organic synthesis is one of the latest challenges in organic synthesis. Reactions in water emerged as a useful alternative route for several organic reactions owing to many of its potential advantages such as safety, economy, and friendly toward catalytic and stereoselective processes and more importantly of environmental concern Citation32,Citation33 and the progress has been dramatic. In addition, water facilitates ion separation through salvation which often results in altered behavior of reactants in an aqueous environment. Keeping these above facts in mind, we recently have tested water as a solvent in many of our ongoing studies toward organic syntheses and transformative reactions. Very recently we have reported Citation33 the selective synthesis of 1,2-disubstituted benzimidazole in water catalyzed by sodium dodecyl sulfate (SDS), and now reporting herein the result of another successful attempt for an efficient synthesis of quinoxaline from α-bromoketones and o-phenylenediamine mediated by water and catalyzed by SDS at room temperature in excellent yields. This is the first report of synthesizing quinoxaline derivatives in a very mild way in water catalyzed by nucleophilic SDS Citation33 at ambient temperature starting from the less reactive safer precursor α-bromoketones in an efficient manner.
Results and discussion
Initially, efforts were directed toward the evaluation of catalytic ability of SDS for the synthesis of quinoxalines. Preliminary studies using phenacylbromide (1 mmol) and o-phenylenediamine (1 mmol) without SDS in water at room temperature did not afford the desired quinoxaline. Increase of the reaction time, temperature or by changing the molar proportion of the reactants did not make any influence on the course of the reaction. Addition of some common salts like NaCl, NH4Cl, KBr, etc. had no positive effect on the reaction. Similar molar ratios of substrates in tap water yielded the desired product Citation3 only in presence of catalytic amounts of SDS. The modified method gave excellent yield of the product within 6 hours at room temperature (). Thus, the catalytic role of SDS in the present transformation is well established.
This excellent catalyzing ability of SDS inspired us to investigate the above transformation in details. In order to evaluate an optimized and general reaction protocol, a couple of experiments were carried out () using varying amounts of SDS (0.34, 0.17, 0.06, 0.03, 0.02, and 0.01 mol%) in combination with different types (both cationic and anionic) and proportions of surfactants viz. tetra-n-butylammonium bromide, cetyltrimethylammonium bromide, cetyl pyridinium chloride, sodium dodecylbenzenesulfonate, and tetra-n-butylammonium iodide, in different reaction conditions for the above model study (). It is interesting to note that, although all the surfactants can afford Citation3 as the major product but their combination with SDS showed excellent selectivity not only in forming the desired product Citation3 but also in directing the reaction to proceed in a very cleaner way (Entry 1–7, ). Thus it was established that, α-bromoketone (1 mmol) and 1,2-diamine (1 mmol) in water (3 ml) gave the best result within 6 hours in presence of SDS (10 mg, 0.03 mol%) at room temperature.
Table 1. Optimization of quinoxaline synthesis using phenacyl bromide and o-phenylenediamine in presence of different surfactants and their amounts.
It was also observed that during the reaction the substrates and reactants do not mix together in water; addition of SDS not only raised the solubility of the components in water but also catalyzed the process tremendously. Addition of catalytic amount of SDS (0.03 mol%) turned the reaction mixture into a clear yellowish colored solution that slowly transferred into reddish yellow as the reaction progressed. After completion of the reaction (checked by Thin layer chromatography (TLC)), products were purified by simple filtration (and in some cases by column chromatography, silica 60–120 mesh) followed by crystallization to get the products in good to excellent yields. Appendix.
In order to demonstrate the versatility of SDS as a catalyst for the synthesis of quinoxalines, a series of α-bromoketones and 1,2-diamines were subjected to undergo one pot condensation–aromatization in presence of SDS under the optimized reaction protocol (). All of the reactions tried showed good selectivity with excellent isolated yields. Appendix.
Table 2. Preperation of quinoxaline derivatives.
While investigating the influence of the substituents present either on ketone part or on 1,2-diamine on the course of the reaction, it was observed that compounds having electron donating or withdrawing groups on the ketone (Entry 2, 3, 5–9, of ) both underwent the reactions in almost similar fashion and gave good yields. Although, p-bromo phenacylbromide (Entry 5, of ) gave better yield than its meta isomer (Entry 9, of ), the corresponding p-nitro and m-nitro derivative underwent the reaction in identical fashion (Entry 10, of ). Disubstituted α-bromoketones (Entry 11, 12, of ) also gave excellent yields of the expected quinoxalines. Sensitive molecules like 1,2-diaminomalionitrile (Entry 15, of ) was also found compatible to the reaction condition and gave 84% yield of the corresponding quinoxaline. All the observed results were summarized in .
Potential of pentacyclic triterpenoid as bioactive candidate is well described Citation34. In order to see the effect on their bioactivities by the introduction of quinoxaline ring on ring A, we applied our protocol on 2α-bromofriedelin Citation5 (prepared from friedelin) Citation4 and to our delight we have isolated the corresponding quinoxaline derivative Citation6 in 58% yield within 8 hours under identical condition (0.03 mol% of SDS). This is also the very first report of preparing quinoxaline derivative of pentacyclic triterpenoid in water at room temperature ().
As was mentioned earlier, a simple filtration or easy work up procedure of the reaction and reuse of the catalyst, SDS directly from the aqueous extract of the reaction mixture for a fresh run, are the great advantages of the developed process. Gratifyingly, it was tested that the recovered water layer can be reused for six consecutive runs ().
Table 3. Recycling experiment using sodium dodecyl sulfate.
It is well known that under ambient condition surfactant molecules can aggregate in an aqueous phase to micelles with hydrophobic core and a hydrophilic corona Citation35,Citation36. To determine whether micellization had occurred or not we first measured the critical micellization concentration (CMC) of SDS () and the value was found to be 8.33 mM. In the present study, under the optimized reaction condition the concentration of SDS was 11.57 mM (10 mg of SDS in 3 ml water). Since the value was far beyond the CMC value of SDS (8.33 mM), micellization was anticipated.
Figure 1. Plot of conductance vs. concentration of sodium dodecyl sulfate (SDS) for the calculation of critical micellization concentration value of SDS.
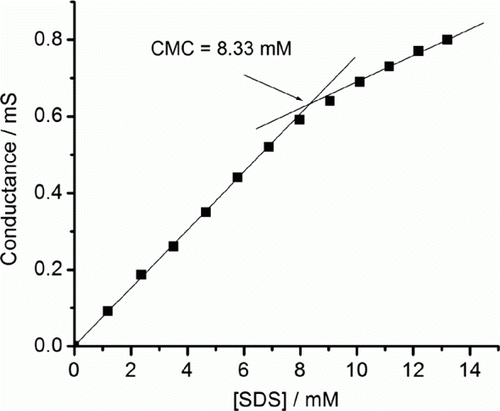
It was reported in the literature Citation37 that the dimensionless packing parameter P of the molecular geometry as an index to predict the size and shape of the micelles. P was defined as V/(a 0 l), where V is the hydrocarbon chain volume, a 0 is the optimum head group area per molecule, and l is the hydrocarbon chain length that is taken to be ca. 80–90% of the fully extended chain length Citation37. The overall prediction was concluded as follows:
Spherical micelles P <1/3 | |||||
Cylindrical micelles 1/3 < P <1/2 | |||||
Bilayers (or vesicles) 1/2 < P <1 | |||||
Inverted structures P >1 |
The value of packing parameter P, an index to predict the size and shape of the micelles Citation37, of SDS was found to be 0.235 (taking l as 90% of the fully extended chain length) indicating the spherical nature of the developed micelles.
For further confirmation dynamic light scattering (DLS) measurement was carried out of a 11.57 mM aqueous solution of SDS that indicated the presence of micelles () of radius 161 nm (diameter of 322 nm) with the Polydispersity index of 0.348.
Figure 2. Graph of intensity vs. size (nm) of the micelles based on dynamic light scattering measurement.
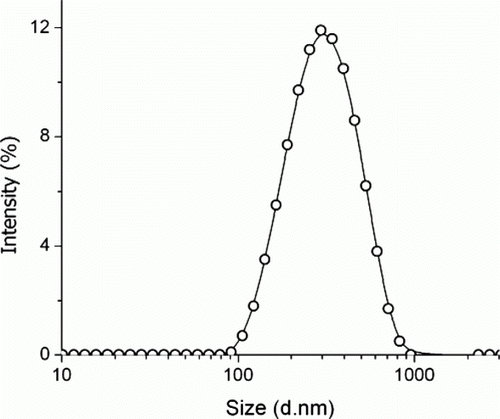
The role of SDS as a nucleophile is well investigated in our previous communication Citation33. Considering the above characteristics of SDS, the most probable mechanism of the miceller SDS in effecting the present transformation may be depicted as shown in . In the miceller solution, 1,2-phenylenediamine and phenacyl bromide, both of which are hydrophobic in nature, are entered into the hydrophobic core of the micelles and thus assist the condensation between the phenacyl bromide and o-phenylenediamine to form dihydroquinoxaline derivative (A) (). Nucleophilic nature of SDS may have assisted the in situ aromatization of the dihydro derivative (A) to afford quinoxalines ().
Experimental
General
All the chemicals used were reagent grade and purified prior to their use. SDS was purchased from Sigma-Aldrich, India. The NMR chemical shift was reported in ppm relative to 7.20–77.0 ppm of CDCl3 solvent as the standards. 1H spectra were recorded in 300 MHz frequencies and 13C NMR spectra were recorded in 75.4 MHz frequencies. Coupling constant “J” was calculated in Hertz. Conductance was measured in Systronics conductivity meter 304 at 25°C (298 K). DLS measurement was performed in Nano ZS90 (Malvern, UK).
General procedure for quinoxalines
In a typical experimental procedure, o-phenlylenediamine (1 mmol) and α-bromoketone (1 mmol) in 1:1 molar ratios was taken in a 50 ml round bottom flask. To this water (3 ml) and 10 mg (0.03 mol%) SDS was admixed. The reaction mixture was then allowed to stir with magnetic spinning bar at room temperature. After the completion of the reaction (checked by TLC), the residue was filtered, washed with water, dried and finally recrystallized from methanol. The desired pure product was characterized by spectral (IR, 1H- and 13C-NMR) data and compared to those reported in literature.
Conclusion
A simple, energy efficient, one step SDS catalyzed (0.03 mol%) greener method for the synthesis of quinoxaline derivatives underwater mediating condition has been developed. Structurally diversified α-bromoketones, commonly regarded as safer chemicals, were used as reaction partners of 1,2-diamines in water at ambient temperature. Effect of the nature and position of the substituents on both the reactants in consideration to the reaction condition was also studied. Disubstituted α-bromoketones and 2α-bromofriedelin (a representative of pentacyclic triterpenoids) also formed the corresponding quinoxalines that may serve as lead compound in near future. Except water, no other organic solvents were used. The ambient reaction conditions, comparatively lower reaction time, excellent product yields, and simple work up procedure not only make this methodology an alternative platform to the conventional acid/base catalyzed thermal processes, but also found to be significant under the umbrella of environmentally greener and safer processes that may find its place in industry. Moreover, water as a solvent used with miceller SDS has both economic and environmental advantages. As micelles of diameter of 322 nm were formed, it was anticipated that the entitled reactions were occurring inside the hydrophilic core of the micelles. Scaling up the reaction up to 5 mol scale gave good results. We believe our developed process not only satisfied the principles of green chemistry, but also can open a new way of synthesizing bioactive molecules by catalyzing SDS in water. Further explorative studies of this efficient combination to various organic syntheses is undergoing in our laboratory.
Acknowledgements
The authors are thankful to UGC, New Delhi, India for financial support to carry out the work.
References
- Dell , A. ; William , D.H. ; Morris , H.R. ; Smith , G.A. Feeney , J. ; G. C.K. Roberts J. Am. Chem. Soc . 1975 , 97 , 2497 – 2502 .
- Sato , S. ; Shiratori , O. ; Katagiri , K. J. Antibiot . 1967 , 20 , 270 – 272 .
- Bailly , C. ; Echepare , S. ; Gago , F. ; Waring , M. Anti-Cancer Drug Des . 1999 , 14 , 291 – 295 .
- Renault , J. ; Baron , M. ; Mailliet , P. Eur. J. Med. Chem . 1981 , 16 , 545 – 548 .
- Kumar , A. ; Kumar , S. ; Saxena , A. ; De , A. ; Mozumdar , S. Catal. Commun . 2008 , 9 , 778 – 784 .
- Justin Thomas , K.R. ; Marappan , V. ; Jiann , T.L. ; Chang-Hao , C. ; Yu-ai , T. Chem. Mater . 2005 , 17 , 1860 – 1866 .
- Dailey , S. ; Feast , J.W. ; Peace , R.J. ; Saga , R.C. ; Till , S. ; Wood , E.L. J. Mater. Chem . 2001 , 11 , 2238 – 2243 .
- Jonathan , L.S. ; Hiromitsu , M. ; Toshihisa , M. ; Vincent , M.L. ; Hiroyuki , F. Chem. Commun . 2002 , 3 , 862 – 863 .
- Jonathan , L.S. ; Hiromitsu , M. ; Toshihisa , M. ; Vincent , M.L. ; Hiroyuki , F. J. Am. Chem. Soc . 2002 , 124 , 13474 – 13479 .
- Peter , P.C. ; Gang , Z. ; Grace , A.M. ; Carlos , H. ; Linda , M.G.T. Org. Lett . 2004 , 6 , 333 – 335 .
- Sascha , O. ; Rudiger , F. Synlett 2004 , 15 , 1509 – 1511 .
- Kazunobu , T. ; Ryusuke , T. ; Tomohiro , O. ; Shuichi , M. Chem. Commun . 2002 , 3 , 212 – 213 .
- Hegedus , L.S. ; Marc , M.G. ; Jory , J.W. ; Joseph , P.B. J. Org. Chem . 2003 , 68 , 4179 – 4182 .
- Zhao , Z. ; Wisnoski , D.D. ; Wolkenberg , S.E. ; Leister , W.H. ; Wang , Y. ; Lindsley , C.W. Tetrahedron Lett . 2004 , 45 , 4873 – 4876 .
- More , S.V. ; Sastry , M.N.V. ; Yao , C.-F. Green Chem . 2006 , 8 , 91 – 95 .
- Antoniotti , S. ; Donach , E. Tetrahedron Lett . 2002 , 43 , 3971 – 3973 .
- Raw , S.A. ; Wilfred , C.D. ; Taylor , R.J.K. Chem. Commun . 2003 , 4 , 2286 – 2287 .
- Kim , S.Y. ; Park , K.H. ; Chung , Y.K. Chem. Commun . 2005 , 6 , 1321 – 1323 .
- Robinson , R.S. ; Taylor , R.J.K. Synlett 2005 , 16 , 1003 – 1005 .
- Cho , C.S. ; Oh , S.G. J. Mol. Catal. A: Chem . 2007 , 276 , 205 – 210 .
- Cho , C.S. ; Oh , S.G. Tetrahedran Lett . 2006 , 47 , 5633 – 5636 .
- Sylvain , A. ; Elisabet , D. Tetrahedron Lett . 2002 , 43 , 3971 – 3973 .
- Steven , A.R. ; Cecilia , D.W. ; Richard , J.K.T. Chem. Commun . 2003 , 4 , 2286 – 2887 .
- Venugopal , D. ; Subrahmanmyam , M. Catal. Commun . 2001 , 2 , 219 – 223 .
- Xekoukoulotakis , N.P. ; Hadjiantoniou , M. ; Maroulis , A.J. Tetrahedron Lett . 2000 , 41 , 10299 – 10302 .
- Zhijian , Z. ; David , D.W. ; Scoot , E.W. ; William , H.L. ; Craig , W.L. Tetrahedron Lett . 2004 , 45 , 4873 – 4876 .
- Shyamaprosad , G. ; Avijit , K.A. Tetrahedron Lett . 2002 , 43 , 8371 – 8373 .
- Zemin , W. ; Nicholas , J.E. Tetrahedron Lett . 2001 , 42 , 8115 – 8118 .
- Orazio , A.A. ; Lucia , D.C. ; Paolino , F. ; Fabio , M. ; Stefania , S. Synlett 2003 , 14 , 1183 – 1185 .
- Sanjay , K.S. ; Priya , G. ; Srinavas , D. ; Bijoy , K. Synlett 2003 14 , 2147 – 2150 .
- Das , B. ; Venkateswarlu , K. ; Suneel , K. ; Majhi , A. Tetrahedron Lett . 2007 , 48 , 5371 – 5374 .
- Wan , J.P. ; Gan , S.-F. ; Wu , J.-M. ; Pan , Y. Green Chem . 2009 , 11 , 1633 – 1637 .
- Ghosh , P. ; Mandal , A. Catal. Commun . 2011 , 12 , 744 – 747 .
- Lee , K.H. J. Nat. Prod . 2010 , 73 , 500 – 516 .
- Dwars , T. ; Schmidt , U. ; Fischer , C. ; Grassert , I. ; Kempe , R. ; Frohlich , R. ; Drauz , K. ; Oehme , G. Angew. Chem. Int. Ed . 1998 , 37 , 2851 – 2853 .
- Bahrami , K. ; Khodaei , M.M. ; Nejati , A. Green Chem . 2010 , 12 , 1237 – 1240 .
- Zheng , O. ; Zhao , J.-X. ; Fu , X.-M. Langmuir 2006 , 22 , 3528 – 3532
- Corey , E.J. ; Ursprung , J.J. J. Am. Chem. Soc . 1956 , 78 , 5041 – 5051 .
- Kane , V.V. ; Stevenson , R. J. Org. Chem . 1960 , 25 , 1394 – 1396 .
Appendix
Characterization of some representative compounds
(1) 2-phenyl quinoxaline
1H NMR (CDCl3, 300 MHz) δ 6.91–6.96 (m, 3H), 7.08–7.17 (m, 2H), 7.48–7.58 (m, 4H), 8.75 (s, 1H); 13C NMR (CDCl3, 75 MHz) δ 127.5, 129.1, 129.5, 129.6, 130.1, 136.7, 141.5, 142.2, 143.3, 151.8.
(2) 2- p -tolylquinoxaline
1H NMR (CDCl3, 300 MHz) δ 2.43 (s, 2H), 7.35 (d, 2H, J = 7.8 Hz), 7.71–7.77 (m, 2H), 8.08–8.15 (m, 4H), 9.31 (s, 1H); 13C NMR (CDCl3, 75 MHz) δ 21.43, 126.0, 127.4, 128.9, 129.3, 129.5, 129.9, 130.5, 131.3, 133.8, 140.2, 140.5, 141.2, 143.1, 151.84.
(3) 2-(4-ethylphenyl)quinoxaline
1H NMR (CDCl3, 300 MHz) δ 1.26–1.33 (m, 3H), 2.74 (q, 2H, J = 7.5 Hz), 7.35 (d, 2H, J = 8.1 Hz), 7.74 (q, 2H J = 7.5 Hz) 8.09–8.15 (m, 4H), 9.30 (s, 1H); 13C NMR (CDCl3, 75 MHz) δ 15.4, 28.8, 127.5, 128.7, 129.0, 129.3, 129.5, 130.2, 134.1, 141.3, 142.3, 143.2, 146.8, 151.8.
(4) 2-(4-bromophenyl)quinoxaline
1H NMR (CDCl3, 300 MHz) δ 7.68–7.71 (m, 2H), 7.75–7.78 (m, 2H), 8.07–8.16 (m, 4H), 9.29 (s, 1H); 13C NMR (CDCl3, 75 MHz) δ 125.0, 129.0, 129.6, 129.9, 130.5, 132.3, 135.6, 141.6, 142.2, 142.7, 150.6.
(5) 2-(4-methoxyphenyl)quinoxaline
1H NMR (CDCl3, 300 MHz) δ 3.89 (s, 3H), 7.08 (s, 2H), 7.70–7.76 (m, 2H), 8.08–8.18 (m, 4H), 9.29 (s, 1H); 13C NMR (CDCl3, 75 MHz) δ 55.5, 114.6, 129.00, 129.05, 129.2, 130.3, 141.1, 142.2, 143.1, 151.4, 161.5.
(6) 2-(4-chlorophenyl)quinoxaline
1H NMR (CDCl3, 300 MHz) δ 7.54 (d, 2H, J = 8.4 Hz), 7.78–7.94 (m, 2H), 8.15 (d, 4H, J = 8.4 Hz), 9.32 (s, 1H); 13C NMR (CDCl3, 75 MHz) δ 128.8, 129.0, 129.4, 129.6, 130.6, 135.1, 136.7, 141.4, 142.3, 142.7, 150.7.
(7) 2-(3-nitrophenyl)quinoxaline
1H NMR (CDCl3, 300 MHz) δ 7.76–7.86 (m, 3H), 8.15–8.21 (m, 2H), 8.38 (d, 1H, J = 6.3 Hz), 8.55 (d, 1H, J = 7.5 Hz), 9.11 (s, 1H), 9.39 (s, 1H); 13C NMR (CDCl3, 75 MHz) δ 122.5, 124.7, 129.2, 129.8, 130.2, 130.6, 130.9, 133.0, 138.4, 142.0, 142.2, 149.0, 149.1.
(8) 2-(3, 4-dimethoxyphenyl)quinoxaline
1H NMR (CDCl3, 300 MHz) δ 3.91 (s, 3H), 4.05 (s, 3H), 7.01 (d, 1H, J = 8.1 Hz), 7.27–7.74 (m, 3H), 7.86 (d, 1H, J = 2.1 Hz), 8.07–8.15 (m, 2H), 9.29 (s, 1H); 13C NMR (CDCl3, 75 MHz) δ 56.02, 56.08, 110.1, 111.1, 120.4, 129.0, 129.1, 129.3, 129.5, 130.2, 141.2, 142.2, 143.1, 149.7, 151.1, 151.3.
(9) 2-(3, 4-dichloroyphenyl)quinoxaline
1H NMR (CDCl3, 300 MHz) δ 7.26 (s, 1H), 7.43–7.46 (m, 1H), 7.57 (d, 1H, J = 1.8 Hz), 7.70 (d, 1H, J = 8.1 Hz), 7.80–7.83 (m, 2H), 8.14–8.19 (m, 2H), 9.20 (s, 1H); 13C NMR (CDCl3, 75 MHz) δ 127.9, 129.2, 129.6, 130.0, 130.4, 130.5, 132.9, 133.3, 135.0, 136.3, 141.3, 142.3, 145.8, 151.3.
Isolation of friedelin
Friedelin was isolated in petroleum ether by a soxhlet apparatus for 72 hours. Solvent was removed in reduced pressure. The brown gummy residue obtained was then purified over a column of silica gel (60–120 mesh). Mixtures of petroleum ether and ethyl acetate were used to run the column. Friedelin was found as white powdered material of melting point 260–262°C. 1H NMR (CDCl3, 300 MHz) δ 0.72 (s, 3H, –CH3), 0.76(s, 3H, −CH3), 0.86(s, 3H, −CH3), 0.88(s, 3H, −CH3), 0.92(s, 3H, −CH3), 1.00(s, 3H, −CH3), 1.05(s, 3H, −CH3), 1.18(s, 3H, −CH3). All other peaks are in good agreement with that reported for friedelin. 13C NMR (CDCl3, 75 MHz) δ 213.1 (C-3), 7.2 (C-23), 14.6 (C-24), 18.5 (C-25), 15.7 (C-26), 18.7 (C-27), 32.1 (C-28), 31.8 (C-29), 32.8 (C-30). All other peaks are in good agreement with that reported for friedelin.
Preperation of 2α-bromofriedelin
2α-bromofriedelin was prepared from friedelin following the process of Corey and Ursprung Citation38.
A solution of 1.28 g of friedelin in 50 ml of chloroform was treated with 1 ml of saturated solution of HBr in chloroform followed by a solution of 0.55 g of bromine in 5 ml chloroform. The depolarization was instantaneous. Chloroform was evaporated in reduced pressure and the residue was purified by column chromatography Silica 60–120 mesh). Mixtures of petroleum ether and ethyl acetate were used to run the column.
Melting point was 208–210°C (210° C as reported). 1H NMR (CDCl3, 300 MHz) δ 0.72 (s, 3H, −CH3), 0.76(s, 3H, −CH3), 0.86(s, 3H, −CH3), 0.88(s, 3H, −CH3), 0.92(s, 3H, −CH3), 1.00(s, 3H, −CH3), 1.05(s, 3H, −CH3), 1.18(s, 3H, −CH3), 3.13 (dd, 2H, J = 6.6 and 13.2 Hz, 2H −C-1), 4.40 (m, 1H, 1H −C-2). All other peaks are in good agreement with that reported for friedelin. 13C NMR (CDCl3, 75 MHz) δ 213.1 (C-3), 7.2 (C-23), 14.6 (C-24), 18.5 (C-25), 15.7 (C-26), 18.7 (C-27), 32.1 (C-28), 31.8 (C-29), 32.8 (C-30), 53.0 (C-2). All other peaks are in good agreement with that reported for friedelin.
Analytical calculation:
Friedelin quinoxaline
Melting point 248–250°C (248–151°C as reported),
1H NMR (CDCl3, 300 MHz) δ 7.43 (t, 1H, J = 7.8 Hz); 7.58 (dq, 1H, J = 2.4 and 8.1 Hz); 8.00 (dt, 1H, J = 2.7 and 7.8 Hz); 8.09 (m, 1H). 0.72 (s, 3H, −CH3), 0.76(s, 3H, −CH3), 0.86(s, 3H, −CH3), 0.88(s, 3H, −CH3), 0.92(s, 3H, −CH3), 1.00(s, 3H, −CH3), 1.05(s, 3H, −CH3), 1.15(s, 3H, −CH3).
Analytical calculation:
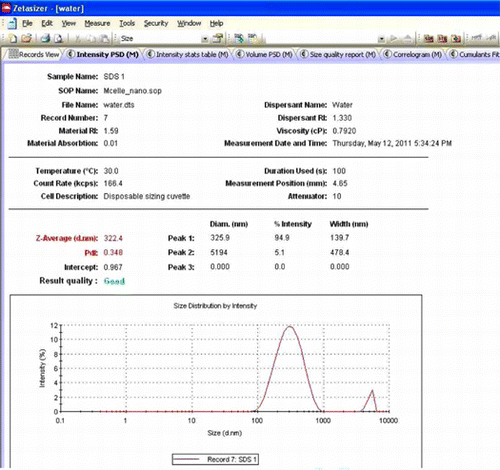