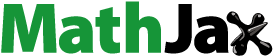
ABSTRACT
The current study aims to assess the aqueous extract of Pinus wallichiana stem for the synthesis of small spherical-shaped (10–30 nm) silver nanoparticles (AgNPs) and their in-vitro and in-vivo biomedical applications. The biosynthesized AgNPs were nonmutagenic and safe at all test doses as per Ames and acute toxicity assay (20, 40, 60, and 80 mg/kg). The percent writhing inhibitory effect generated by AgNPs was 42.51, 50.84, and 59.06 at test doses of 10, 20, and 30 mg/kg, respectively. The percent decreased in gastrointestinal tract motility observed was 41.34%, 32.69%, and 28.48% at 10, 20, and 30 mg/kg, respectively. They also showed a significant antipyretic effect after 1, 2, and 3 h in comparison to normal saline. The AgNPs of P. wallichiana showed good antibacterial activity against Acinetobacter baumannii (60% with MIC50 = 2.36 mg/ml and MBC = 5.0 mg/ml). These nanoparticles also possessed good antioxidant activity of 61.77 ± 0.828% and 70.25 ± 0.56% at 400 and 500 µg/ml, respectively and lack phytoagglutinin potential. Because of their high potency as biomedical agents, these nanoparticles can be a good alternative to the currently available drugs and approaches.
GRAPHICAL ABSTRACT
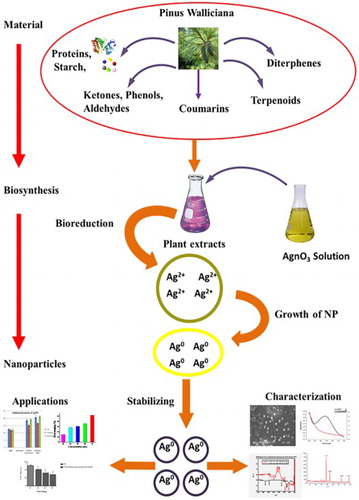
Introduction
Bionanotechnology is the integration between biotechnology and nanotechnology and has emerged as environment-friendly technology for developing biosynthetic nanomaterial. Different methods are available for the synthesis of metal nanoparticles, but “green nano-synthesis” has been reported to be an environment-friendly alternative and is preferred over the other technologies ( Citation1). Nanomaterials mostly possess unique and significantly good properties in comparison to their macroscaled counterparts ( Citation2). Metal nanoparticle synthesis is an area of interest in the current era for various applications such as optics, catalysis, electronics, and biotechnology ( Citation3, Citation4). Most stable nanoparticles are being synthesized using gold, silver, and copper metals, which are useful in different areas e.g. biological labeling, photography, photonics, surface-enhanced Raman scattering detections, and optoelectronics ( Citation5–7). Environmentally benign and commercially viable procedures are required to synthesize highly stabilized metal nanoparticles particularly silver nanoparticles (AgNPs). Although a small number of bottom-up approaches are available that involve silver salt reduction either by citrates, boron hydrides, alkyl sulfates, or alcohols are in practice, they are generally energy-intensive, costly and use environmentally dangerous chemicals. Moreover, nano-crystalline silver colloids formed by such aqua-chemical methods possess size-related issues on storage due to aggregation with time ( Citation8). Therefore, “green nano-synthesis” has been preferred over chemical methods and is considered as an environment-friendly alternative. Green syntheses use environmentally benign aqueous solvents at biological pH and offer better manipulation, control over crystal growth and their stabilization due to slower kinetics ( Citation7, Citation8).
Pinus wallichiana (Blue Pine), a coniferous evergreen tree, is native to Hindu-Kush mountains, Himalaya, Eastern Afghanistan, Karakoram, and Northern areas of Pakistan. It is a high altitude Pine, occurring from 1800 to 4000 m ( Citation9). Resin of this plant is used as a stimulant and for the cure of gonorrhea and stomachic. Externally it is used as a plaster to buboes and blisters for suppuration. The wood of P. wallichiana is used for burning sensation of body, cough, ulceration, fainting and is good for re-plantation and soil maintenance ( Citation10). Keeping in view the traditional medicinal importance of the selected plant, the current research was performed by using aqueous extract of P. wallichiana stem for the synthesis of AgNPs, their characterization, and screening for various in-vitro and in-vivo biological activities.
Material and methods
Synthesis of AgNPs
The stem of P. wallichiana, collected from Malamjabba, Khyber Pakhtunkhwa, Pakistan was identified in the Department of Botany, University of Peshawar, Pakistan. The AgNPs were fabricated through a green approach; using stem aqueous extract. Briefly, 25 g of the powdered stem was suspended in 500 ml of distilled water and boiled for 30 min to obtain aqueous extract followed by filtration. To remove cell debris, the aqueous extract was centrifuged at 4°C at 10,000 rpm, twice. The resultant supernatant was subjected to filtration through 0.2 μm filter and employed for the fabrication of AgNPs. Silver nitrate (AgNO3) was purchased from Merck and 1 mM solution was used for the synthesis of nanoparticles.
The plant’s aqueous extract was mixed with AgNO3 solution in 1:9. Shaking in the water bath for 1 h at 75°C and the change from yellow to dark brownish-black indicated the reduction of Ag+ to Ago nanoparticles. Finally, rotary evaporator was used to concentrate on the prepared nanoparticle solution under vacuum at 45°C. Synthesized AgNPs were concentrated and dried in sterile Petri plates at room temperature ( Citation7).
Characterization of synthesized AgNPs
UV-vis spectrophotometry
To confirm the synthesis of AgNPs, the colloidal silver solution was analyzed using UV-dual beam spectrophotometer (UV-1100, Shimadzu). Both the AgNPs and aqueous plant extract solutions were scanned by mixing 1 ml sample solution with 20 ml of distilled water. Aqueous plant extracts were scanned from 300 to 550 nm, while the AgNPs were scanned from 400 to 600 nm. Characteristic peak(s) were observed at point(s) of maximum absorbance (s) giving knowledge regarding the analyte, sample purity, and charge transfer ( Citation7).
X-ray diffraction
Crystalline nature of AgNPs was determined by X-ray diffraction (XRD) through JDX 3532 JEOL, Japan. The diffracted intensities were calculated from 10° to 90° of 2θ angles. A voltage of 20–40 kV was used and measurement was done at a wavelength of 1.5418 Å in θ − 2θ configurations using the Debye–Scherrer equation ( Citation11).
Scanning electron microscopy
The structure of the synthesized AgNPs was analyzed using a scanning electron microscope (SEM) (JSM5910, JEOL, Japan). Copper grid carbon was coated with a thin film of AgNPs, mixed with distilled water and allowed to dry in the presence of hot air for 5 min and then examined under an electron microscope at various magnification powers ( Citation11).
Energy dispersive X-ray detection
Elemental analysis of AgNPs was carried out with energy dispersive X-ray (EDX) detection coupled with SEM (JSM-5910 with model INCA-200), Oxford instruments, UK. The AgNPs were analyzed at sticky tape fixed on a microscopic stub of aluminum on both sides ( Citation11).
Fourier transform infra-red (FTIR) spectroscopy
The plant-derived AgNPs and aqueous extract(s) were analyzed by FTIR spectroscopy (Prestige-21, Shimadzu, Japan) from 4000 to 400 cm−1 to determine the functional groups involved in AgNP synthesis ( Citation11).
Toxicity studies
Ames assay
A commercial test kit Muta-Chromate based on validated Ames bacterial reverse-mutation test was used to perform the fluctuation test. Chemicals used were 40% w/v d-glucose, Davis–Mingioli salt (concentrated 5.5 times 2 mg/ml bromocresol purple), 0.1 mg/ml l-histidine, 0.1 mg/ml d-biotin and standard mutagens; sodium azide (NaN3) (0.5 μg/100 μl), and 2-nitro fluorine (30 μg/100 μl). The reagent mixture was prepared by mixing bromocresol purple (2.38 ml), Davis–Mingioli (21.62 ml), d-biotin (1.19 ml), d-glucose (4.75 ml), and l-histidine (0.06 ml). Sterile distilled water, test samples (extracts and NPs), reagent mixture, and standard mutagens were mixed in bottles. Two mutant strains (TA100 (Salmonella typhimurium) and TA98 (S. typhimurium)) were inoculated in nutrient broth for 18–24 h at 37°C. About 5 μl of culture broth was mixed thoroughly in bottles. The contents of each bottle were transferred to a multichannel reagent vessel and 200 μl of the mixture was transferred by a multichannel pipette in each well of 96-well micro-titre plate. To prevent evaporation from the plate, it was kept in airtight plastic bag and incubated at 37°C for 4 days ( Citation12, Citation13).
Interpretation of results: The standard and background test plates were visually observed. The partial yellow, turbid, or yellow color wells were marked as positive, purple color were marked as −ive wells, while the results of the test samples were analyzed as per the reported procedure ( Citation13).
Acute toxicity assay
Swiss Albino (Balb/c) mice of either sex and specified weight range (30 ± 5 g) were used during the experimental studies provided by Veterinary Research Institute (VRI), Peshawar, Pakistan. Animals were having free access to water and nutrients and were provided with 12 h light and dark cycle at 22 ± 2°C in accordance with Animals Scientific Procedure Act (1986) United Kingdom.
Acute toxicity of AgNPs was determined according to Khan et al. Citation2010 on Balb/c in four groups (n = 6). The P. wallichiana stem-derived AgNPs were tested at different doses; 20, 40, 60, and 80 mg/kg. The test doses were injected through intra-peritoneal (i.p) route and the animals were kept under observation for 24 h with unrestricted access to food and water. After 24 h, a number of survived and dead mice were recorded ( Citation14).
Biomedical applications
In-vitro pharmacological studies
Antibacterial activity
The antibacterial activity of synthesized AgNPs was determined against vancomycin-resistant Staphylococcus aureus (VRSA), Pseudomonas aeruginosa, Morganella morganii, Escherichia coli, S. aureus, Proteus vulgaris, and Acinetobacter baumannii. Nutrient agar and broth media were prepared, autoclaved, and incubated for 24 h at 37°C for sterility check. Uniform bacterial lawns were prepared on sterile nutrient agar plates and using 6 mm borers, wells were made. The AgNPs (100 μl) were transferred to respective wells from the stock solution of AgNPs (3 mg/ml of Dimethyl Sulfoxide (<1%)) and incubated at 37°C for 24 h. The Amoxicillin was used as a positive control and DMSO (<1%) as a negative control throughout the experiment. After incubation, zone of inhibition (mm) was measured in comparison with the positive control ( Citation15). Minimum bactericidal concentration (MBC) and minimum inhibitory concentration (MIC) were determined as per the reported procedure ( Citation16).
Antifungal activity
The antifungal potential of AgNPs was determined against Hemimycena pseudocrispula, Penicillium notatum, Aspergillus niger, A. parasiticus, Verticillium longisporum, and P. chrysogenum. Sterile Sabouraud dextrose agar (SDA) plates were used to refresh the above-mentioned fungal cultures. Stock solution (24 mg/ml) of the AgNPs was prepared in DMSO and 67 μl was transferred to test tubes containing 4 ml of the SDA media before making slants. The slants were inoculated with 5–7 days old fungal culture and incubated at 28 ± 1°C for 7 days. The DMSO (≤1%) and Miconazole were used as the negative and positive control, respectively. The percent linear growth inhibition was measured in comparison with negative control ( Citation17).
Antioxidant activity
The antioxidant activity of the AgNPs was determined according to the reported protocol ( Citation18). Different concentrations (100, 200, 300, 400, and 500 μg/ml) of the test sample were prepared. Furthermore, each concentration (1 ml) was mixed with 2 ml of freshly prepared 2,2-diphenyl-1-picryl hydrazyl (DPPH) solution followed by incubation for 10 min in darkroom. The absorbance of each sample was measured using UV-visible spectrophotometer (517 nm) for analyzing the antioxidant potential of the samples by the free radical scavenging effect of DPPH. The control used in the procedure was 1 ml methanol + 2 ml DPPH solution.
Brine shrimp lethality assay
Brine shrimp lethality bioassay ( Citation19) was used to determine the cytotoxic potential of AgNPs. Artificial seawater was prepared by mixing commercial salt with double-distilled (38 g/l) under constant aeration for 48 h. Cone-shaped vessels (1 l) filled with sterile artificial seawater were used for brine shrimp egg hatching. Active nauplii, after hatching, were collected for the assay. The stock solution of AgNPs (10 mg/ml of distilled water) was serially diluted (10, 100, and 1000 μg/ml) and 1 ml solution from each concentration was added into the test tubes containing 30 nauplii and 1 ml of seawater. The mortality rate was determined by counting the number of dead nauplii after 24 h. Standard drug (Etoposide) was used as a positive control. The mean mortality in the test and control tubes were compared to evaluate the percentage lethality. The LC50 values were determined from the best-fit line plotted concentration versus percentage.
Hemagglutination activity
Hemagglutination activity of the AgNPs was carried out against ABO blood groups according to the reported procedure ( Citation20). Different dilutions (1:2, 1:4, 1:8, and 1:16) were made from stock solution (1 mg/ml) of AgNPs in the phosphate buffer. The RBC’s suspension (2%) was made in the phosphate buffer from fresh blood, collected from a healthy volunteer. 1 ml sample + 1 ml RBC’s suspension were taken in a test tube and were incubated at 37°C for 30 min. This procedure was followed for each dilution. Rough and smooth button formation after centrifugation represented positive and negative results, respectively. The extent of deposition determined the intensity of positive result.
Anti-thrombolytic activity
The anti-thrombolytic activity of AgNPs was determined as per the reported procedure (
Citation21). From healthy human volunteers, 6 ml blood was collected and 1 ml of it was added to the pre-weighed microcentrifuge tubes followed by incubation till clotting. Clot formation is followed by serum aspiration without disturbance. Each clot containing tube was reweighed to find out the clot weight. In the next step, 20, 40, 60, 80, and 100 µl of AgNPs were added to each tube, having pre-weighed clot and were incubated for 2 h at 37°C. The controls used in the procedure were Streptokinase (positive) and distilled water (negative). The fluid obtained was removed after incubation, and clot lysis was observed by measuring the weight difference after clot disruption. The whole experiment was repeated thrice and percent clot lysis was measured.
In-vivo assays
Analgesic activity
The Balb-C mice of either sex (18–22 g) were used for determining analgesic effect using acetic acid-induced writhing ( Citation22). For the experiments, mice were divided into three groups (n = 6) receiving doses of 10, 20, and 30 mg/kg, respectively. At least, 2 h before starting the experiment, mice were withdrawn from food. The AgNPs, diclofenac sodium (positive control), and normal saline (negative control) were i.p. administered, 30 min prior to acetic acid (1%, 10 ml/kg). The acetic acid was administered through i.p. route in order to induced writhing behavior in mice. Finally, numbers of writhes were observed for 20 min; past 5 min of acetic acid induction.
Gastrointestinal tract motility (GIT) (charcoal meal protocol)
The AgNPs were analyzed for its possible effect on GIT motility ( Citation23). The selected 25–30 g Balb-c mice were distributed into five groups (n = 6). Group I was administered with normal saline (10 ml/kg) and Group II with castor oil (0.1 ml/kg), serving as a negative and positive control, respectively. The other groups (III, IV, and V) were treated with AgNPs at doses of 10, 20, and 30 mg/kg, i.p. After 15 min, the groups were treated with 0.3 ml of charcoal suspension. The mice were dissected after 30 min of treatment to observe the movement of charcoal in the small intestine for calculation of percent GIT motility.
Antipyretic activity
The antipyretic activity of AgNPs was determined by inducing pyrexia in Balb-C, weighing 25–30 g, using Brewer’s yeast (Merck, Germany) ( Citation22). The mice were having open access to water overnight but without food and were divided into four groups (n = 6); Group 1 (normal saline, 10 ml/kg), Group 2 (paracetamol, 50 mg/kg), Groups 3 and 4 (10 and 20 mg/kg, respectively). The normal body temperature of mice was noted before developing hyperpyrexia. To create hyperpyrexia, Brewer’s yeast (20%, 10 ml/kg b.w) was injected in the back of mice, below the nape of the neck. After 18 h, changes were observed in the rectal temperature and mice showing a rise in rectal temperature (0.3–0.5°C) were selected for dosing. The animal’s rectal temperature was recorded at an interval of 30 min for 3 h after the AgNP injection. The temperature was recorded for possible antipyretic activity.
Statistical analysis
One-way ANOVA followed by Dunnett’s post hoc analysis was used for data analysis. P < .05 was considered as significant.
Results
Characterization of AgNPs
AgNP formation was confirmed by the appearance of brown color after 30 min of heating at 55°C and characterized using various techniques.
Ultra violet -visible spectroscopy
The metallic nanoparticles are excited with free electrons which give resultant surface plasmon resonance (SPR) absorption bands on interaction with a specific wavelength. The UV-vis spectra of the P. wallichiana stem extract and the derived AgNPs are shown in . The corresponding SPR peaks of the spectral patterns at 424 nm confirmed the biogenic synthesis of spherical AgNPs. The present results showed the increased intensity of plasmon bands, which then slowly shifted lower that might be due to particle size and shape, as reported ( Citation24, Citation25). Njagi et al. explained the spectrum absorption of colloidal silver observed between 400 and 450 nm due to SPR ( Citation26). The present result findings are in accordance with Anandalalashmi and Ahmad et al for AgNPs from Pedalium murex leaf and Azadirachta indica ( Citation27, Citation28).
X-ray diffraction
The XRD analysis was carried out for the determination of crystal size of AgNPs. Significant peaks were observed from XRD results and the Debye–Scherer’s equation was used to calculate the crystal size of AgNPs. Diffraction peaks were observed at 29.65°, 38.2°, 44.35°, 64.5°, and 77.4° corresponding to 111, 200, 220, and 310 face centered cubic (FCC) planes, respectively, as shown in (a). These peaks were absent in stem powder and aqueous extract indicating their non-crystalline nature ((b,c)), respectively. The crystal size observed for AgNPs in the current study was 305.64 Å ().
Table 1. Crystalline size of P. wallichiana AgNPs using the Debye–Scherer’s equation.
The XRD analysis showed variation in corresponding nanoparticles with the index of FCC and compared to the plant sample, no such peaks were detected as supported by Huang et al. ( Citation29) who reported intense peaks at FCC planes in nanoparticles of Cacumen platycladi leave extract. Similarly, Geetha et al. studied the XRD profile of flower aqueous extract of Carapa guianensis-derived AgNPs and observed peaks at 111, 200, 220, and 311 FCC plane, making it in line with our study ( Citation30).
Scanning electron microscopy
The SEM analysis of AgNPs specified the presence of small spherical-shaped particles with a size range of 10–30 nm, as shown in (a–f). Many plants-synthesized various secondary metabolites can be utilized for the synthesis of metal nanoparticles. Similar results were reported by Ankanna et al. synthesizing AgNPs with dispersed spherical shapes from the aqueous extract of Boswellia Ovaliafoliolata ( Citation31). Many other studies have reported a variety of plants that have been used for the synthesis of nanoparticles with spherical shapes that exhibit various bioactivities ( Citation32, Citation33).
EDX detection
The P. wallichiana stem extracts analyzed with EDX showed percent elemental analysis as carbon (60.67), oxygen (39.05), magnesium (0.06), silicon (0.05), potassium (0.06), and calcium (0.10) (). The EDX of biosynthesized AgNPs was C (8.81%), O (64.67%), and K (2.26%), as shown in . As the synthesis of AgNPs was accomplished with AgNO3, additional peaks were observed for Ag (17.92%) at 3 keV, strongly confirming the reduction of AgNO3 to AgNPs.
Our results are supported by different reported studies with sharp peaks at 3 keV in EDX ( Citation34–37). As these AgNPs were synthesized with different plant materials, Acacia nilotica, Citrus sinensis, Cosmos Sulphureus, and Dracocephalum moldavica, still the resultant Ag availability was determined at around 3 keV through EDX analysis.
FTIR spectroscopy
The FTIR spectra of P. wallichiana stem aqueous extract showed various stretches of bonds with different peaks; 3315.63 cm−1 confirmed the presence of OH group of alcohol/phenol stretch, 2920.23 cm−1 C–H methylene asymmetric stretching and 1616.35 cm−1 represents the C=C stretch of alkenes. In the case of AgNPs the OH group peak at 3315 cm−1 disappeared confirming the reduction reaction and synthesis of AgNPs (). The corresponding peak at 1288.92 cm−1 became wide in the case of AgNPs representing the –C–O group of polyols; polysaccharides, terpenoids, and flavones, involved in the capping of synthesized AgNPs.
The FTIR is an advanced tool for studying the relationship of functional groups within biomolecules and metal particles, more precisely the surface composition of these compounds for capping and efficient stabilizations ( Citation38). Devaraj et al. characterized AgNPs of C. guianensis leaves and the FTIR spectra obtained the presented strong absorption band similar to our results ( Citation39). The present study is also supported by Banerjee et al. characterizing AgNPs of Musa balbisiana, Ocimum tenuiflorum, and A. indica leaves, whereas Ahmed and Ikram (Citation2015) and Benakashani et al. characterized AgNPs of Terminalia arjuna and Capparis spinosa leave extracts, respectively ( Citation40–42).
Toxicity studies
Mutagenicity test (Ames assay)
The mutagenicity of AgNPs was tested against S. typhimurium TA98 and TA100 and the results are presented in . For the S. typhimurium (TA-98), the background was nontoxic and nonmutagenic (5/96), the standard was highly mutagenic (85/96), while the test sample was nontoxic and nonmutagenic (4/96) in terms of positive wells to the bacterial strain. For S. typhimurium (TA-100), the background was nontoxic and nonmutagenic (10/96), the standard was highly mutagenic (87/96) and the test sample was nontoxic and nonmutagenic (0/96).
Table 2. Mutagenic activity of AgNPs.
Ames test is a bioassay for evaluating the DNA aggregating or mutating potential (carcinogenic) of nanoparticles and other compounds by utilizing various bacterial strains. Li et al. evaluated the AgNP genotoxicity using Salmonella strains and observed that the treatment with the AgNPs does not change mutation frequency ( Citation43). Kim et al. assessed the biocompatibility of AgNPs and the effect was concentration dependent in animal models ( Citation44). Heshmati et al. examined the mutagenicity and inhibitory effects of nanoparticles and reported that TA100, TA90, and YG1029S were mutated, whereas in other strains the mutation had reduced ( Citation45).
Acute toxicity assay
In this study, P. wallichiana stem-derived AgNPs were screened for possible in-vivo biological efficacy at different test doses; 20, 40, 60, and 80 mg/kg as per OECD guidelines. The synthesized AgNPs were found safe at all test doses and no lethality was observed ().
The administration of herbal products without adequate scientific studies on safely raised toxicity concerns ( Citation46). The potential health risks caused by characteristic antagonistic plant extracts or chemical compounds in humans are measured using animal toxicity studies ( Citation47, Citation48). The toxicity test is used mostly to evaluate human toxicity risks and alterations in hematological parameters when data are interpreted from animal studies ( Citation49). A study was conducted on the acute toxicity on the eye and oral irritation, corrosion, and dermal toxicity of mice exposed to synthesized colloidal AgNPs. The AgNPs at 5000 mg/kg for 72 h exposure showed no acute toxicity and mortality. The body weights of treated and untreated mice were having no significant changes. However, in the first 24 h a transient eye irritation was observed. It was concluded that the exposure of colloidal AgNPs was relatively safe and recommended for short periods of time ( Citation50). For the application of AgNPs, the in-depth knowledge of biological system is necessary, as AgNPs alone may not damage the system, but ligand binding AgNPs result in different toxicity patterns. Zhao and Wang investigated acute and chronic toxicities of AgNPs against Daphnia magna. The results showed that at 500 µg, AgNPs had no effect on D. magna for 48 h, where the accumulation of AgNPs was observed (22.9 mg) in dry weight. However, the free Ag+ from AgNO3 showed 50% lethality after 48 h. It was concluded that acute toxicity effect was because of Ag+ not due to AgNPs ( Citation51).
Another study assessed the biokinetics and acute toxicity of AgNPs at concentrations of 7.5, 30, or 120 mg/kg in mice. The toxic effects were determined via general behavior, histopathological observation, and serum biochemical parameters. Tissue distribution and biokinetics of AgNPs were evaluated in male and female mice at 120 mg/kg. After two weeks, no obvious acute toxicity was observed and only elevated level of AgNPs was observed in some organs of both genders. These results showed that AgNPs could be extensively distributed to several body tissues (mainly in the liver and spleen) and seem to be gender-associated ( Citation52).
In vitro pharmacological studies
Analgesic activity
The P. wallichiana stem-derived AgNPs showed a significant decrease in the number of writhes as determined via two-way ANOVA and Dunnett’s post hoc analysis after different intervals (30, 60, and 90 min). The values are presented as mean ± SEM and the results were considered significant at P < 0.05. In the saline treated group, mean writhing was 74.33 ± 8.95. The percent inhibitory effect of writhing produced by different test doses of AgNPs was 42.51 (10 mg/kg), 50.84 (20 mg/kg), and 59.06 (30 mg/kg) ().
Gastrointestinal tract motility
The AgNPs were screened for its possible effect on GIT motility. The percent reduction in GIT motility was observed in a dose-dependent manner, as shown in . The percent decrease in GIT motility detected at different test doses was 41.34 (10 mg/kg), 32.69 (20 mg/kg), and 28.48 (30 mg/kg).
The uptake of nanoparticles in GIT is highly affected by its size. Furthermore, the toxicology of nanoparticles in GIT and its efficacy in other organs has been reported ( Citation53, Citation54). Ferula assafoetida belongs to genus Ferula and is illustrious for its antispasmodic activity. The crude extract interaction with M3 receptor (muscarinic) of the small intestine may cause relaxation of the intestine, where acetylcholine can act and M3 receptor activation will lead to increase contraction ( Citation55, Citation56).
Antipyretic activity
The AgNPs showed significant antipyretic effect after 1, 2, and 3 h in comparison to negative control (normal saline), as presented in . Paracetamol (50 mg/kg) showed a temperature reduction with a mean value of 36.74 ± 0.10 in comparison to the normal saline. The AgNP reduction in temperature observed was 36.40 ± 3.11 at 10 mg/kg and 36.56 ± 0.36 at 20 mg/kg body weight of the animal.
Brewer’s yeast-induced pyrexia model is used to induce the hyperthermia-like condition. It causes the release of prostaglandins that is considered as an important mediator of pyrexia. This mediator affects thermoregulatory center in body and causes fever ( Citation57, Citation58). Therefore, antipyretic agents are needed to reduce this increase in temperature. A variety of antipyretics inhibit the cyclooxygenase pathway within the hypothalamus and control temperature or they may decrease the level of prostaglandin E2 ( Citation59).
Antibacterial activity
The AgNPs showed good activity against A. baumannii (60% with MIC50 = 2.36 mg/ml and MBC = 5.0 mg/ml), while the presented moderate activity against VRSA (56% with MIC50 = 2.92 mg/ml and MBC = 7.0 mg/ml), E. coli (54% with MIC50 = 2.52 mg/ml and MBC = 6.44 mg/ml), P. vulgaris (53% with MIC50 = 2.76 mg/ml, MBC = 4.24 mg/ml), S. aureus (50% with MIC50 = 5.0 mg/ml and MBC = 8.96 mg/ml), M. morganii (45.5% with MIC50 = 3.12 mg/ml and MBC = 6.16 mg/ml), and P. aeruginosa (45.1% with MIC50 = 3.76 mg/ml and MBC = 6.32 mg/ml) (, ).
Table 3. MIC50 (mg/ml) and MBC50 (mg/ml) of AgNPs of P. wallichiana.
The results of the present study demonstrate the ability of AgNPs to combat against pathogenic bacteria. These AgNPs could be further exploited to serve as a starting material for antimicrobial drug development. These nanoparticles possess large surface to volume area, which makes them highly reactive in bacterial growth inhibition. Cell penetration by interaction with sulfur and phosphorous-containing compounds may cause damage to compounds like protein and DNA ( Citation59). The AgNP causes cell wall distraction of microbes by “pits” formation ( Citation60).
Another study described that maximum inhibition zone was observed for B. ciliata AgNP compared to their crude extract ( Citation61). One of the possible mechanisms of antibacterial activity of metallic nanoparticles is weakening DNA replication and protein inactivation ( Citation62).
Antifungal activity
The AgNPs exhibited moderate antifungal activity against P. chrysogenum (50%), P. notatum (45%), and A. niger (40%), while it presented low activity against H. pseudocrispula (30%), V. longisporum (21%), and A. parasiticus (13%), as presented in .
The antifungal effect was reported in terms of membrane disruption causing fungal cells death ( Citation63). A study reported that nanoparticles and Ag+ had a substantial effect on the colony formation of plant pathogenic fungi; Bipolaris sorokiniana and Magnaporthe grisea. The EC50 of silver compounds, which effectively inhibit colony formation, was higher for B. sorokiniana ( Citation64).
Antioxidant activity
The antioxidant activity of the AgNPs of P. wallichiana is given in . It was observed that the test sample possessed good antioxidant activity of 61.77 ± 0.828 and 70.25 ± 0.56% at 400 and 500 µg/ml, respectively. The moderate activity of 54.19 ± 0.485 and 46.55 ± 0.55, respectively, was observed at 300 and 200 µg/ml, while low activity (38.59 ± 0.638) was observed at 100 µg/ml. The EC50 of AgNPs recorded in the current study was 303.03 µg/ml.
Table 4. Antioxidant activity of the stem-derived AgNPs of P. wallichiana.
A study reported that instead of metal oxides, the silver and gold nanoparticles are strong superoxide, nitric oxide, DPPH, and hydroxyl radical scavengers ( Citation65). Dalbergia spinosa-based AgNPs possessed strong scavenging capacities in comparison to that of control, emphasizing its potential application in pharmaceuticals ( Citation66).
Uddin et al. assessed the DPPH% scavenging activity of B. ciliata methanolic extract and AgNPs and observed greater antioxidant of AgNPs than the crude extract. It has been reported that the AgNPs of Iresine herbstii possessed higher antioxidant activity ( Citation67, Citation68).
Hamagglutination activity
The result of hamagglutination activity of AgNPs at all test dilutions; 1:2, 1:4, 1:8, and 1:16 was negative as indicated by smooth button formation. This shows that stem-derived AgNPs of P. wallichiana lack phytoagglutinin potential. The results of the current study are supported by the hemagglutination activity of D. mucronata AgNPs and extracts which showed no hemagglutination ( Citation7).
Brine shrimp lethality assay
The AgNPs showed 53.33%, 36.66%, and 30% mortality at 1000, 100, and 10 μg/ml, respectively, with LD50 value of 717.829, as shown in . Brine shrimp cytotoxic activities can help to define different pharmacological properties of any plant extract ( Citation69). Cytotoxic properties of nanoparticles towards shrimp’s larvae can be an indication of its anticancer potential and could be an alternate source of anticancer medications ( Citation70). Artemia-based toxicity assays of nanoparticles are cheap, easily available, and reliable and are thus an important answer to routine needs of toxicity screening, for industrial monitoring requirements or for regulatory purposes ( Citation71).
Anti-thrombolytic activity
The percent clot lysis by AgNPs at 100, 80, 60, 40, and 20 µg/ml was 26.33, 21.45, 16.66, 9.45, and 4.65, respectively. The percent activity of Streptokinase was 60.73%, while that of distilled water was 3.39%, as shown in . The AgNPs possess higher thrombolytic potential than crude extract. The AgNPs and crude extract of leaves showed 25.8% and 15.9% thrombolytic activity at 100 μg/ml, respectively ( Citation7).
Table 5. Anti-thrombolytic activity of AgNPs of P. wallichiana.
Thrombus formation in the blood vessels causes thrombotic diseases like myocardial or cerebral infarction. Different agents used for dissolving the clots in blood vessels can have some serious and lethal consequences. Since ancient times, several herbal products have been used for the treatment of several diseases, however in some cases, toxicity has been observed ( Citation72). Hence different agents like nanoparticles could be one of the potential sources for the treatment of thrombotic diseases.
Conclusion
The AgNPs from the aqueous extract of P. wallichiana stem, characterized by standard procedures, were nonmutagenic and safe at all test doses as per Ames and acute toxicity assay. The AgNPs showed significant antipyretic effect after 1, 2, and 3 h in comparison to normal saline. The AgNPs of P. wallichiana showed good antibacterial activity against A. baumannii (60% with MIC50 = 2.36 mg/ml and MBC = 5.0 mg/ml). The AgNPs also possessed good antioxidant activity of 61.77 ± 0.828% and 70.25 ± 0.56% at 400 and 500 µg/ml, respectively and no hemagglutination activity was observed.
Disclosure statement
No potential conflict of interest was reported by the author(s).
Notes on contributors
Ms. Nazish Khan did her Bachelors in Biotechnology from University of Peshawar and then her PhD in the same University. She worked on the characterization of some medicinally important plants of the Swat region, the region she belongs to and the work of this article is part of her PhD work.
Dr. Ibrar Khan did his Bachelors and PhD in Biotechnology from Centre of Biotechnology and Microbiology, University of Peshawar, Pakistan in 2007 and 2011, respectively. He started his career as Lecturer in 2010 and now is working as an Associate Professor in the same Centre. He has so far earned about Rs. 9 million research grants from various funding agencies and has many publications in the journals of repute.
Dr. Akhtar Nadhman did his Bachelors from Centre of Biotechnology and Microbiology, University of Peshawar in 2007 and PhD from Quaid e Azam University Islamabad, Pakistan and Harvard University in Biotechnology 2014. He has published many research articles in the field of nanotechnology in prestigious journals. He is currently the Director of Institute of Integrative Biosciences, CECOS University, Peshawar Pakistan.
Dr. Sadiq Azam did his Bachelors and PhD in Biotechnology from Centre of Biotechnology and Microbiology, University of Peshawar, Pakistan in 2007 and 2011, respectively. He started his career as Lecturer in 2012 and now is working as an Assistant Professor in the same Centre. He has so far earned about Rs. 8 million research grants from various funding agencies and has many publications in the journals of repute.
Mr. Inam Ullah is an M. Phil leading to PhD scholar at the Centre of Biotechnology and Microbiology, University of Peshawar, Pakistan. He has published about five research articles so far, and has the helped in in vitro experiments in the current work.
Mr. Farhan Ahmad did his Bachelors in Biotechnology from Centre of Biotechnology and Microbiology, University of Peshawar, Pakistan in 2007 and is in the last stage of completion of his PhD from Institute of Biotechnology and Genetic Engineering, Agriculture University, Peshawar, Pakistan. He started his career as Lecturer in 2014 at the Department of Biotechnology, Bacha Khan University, Charsadda, Pakistan and is still serving in the same university.
Dr. Hamid Ali Khan did his Bachelors in Biotechnology from Department of Biotechnology, Malakand University, Pakistan in 2007 and his PhD from China. He is currently working as an Assistant Professor at the Institute of Biological Sciences, Sarhad University of Science & IT, Peshawar, KP, Pakistan
Additional information
Funding
References
- Rai, M.; Yadav, A.; Gade, A. Biotechnol. Adv. 2009, 27, 76–83. doi: 10.1016/j.biotechadv.2008.09.002
- Li, L.-S.; Walda, J.; Manna, L.; Alivisatos, A.P. Nano Lett. 2002, 2, 557–560. doi: 10.1021/nl0255146
- Hussain, I.; Brust, M.; Papworth, A.J.; Cooper, A.I. Langmuir. 2003, 19, 4831–4835. doi: 10.1021/la020710d
- Albrecht, M.A.; Evans, C.W.; Raston, C.L. Green Chem. 2006, 8, 417–432. doi: 10.1039/b517131h
- Smith, A.; Duan, H.; Rhyner, M.; Ruan, G.; Nie, S. Phys. Chem. Chem. Phys. 2006, 8, 3895. doi: 10.1039/b606572b
- Narayanan, R. J. Phys. Chem. 2005, 109, 12663–12676. doi: 10.1021/jp051066p
- Shah, A.; Lutfullah, G.; Ahmad, K.; Khalil, A.T.; Maaza, M. Green Chem. Lett. Rev. 2018, 11, 318–333. doi: 10.1080/17518253.2018.1502365
- Lee, K.J.; Jun, B.H.; Choi, J.; Lee, Y.I.; Joung, J.; Oh, Y.S. Nanotechnology 2007, 18, 335601. doi: 10.1088/0957-4484/18/33/335601
- Ghimire, B.; Mainali, K.P.; Lekhak, H.D.; Chaudhary, R.P.; Ghimeray, A.K. Himalayan J. Sci. 2011, 6, 19–26. doi: 10.3126/hjs.v6i8.1798
- Maimoona, A.; Naeem, I.; Saddiqe, Z.; Ali, N.; Ahmed, G.; Shah, I. J. Med. Plants Res. 2011, 5, 2724–2728.
- Ahmad, B.; Shireen, F.; Bashir, S.; Khan, I.; Azam, S. IET Nanobiotechnol. 2016, 10, 281–287. doi: 10.1049/iet-nbt.2015.0053
- Razak, M.F.A.; Aidoo, K.E. Asian J. Pharm. Clin. Res. 2011, 4, 1256–1267.
- Gilbert, R. Mutation Res./Environ. Mutagen. Relat. Subj. 1980, 74, 283–289.
- Khan, H.; Saeed, M.; Khan, M.A.; Dar, A.; Khan, I. J. Ethnopharmacol. 2010, 127, 521–527. doi: 10.1016/j.jep.2009.10.003
- Ahmad, B.; Bashir, S.; Azam, S.; Ali, N. J. Med. Plants Res. 2011, 5, 3380–3386.
- Banso, A. J. Med. Plants Res. 2009, 3, 082–085.
- Ahmad, B.; Ali, N.; Bashir, S.; Choudhary, M.; Azam, S.; Khan, I. Afr. J. Biotechnol. 2009, 8, 5084–5087.
- Kanatt, S.R.; Chander, R.; Sharma, A. Food Chem. 2007, 100, 451–458. doi: 10.1016/j.foodchem.2005.09.066
- Krishnaraju, A.V.; Rao, T.V.; Sundararaju, D.; Vanisree, M.; Tsay, H.-S.; Subbaraju, G.V. Int. J. Appl. Sci. Eng. 2006, 4, 115–125.
- Kaya, O.; Akçam, F.Z.; Yayli, G. Turk. J. Med. Sci. 2012, 42, 145–148.
- Thirunavukarasu, T.; Lakshmi, K.S.; Tamilarasan, M.; Sivamani, S.; Sangeetha, D.; Rajesh, T. Int. J. Phytomedicine. 2014, 6, 109–114.
- Trongsakul, S.; Panthong, A.; Kanjanapothi, D.; Taesotikul, T. J. Ethnopharmacol. 2003, 85, 221–225. doi: 10.1016/S0378-8741(03)00020-5
- Al-Qarawi, A.; Ali, B.; Al-Mougy, S.; Mousa, H. Food Chem. Toxicol. 2003, 41, 37–39. doi: 10.1016/S0278-6915(02)00203-X
- Kelly, K.L.; Coronado, E.; Zhao, L.L.; Schatz, G.C. J. Phys. Chem. B. 2003, 107, 668–677. doi: 10.1021/jp026731y
- Lee, K.-S.; El-Sayed, M.A. J. Phys. Chem. B. 2006, 110, 19220–19225. doi: 10.1021/jp062536y
- Njagi, E.C.; Huang, H.; Stafford, L.; Genuino, H.; Galindo, H.M.; Collins, J.B.; Hoag, G.E.; Suib, S.L. Langmuir. 2010, 27, 264–271. doi: 10.1021/la103190n
- Anandalakshmi, K.; Venugobal, J.; Ramasamy, V. Appl. Nanosci. 2016, 6, 399–408. doi: 10.1007/s13204-015-0449-z
- Ahmed, S.; Ahmad, M.; Swami, B.L.; Ikram, S. J. Radiat. Res. Appl. Sci. 2016, 9, 1–7. doi: 10.1016/j.jrras.2015.06.006
- Huang, J.; Zhan, G.; Zheng, B.; Sun, D.; Lu, F.; Lin, Y.; Chen, H.; Zheng, Z.; Zheng, Y.; Li, Q. Ind. Eng. Chem. Res. 2011, 50, 9095–9106. doi: 10.1021/ie200858y
- Geetha, R.; Ashokkumar, T.; Tamilselvan, S.; Govindaraju, K.; Sadiq, M.; Singaravelu, G. Cancer. Nanotechnol. 2013, 4, 91–98. doi: 10.1007/s12645-013-0040-9
- Ankanna, S.; Prasad, TNVKV; Elumalai, E.; Savithramma, N. Dig. J. Nanomater. Biostruct. 2010, 5, 369–372.
- Jameel, Z.N. Energy Procedia. 2017, 119, 236–241. doi: 10.1016/j.egypro.2017.07.075
- Priya, R.S.; Geetha, D.; Ramesh, P. Ecotoxicol. Environ. Saf. 2016, 134, 308–318. doi: 10.1016/j.ecoenv.2015.07.037
- Rachel, G.A. Proteus. 2014, 4, 18.
- Awad, M.A.; Hendi, A.A.; Ortashi, K.M.; Elradi, D.F.; Eisa, N.E.; Al-lahieb, L.A.; Al-Otiby, S.M.; Merghani, N.M.; Awad, A.A. Int. J. Phys. Sci. 2014, 9, 34–40. doi: 10.5897/IJPS2013.4080
- Malaka, R.; Hema, J.A.; Muthukumarasamy, N.P.; Sambandam, A.; Subramanian, S.; Sevanan, M. Nano. Biomed. Eng. 2015, 7, 160–168.
- Haghighi Pak, Z.; Abbaspour, H.; Karimi, N.; Fattahi, A. Appl. Sci. 2016, 6, 69. doi: 10.3390/app6030069
- Padalia, H.; Moteriya, P.; Chanda, S. Arabian J. Chem. 2015, 8, 732–741. doi: 10.1016/j.arabjc.2014.11.015
- Devaraj, P.; Kumari, P.; Aarti, C.; Renganathan, A. J. Nanotechnol.. 2013, 2013, 1–5. doi: 10.1155/2013/598328
- Banerjee, P.; Satapathy, M.; Mukhopahayay, A.; Das, P. Bioresources Bioprocess. 2014, 1, 3. doi: 10.1186/s40643-014-0003-y
- Ahmed, S.; Ikram, S. J. Nanomed. Nanotechnol. 2015, 6, 2–6.
- Benakashani, F.; Allafchian, A.; Jalali, S. Karbala Int. J. Mod. Sci. 2016, 2, 251–258. doi: 10.1016/j.kijoms.2016.08.004
- Li, Y.; Chen, D.H.; Yan, J.; Chen, Y.; Mittelstaedt, R.A.; Zhang, Y.; Biris, A.S.; Heflich, R.H.; Chen, T. Mutation Res./Genet. Toxicol. Environ. Mutagen. 2012, 745, 4–10. doi: 10.1016/j.mrgentox.2011.11.010
- Kim, J.S.; Sung, J.H.; Ji, J.H.; Song, K.S.; Lee, J.H.; Kang, C.S.; Yu, I.J. Saf. Health. Work. 2011, 2, 34–38. doi: 10.5491/SHAW.2011.2.1.34
- Heshmati, M.; ArbabiBidgoli, S.; Khoei, S.; Rezayat, S.M.; Parivar, K. Iran. J. Pharm. Res.: IJPR. 2015, 14, 1171.
- Saad, B.; Azaizeh, H.; Abu-Hijleh, G.; Said, O. Evid. Based Complement. Alternat. Med. 2006, 3, 433–439. doi: 10.1093/ecam/nel058
- Oyedemi, S.; Yakubu, M.; Afolayan, A. Hum. Exp. Toxicol. 2010, 29, 377–384. doi: 10.1177/0960327110363864
- Klaassen, C.D.; Watkins, J.B. Casarett and Doull’s Toxicology: The Basic Science of Poisons; McGraw-Hill: New York, 1996.
- Olson, H.; Betton, G.; Robinson, D.; Thomas, K.; Monro, A.; Kolaja, G.; Lilly, P.; Sanders, J.; Sipes, G.; Bracken, W. Regul. Toxicol. Pharmacol. 2000, 32, 56–67. doi: 10.1006/rtph.2000.1399
- Maneewattanapinyo, P.; Banlunara, W.; Thammacharoen, C.; Ekgasit, S.; Kaewamatawong, T. J. Vet. Med. Sci. 2011, 73, 1417–1423. doi: 10.1292/jvms.11-0038
- Zhao, C.M.; Wang, W.X. Environ. Toxicol. Chem. 2011, 30, 885–892. doi: 10.1002/etc.451
- Xue, Y.; Zhang, S.; Huang, Y.; Zhang, T.; Liu, X.; Hu, Y.; Zhang, Z.; Tang, M. J. Appl. Toxicol. 2012, 32, 890–899. doi: 10.1002/jat.2742
- Rosenkranz, P.; Chaudhry, Q.; Stone, V.; Fernandes, T.F. Environ. Toxicol. Chem. 2009, 28, 2142–2149. doi: 10.1897/08-559.1
- Arora, S.; Rajwade, J.M.; Paknikar, K.M. Toxicol. Appl. Pharmacol. 2012, 258, 151–165. doi: 10.1016/j.taap.2011.11.010
- Fatehi, M.; Farifteh, F.; Fatehi-Hassanabad, Z. J. Ethnopharmacol. 2004, 91, 321–324. doi: 10.1016/j.jep.2004.01.002
- Nemeth, P.; Ort, C.; Wood, J.D. J. Physiol. 1984, 355, 411–425. doi: 10.1113/jphysiol.1984.sp015427
- Ranels, H.J.; Griffin, J.D. Brain Res. 2003, 964, 42–50. doi: 10.1016/S0006-8993(02)04063-5
- Hullatti, K.; Sharada, M. Phcog Mag. 2007, 3, 173.
- Aronoff, D.M.; Neilson, E.G. Am. J. Med. 2001, 111, 304–315. doi: 10.1016/S0002-9343(01)00834-8
- Sondi, I.; Salopek-Sondi, B. J. Colloid Interface Sci. 2004, 275, 177–182. doi: 10.1016/j.jcis.2004.02.012
- Phull, A.-R.; Abbas, Q.; Ali, A.; Raza, H.; Zia, M.; Haq, I.-U. Future J. Pharm. Sci. 2016, 2, 31–36. doi: 10.1016/j.fjps.2016.03.001
- Feng, Q.L.; Wu, J.; Chen, G.; Cui, F.; Kim, T.; Kim, J. J. Biomed. Mater. Res. 2000, 52, 662–668. doi: 10.1002/1097-4636(20001215)52:4<662::AID-JBM10>3.0.CO;2-3
- Kim, K.-J.; Sung, W.S.; Suh, B.K.; Moon, S.-K.; Choi, J.-S.; Kim, J.G.; Lee, D.G. Biometals. 2009, 22, 235–242. doi: 10.1007/s10534-008-9159-2
- Jo, Y.-K.; Kim, B.H.; Jung, G. Plant Dis. 2009, 93, 1037–1043. doi: 10.1094/PDIS-93-10-1037
- Ramamurthy, C.; Padma, M.; Mareeswaran, R.; Suyavaran, A.; Kumar, M.S.; Premkumar, K.; Thirunavukkarasu, C. Colloids Surf. B. 2013, 102, 808–815. doi: 10.1016/j.colsurfb.2012.09.025
- Muniyappan, N.; Nagarajan, N. Process Biochem. 2014, 49, 1054–1061. doi: 10.1016/j.procbio.2014.03.015
- Uddin, G.; Rauf, A.; Arfan, M.; Ali, M.; Qaisar, M.; Saadiq, M.; Atif, M. Middle-East J. Sci. Res. 2012, 11, 1140–1142.
- Dipankar, C.; Murugan, S. Colloids Surf. B. 2012, 98, 112–119. doi: 10.1016/j.colsurfb.2012.04.006
- Hatano, T.; Edamatsu, R.; Hiramatsu, M.; Mori, A.; Fujita, Y.; Yasuhara, T.; Yoshida, T.; Okuda, T. Chem. Pharm. Bull. 1989, 37, 2016–2021. doi: 10.1248/cpb.37.2016
- Ghareeb, M.A.; Hussein, A.; Hassan, M.; Laila, A.; Mona, A.; Amal, M. Int. J. Phytopharmacology. 2014, 5, 143–157.
- Rajabi, S.; Ramazani, A.; Hamidi, M.; Naji, T. DARU J. Pharm. Sci. 2015, 23, 20. doi: 10.1186/s40199-015-0105-x
- Rahman, M.A.; Sultana, R.; Emran, T.B.; Islam, M.S.; Rahman, M.A.; Chakma, J.S.; Rashid, H.-U.; Hasan, C.M.M. BMC Complement. Altern. Med. 2013, 13, 25. doi: 10.1186/1472-6882-13-25