ABSTRACT
In this paper, we focused on the green synthesis of titanium dioxide (TiO2) nanoparticles via an ecofriendly process using Citrus limon extract. Several analytical techniques were used to characterize the prepared samples: X-ray diffraction (XRD), infrared spectroscopy, Raman spectroscopy, Scanning Electron Microscopy (SEM) and X-ray photoelectrons spectroscopy (XPS). XRD diffractograms revealed an anatase crystal structure of TiO2. SEM analysis showed the presence of particles with nanometric sizes, and the Fourier transform infrared (FTIR) spectra confirmed the presence of the synthesized nanoparticles. The antibacterial activity of the green prepared TiO2 nanoparticles was assessed. Additionally, the antibacterial efficiency of the green prepared TiO2 nanoparticles was compared with TiO2 nanoparticles prepared via a chemical process. The results prove the efficacy of this ecofriendly process. This method can be used for the rapid and environmentally friendly biosynthesis of stable titanium oxide nanoparticles. This work is a contribution to the valorization of bio-sources for the synthesis of titanium dioxide crystals using Citrus limon fruit extract and tetrabutile titanate as a precursor.
GRAPHICAL ABSTRACT
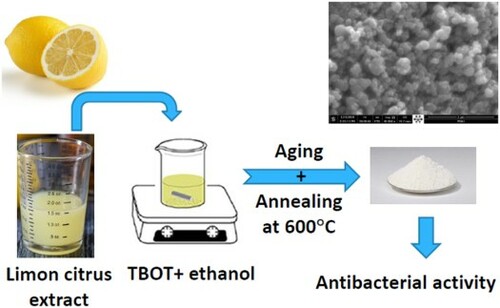
1. Introduction
Over the past three decades, metal oxide-based nanomaterials have been considered as a vital and widespread area of research due to their unique and tunable physico-chemical characteristics that make them useful for various applications (Citation1–5). Of them, TiO2-based nanomaterials have aroused substantial attention because of their easy synthesis, nontoxicity and biocompatibility, which explains their use in countless applications covering photodegradation of pollutants, paints, pharmaceuticals, personal care products, and food additive, among others (Citation6). Particularly, TiO2 plays an essential role in modern pharmaceutics, as it is often applied as pigment (E171), as coating to preserve sensitive active pharmaceutical ingredients from UV or visible light damage, and in packaging to prevent medicines from moisture, heat and light.
Typically, two general approaches are applied for the synthesis of TiO2 structures with different phases, shapes and dimensions: top-down, which is a subtractive procedure starting from bulk materials to obtain nanomaterials, and bottom-up, which consists of the assembly of precursor molecules to grow nanomaterials.
Bottom-up synthesis of TiO2 nanostructures can be achieved by various chemical routes: sol–gel (Citation7–10), hydrothermal (Citation11), solvothermal (Citation12, Citation13), precipitation (Citation14) and electrochemical methods (Citation15). Among them, the sol–gel method is considered as the simplest approach for the preparation of TiO2 NPs since it is performed at room temperature and pressure, yields high homogeneity and purity and tolerates the doping of nanoparticles. However, this approach suffers from several shortcomings, including an inherent tendency towards aggregation and polydispersity of synthesized nanoparticles in addition to the use of toxic chemicals harmful to the environment: acids (HCl, HNO3) or bases (NaOH) are often used as reducing agents or as catalysts to favor the hydrolysis of metallic precursors which are commonly metal alkoxides or metal salts (Citation16, Citation17). Aggregation issue can be countered, to some extent, by introducing a suitable capping/stabilizer agent during the synthesis processing. Typically, organic compounds bearing carboxylic acid groups, such as polymers like polyethylene glycol (PEG) (Citation18), fatty acids (Citation19–22) and organic acids (Citation23), are often used as capping agents during the preparation of metal oxide nanoparticles.
Inspired by the biomineralization phenomenon, referred to as the processes by which organisms produce minerals, many biological systems, including bacteria, fungi, algae, yeast, mushroom and plant extracts, are increasingly applied towards the synthesis of nanomaterials, notably metal and metal oxides (Citation24, Citation25). Biosystems are used in an attempt to mimic biological mediums suitable for natural mineral growth. In this respect, plant-based methods for which plant extracts are either used as capping agents or both as reducing and capping/stabilizer agents have recently gained more interest in the synthesis of metal oxide nanoparticles with respect to standard physical and chemical methods.
To date, a limited number of papers have been published on the bio-mediated synthesis of TiO2 nanostructures using extracts from various plants, such as Euphorbia prostrata (Citation26), Trigonella foenum graecum (Citation27), Mentha arvensis (Citation28), Aloe vera (Citation29), Sesbania grandiflora (Citation30), Jatropha curcas L. (Citation31), Solanum trilobatum (Citation32), Annona squamosal (Citation33), Eclipta prostrate (Citation34), Catharanthus roseus (Citation35), Phyllanthus niruri (Citation36), Cinnamon powder (Citation37), Bixa orellana seed (Citation38), pineapple, grape and orange (Citation39), mango-peel (Citation40) and Plectranthus amboinicus(Citation41). Recently, leaf extracts from Piperbetel, Ocimum tenuiflorum, Moringa oleifera and Coriandrum sativum were used as reducing agents for the synthesis of TiO2 nanoparticles from titanium tetraisopropoxide precursor (Citation42). More recently, aqueous Citrus limon extract was used as capping agent for the synthesis of TiO2 nanoparticles already generated by the hydrolysis of titanium butoxide using acetic acid and applied to design a dye-sensitized solar cell (Citation43).
In this study, Citrus limon extract was used as a biological source of citric acid that acts both as a reducing agent to favor the hydrolysis of the precursor molecules (titanium butoxide) and as a capping agent that hinders the surface chemical reactivity of the TiO2 nanoparticles via the formation of a strong coordination between citrate ions and the Ti4+ ions so that the aggregation of resulting TiO2 nanoparticles could be reduced (Citation44). Citrus limon was reported to contain mainly citric acid together with other bioactive compounds, notably phenolic and amino acids, vitamins, flavonoids and carbohydrates (Citation45). The synthesized TiO2 nanoparticles have been subjected to numerous characterizations using Fourier transform infrared (FTIR), XRD, Raman, XPS and SEM techniques. Furthermore, their antibacterial activities were assessed for both gram-positive and gram-negative bacteria.
2. Experimental section
2.1. Reagents and chemicals
Tetrabutyl titanate (C16H36O4Ti) was used as the precursor compound for the chemical and green processes. All chemicals and solvents were purchased from the Shanghai Chemical Reagent Company (China) and were used without any further purification. Citrus limon fruit was purchased from a local market.
2.2. Minimum inhibitory concentration determination
The minimum inhibitory concentration (MIC) of bacterial growth is the concentration of nanoparticles that completely inhibits bacterial growth. MIC was determined via the twofold microdilution method with sterile flat-bottom 96-well polystyrene plates, as detailed in the reference (Citation46). The test samples were serially diluted twofold with nutrient soup. The culture-inoculated plates were incubated at 37 °C for 24 h. The MIC values were evaluated as specific concentrations where a noticeable reduction in color formation due to bacterial growth inhibition was observed. All experiments were carried out in triplicate.
2.3. Capping/reducing agent extraction
Fresh Citrus limon fruits purchased from a local supermarket were washed with tap water and Millipore water, cut into pieces, then squeezed. The collected juice was filtered for 3 times using Whatman filter paper to obtain a clear solution.
2.4. Green synthesis of TiO2 nanostructures
TiO2 nanoparticles (G-TiO2) were prepared via a green method as follows: Tetrabutile titanate (C16H36O4Ti) (23 mL) was dissolved in ethanol (23 mL), and the solution was stirred for 15 min. Then, 23 mL of Citrus limon extract was added dropwise to the above solution under stirring until total precipitation. The mixture was then kept for drying in an incubator at 80 °C. Finally, the dried powder was calcined at 600 °C for 2 h. The titanium dioxide (C-TiO2) was prepared via the classical sol–gel method as described in one of our previous articles (Citation8).
2.5. Characterization techniques
Structural investigations were carried out using an XRD apparatus type Ultima IV from Rigaku Company. This diffractometer is equipped with a Cu Kα radiation source providing a wavelength λ: 1.54056 Å. Spectroscopic studies were achieved by a Raman microscope type SENTERRA II Compact from Brucker Company equipped with a laser source providing an excitation 532-nm wavelength. TiO2 nanoparticle surface topography investigations were conducted using a scanning electron microscope type Inspect F50. The chemical composition of the as-prepared TiO2 samples was realized by an XPS system type Thermo Scientific K-Alpha, including a Al Kα radiation source of an energy 1486.6 eV.
3. Results and discussion
3.1. XRD characterization
The structural phase of the green synthesized TiO2 (G-TiO2) was revealed by XRD and compared to that of the chemically synthesized TiO2. XRD diffractograms of the two samples calcined under the same conditions, at 600°C for 2 h, are depicted in . The diffraction pattern of the G-TiO2 sample showed several peaks located at 2θ values of 25.34°, 37.04°, 37.86°, 38.56°, 48.08°, 53.94°, 55.10°, 62.68°, 70.42° and 75.04° corresponding to the (011), (013), (004), (112), (020), (015), (121), (123), (024) and (116) crystallographic planes, respectively, of the tetragonal lattice belonging to the space group I41/amd, which match well with the JCD card N°: 96-900-8214 of the anatase phase of TiO2 (Citation47). A small peak located at 27° corresponds to the plane (111) of the rutile phase (JCPD card N° 96-900-4143) (Citation47). In addition, based on the Rietveld refinement performed using Match software (supplementary data), the fraction of the anatase phase was estimated at 96.8% against 3.2% for the rutile phase. In contrast, even though the chemically synthesized TiO2 (C-TiO2) sample was subjected to the same annealing process as the green synthesized one, all the peaks appearing in its XRD pattern match well with the rutile phase (JCPD card N° 96-900-9084) (Citation9, Citation10). Remarkably, the green synthesis approach seems to provide a more thermodynamically stable anatase phase relative to the chemical one.
3.2. Raman characterization
Raman spectroscopy of the synthesized TiO2 sample was carried out to gather more information about the phase structure of the samples. (G-TiO2) exhibits Raman peaks located at 143 cm−1 (Eg), 197 cm−1 (Eg), 396 cm−1 (B1g), 519 cm−1 (A1g+B1g) and 639 cm−1 (Eg) corresponding to the anatase phase (Citation48, Citation49). The inset figure corresponding to a zoom of the dashed region revealed a small peak located at 447 cm−1 (Eg) attributed to the rutile phase (Citation48, Citation49). In contrast, the spectrum of the C-TiO2 presents three main peaks located at 238, 445 and 610 cm−1 attributed to the multiphonon process, Eg and A1g vibration modes of the rutile phase, respectively (Citation50).
3.3. Elemental analysis
Further investigations of the as-prepared samples TiO2 were carried out using X-ray photoelectrons spectroscopy (XPS). The survey spectra corresponding to both the G-TiO2 and the C-TiO2 nanoparticles as well the high-resolution spectra corresponding to the different elements identified in the samples are depicted in and .
The TiO2 surveys for the C-TiO2 and G-TiO2 samples revealed the characteristic peaks corresponding to Ti2p and O1s. Both spectra show a peak located at 287 eV attributed to the C1s state of the adventitious carbon from the environmental contaminations. This result indicates the purity of the synthesized samples.
The deconvolution of the high-resolution scan of the Ti2p of the C-TiO2 sample presents two peaks located at 459.78 and 465.48 eV corresponding to the Ti2p3/2 and Ti2p1/2 spin–orbit splitting, separated by 5.7 eV matching well with the reported values for TiO2 (Citation51). Similarly, the deconvolution of the high-resolution plot of Ti2p of the G-TiO2 sample presents two peaks located at 459.3 and 465 eV corresponding to the Ti2p3/2 and Ti2p1/2 spin–orbit splitting, separated by 5.3 eV. The deconvolution of the O1s peak of the C-TiO2 sample shows a major peak at 530.38 eV, attributed to the lattice oxygen (TiO2), and a small peak at 531.48 eV, attributed to the adsorbed hydroxyl groups, Ti–OH (Citation52, Citation53). Likewise, the fitting of the O1s peak of the G-TiO2 sample shows a core peak at 530.35 eV, attributed to the lattice oxygen (TiO2) in addition to a slight peak at 531.39 eV assigned to the adsorbed hydroxyl groups, Ti–OH.
3.4. Morphologic analysis
Morphological features of the C-TiO2 and the G-TiO2 nanoparticles were revealed by SEM and the recorded images are shown in . The C-TiO2 sample ((a)) showed a porous structure of capsule-shaped nanoparticles. In contrast, the G-TiO2 samples appeared to be clearly different in terms of morphology with respect to the C-TiO2 sample. In fact, the SEM image of G-TiO2 nanoparticles display a porous spherically shaped structure ((b)).
3.5. Infrared spectroscopy
shows the FTIR spectrum of G-TiO2. It indicates the presence of a broad band around 3200 cm−1 characteristic of OH stretching vibrations and a band at 1650 cm−1 corresponding to the bending vibration of the Ti–OH group (Citation53). The FTIR region 800–400 cm−1 characteristic of the Ti-O-Ti stretching vibration (Citation54–56).
3.6. Antibacterial activity
The antibacterial efficacy of green synthesized TiO2 nanoparticles is usually evaluated on model bacterial strains, such as Escherichia coli (Citation10). Generally, the main procedures involve microdilution and turbidity assays (Citation57, Citation58). Herein, the antibacterial activity of the G-TiO2 nanoparticles was compared to both those of C-TiO2 nanoparticles and the standard antibiotic drug Ciprofloxacin. In this respect, the activities of the above agents were evaluated via their action against four different bacterial strains: Klebsiella, MRSA, Bacillus, and E. coli in terms of MIC values by performing microdilution experiments.
As presented in , the G-TiO2 and C-TiO2 seem to entirely inhibit the E. coli bacteria at the same concentration (12.5 μg/ml), while, for the three other bacteria pathogens, G-TiO2 nanoparticles appear to inhibit the bacterial growth at lower concentrations compared to the C-TiO2. Generally, the antibacterial activity of TiO2 nanostructures is known to be influenced by their physico-chemical properties including, structural phase, size, shape, surface-to-mass ratio, and surface chemical groups (Citation59). However, the most accepted mechanism for antibacterial activity is based on the generation of reactive oxygen species (ROS) associated with the photocatalytic activity of TiO2 nanostructures (Citation60). Thus, the photocatalytic performance of anatase is generally considered to be higher than that of the rutile because of the higher density of localized states, associated with higher surface-adsorbed hydroxyl groups (8% for G-TiO2 against 3% for C-TiO2, ), and slower charge recombination in anatase relative to rutile (Citation61).
Table 1. MIC values of the corresponding to the antibacterial activity of G-TiO2, C-TiO2 and Ciprofloxacin antibiotic against various bacterial pathogens (µg/ml).
G-TiO2 appeared to perform the same antibacterial effect as the Ciprofloxacin antibiotic. The remarkable antibacterial activity of G-TiO2 could be explained by its multiple antimicrobial mechanisms of action, compared to Ciprofloxacin antibiotic which acts only by targeting two specific DNA enzymes (DNA topoisomerase and DNA-gyrase) causing the inhibition of the DNA replication and consequently the inhibition of the bacteria multiplication (Citation62). Overall, G-TiO2 could be a promising alternative to fight bacteria pathogens particularly antibiotic-resistant strains.
4. Conclusion
This work contributes to the valorization of bio-resources in the synthesis of titanium dioxide crystals using Citrus limon fruit extract as a capping/reducing agent on tetrabutile titanate precursor. Several analytical techniques were used to characterize the prepared samples: X-ray diffraction, infrared spectroscopy, SEM and XPS spectroscopy. Structural characterization by X-ray diffraction revealed an anatase crystal structure of the synthesized TiO2. The MIC values of the green synthesized TiO2 showed that its antibacterial potency is similar to that of the standard-antibacterial drug Ciprofloxacin.
Supplemental Material
Download MS Word (58.6 KB)Disclosure statement
No potential conflict of interest was reported by the author(s).
Additional information
Funding
References
- Arafat, M.M.; Dinan, B.; Akbar, S.A.; Haseeb, A. Gas Sensors Based on one Dimensional Nanostructured Metal-Oxides: A Review. Sensors 2012, 12, 7207–7258.
- Kannan, K.; Radhika, D.; Sadasivuni, K.K.; Reddy, K.R.; Raghu, A.V. Nanostructured Metal Oxides and its Hybrids for Photocatalytic and Biomedical Applications. Adv. Colloid Interface Sci. 2020, 281, 102178.
- Solanki, P.R.; Kaushik, A.; Agrawal, V.V.; Malhotra, B.D. Nanostructured Metal Oxide-Based Biosensors. NPG Asia Mater. 2011, 3, 17–24.
- van de Krol, R.; Liang, Y.; Schoonman, J. Solar Hydrogen Production With Nanostructured Metal Oxides. J. Mater. Chem. 2008, 18, 2311–2320.
- Wu, H.B.; Chen, J.S.; Hng, H.H.; Lou, X.W.D. Nanostructured Metal Oxide-Based Materials as Advanced Anodes for Lithium-ion Batteries. Nanoscale. 2012, 4, 2526–2542.
- Ziental, D.; Czarczynska-Goslinska, B.; Mlynarczyk, D.T.; Glowacka-Sobotta, A.; Stanisz, B.; Goslinski, T.; Sobotta, L. Titanium Dioxide Nanoparticles: Prospects and Applications in Medicine. Nanomaterials 2020, 10, 387.
- Kaiba, A.; Ouerghi, O.; Geesi, M.H.; Elsanousi, A.; Belkacem, A.; Dehbi, O.; Alharthi, A.I.; Alotaibi, M.A.; Riadi, Y. Characterization and Catalytic Performance of Ni-Doped TiO2 as a Potential Heterogeneous Nanocatalyst for the Preparation of Substituted Pyridopyrimidines. J. Mol. Struct. 2020, 1203, 127376.
- Geesi, M.H.; Ouerghi, O.; Elsanousi, A.; Kaiba, A.; Riadi, Y. Ultrasound-assisted Preparation of Cu-Doped TiO2 Nanoparticles as a Nanocatalyst for Sonochemical Synthesis of Pyridopyrimidines. Polycyc. Arom. Compd. 2020, 42, 1–11.
- Riadi, Y.; Geesi, M.H.; Ouerghi, O.; Azzallou, R.; Dehbi, O.; Lazar, S. Sol-gel TiO2 Nanostructures Single Doped with Copper and Nickel as Nanocatalysts for Enhanced Performance for the Liebeskind–Srogl Reaction. Mater. Chem. Phys. 2021, 267, 124607.
- Ouerghi, O.; Geesi, M.H.; Ibnouf, E.O.; Ansari, M.J.; Alam, P.; Elsanousi, A.; Kaiba, A.; Riadi, Y. Sol-gel Synthesized Rutile TiO2 Nanoparticles Loaded with Cardamom Essential oil: Enhanced Antibacterial Activity. J. Drug Deliv. Sci. Technol. 2021, 64, 102581.
- Iraj, M.; Nayeri, F.D.; Asl-Soleimani, E.; Narimani, K. Controlled Growth of Vertically Aligned TiO2 Nanorod Arrays Using the Improved Hydrothermal Method and Their Application to Dye-Sensitized Solar Cells. J. Alloys Compd. 2016, 659, 44–50.
- Riadi, Y.; Geesi, M.H.; Ouerghi, O.; Dehbi, O.; Elsanousi, A.; Azzallou, R. Synergistic Catalytic Effect of the Combination of Deep Eutectic Solvents and Hierarchical H-TiO2 Nanoparticles Toward the Synthesis of Benzimidazole-Linked Pyrrolidin-2-One Heterocycles: Boosting Reaction Yield. Polycyc. Arom. Compd. 2021, 1–15.
- Moura, K.F.; Maul, J.; Albuquerque, A.R.; Casali, G.P.; Longo, E.; Keyson, D.; Souza, A.G.; Sambrano, J.R.; Santos, I.M.G. TiO2 Synthesized by Microwave Assisted Solvothermal Method: Experimental and Theoretical Evaluation. J. Solid State Chem. 2014, 210, 171–177.
- Buraso, W.; Lachom, V.; Siriya, P.; Laokul, P. Synthesis of TiO2 Nanoparticles via a Simple Precipitation Method and Photocatalytic Performance. Mater. Res. Expr. 2018, 5, 115003.
- Anandgaonker, P.; Kulkarni, G.; Gaikwad, S.; Rajbhoj, A. Synthesis of TiO2 Nanoparticles by Electrochemical Method and Their Antibacterial Application. Arab. J. Chem. 2019, 12, 1815–1822.
- Mishra, S.; Daniele, S. Molecular Engineering of Metal Alkoxides for Solution Phase Synthesis of High-Tech Metal Oxide Nanomaterials. Chem. A Eur. J. 2020, 26, 9292–9303.
- Bluthardt, C.; Fink, C.; Flick, K.; Hagemeyer, A.; Schlichter, M.; Volpe Jr, A. Aqueous Synthesis of High Surface Area Metal Oxides. Catal. Today 2008, 137, 132–143.
- Singh, S.; Maurya, I.C.; Srivastava, P.; Bahadur, L. Synthesis of Nanosized TiO2 Using Different Molecular Weight Polyethylene Glycol (PEG) as Capping Agent and Their Performance as Photoanode in Dye-Sensitized Solar Cells. J. Solid State Electrochem. 2020, 24, 2395–2403.
- Cozzoli, P.D.; Kornowski, A.; Weller, H. Low-temperature Synthesis of Soluble and Processable Organic-Capped Anatase TiO2 Nanorods. J. Am. Chem. Soc. 2003, 125, 14539–14548.
- Cassagneau, T.; Fendler, J.H.; Mallouk, T.E. Optical and Electrical Characterizations of Ultrathin Films Self-Assembled from 11-Aminoundecanoic Acid Capped TiO2 Nanoparticles and Polyallylamine Hydrochloride. Langmuir 2000, 16, 241–246.
- Zhang, J.; Liu, X.; Wang, S.; Wu, S.; Cao, B.; Zheng, S. Synthesis and Catalytic Activity of Au-Supported Porous TiO2 Nanospheres for CO Oxidation. Powder Technol. 2012, 217, 585–590.
- Dinh, C.-T.; Nguyen, T.-D.; Kleitz, F.; Do, T.-O. Shape-controlled Synthesis of Highly Crystalline Titania Nanocrystals. ACS Nano 2009, 3, 3737–3743.
- Yang, S.; Yang, B.X.; Wu, L.; Li, Y.H.; Liu, P.; Zhao, H.; Yu, Y.Y.; Gong, X.Q.; Yang, H.G. Titania Single Crystals with a Curved Surface. Nature Commun. 2014, 5, 1–7.
- Singh, P.; Kim, Y.-J.; Zhang, D.; Yang, D.-C. Biological Synthesis of Nanoparticles from Plants and Microorganisms. Trends Biotechnol. 2016, 34, 588–599.
- Peralta-Videa, J.R.; Huang, Y.; Parsons, J.G.; Zhao, L.; Lopez-Moreno, L.; Hernandez-Viezcas, J.A.; Gardea-Torresdey, J.L. Plant-based Green Synthesis of Metallic Nanoparticles: Scientific Curiosity or a Realistic Alternative to Chemical Synthesis? Nanotechnol. Environ. Eng. 2016, 1, 1–29.
- Zahir, A.A.; Chauhan, I.S.; Bagavan, A.; Kamaraj, C.; Elango, G.; Shankar, J.; Arjaria, N.; Roopan, S.M.; Rahuman, A.A.; Singh, N. Green Synthesis of Silver and Titanium Dioxide Nanoparticles Using Euphorbia Prostrata Extract Shows Shift from Apoptosis to G0/G1 Arrest Followed by Necrotic Cell Death in Leishmania donovani. Antimicrob. Agents Chemother. 2015, 59, 4782–4799.
- Subhapriya, S.; Gomathipriya, P. Green Synthesis of Titanium Dioxide (TiO2) Nanoparticles by Trigonella Foenum-Graecum Extract and its Antimicrobial Properties. Microb. Pathog. 2018, 116, 215–220.
- Ahmad, W.; Jaiswal, K.K.; Soni, S. Green Synthesis of Titanium Dioxide (TiO2) Nanoparticles by Using Mentha arvensis Leaves Extract and its Antimicrobial Properties. Inorg. Nano-Met. Chem. 2020, 50, 1032–1038.
- Venkatesh, K.S.; Krishnamoorthi, S.R.; Palani, N.S.; Thirumal, V.; Jose, S.P.; Wang, F.-M.; Ilangovan, R. Facile One Step Synthesis of Novel TiO2 Nanocoral by Sol–Gel Method Using Aloe Vera Plant Extract. Ind. J. Phys. 2015, 89, 445–452.
- Srinivasan, M.; Venkatesan, M.; Arumugam, V.; Natesan, G.; Saravanan, N.; Murugesan, S.; Ramachandran, S.; Ayyasamy, R.; Pugazhendhi, A. Green Synthesis and Characterization of Titanium Dioxide Nanoparticles (TiO2 NPs) Using Sesbania grandiflora and Evaluation of Toxicity in Zebrafish Embryos. Process Biochem. 2019, 80, 197–202.
- Goutam, S.P.; Saxena, G.; Singh, V.; Yadav, A.K.; Bharagava, R.N.; Thapa, K.B. Green Synthesis of TiO2 Nanoparticles Using Leaf Extract of Jatropha curcas L. for Photocatalytic Degradation of Tannery Wastewater. Chem. Eng. J. 2018, 336, 386–396.
- Rajakumar, G.; Rahuman, A.A.; Jayaseelan, C.; Santhoshkumar, T.; Marimuthu, S.; Kamaraj, C.; Bagavan, A.; Zahir, A.A.; Kirthi, A.V.; Elango, G. Solanum trilobatum Extract-Mediated Synthesis of Titanium Dioxide Nanoparticles to Control Pediculus humanus Capitis, Hyalomma Anatolicum and Anopheles subpictus. Parasitol. Res. 2014, 113, 469–479.
- Roopan, S.M.; Bharathi, A.; Prabhakarn, A.; Rahuman, A.A.; Velayutham, K.; Rajakumar, G.; Padmaja, R.D.; Lekshmi, M.; Madhumitha, G. Efficient Phyto-Synthesis and Structural Characterization of Rutile TiO2 Nanoparticles Using Annona squamosa Peel Extract. Spectrochim. Acta Part A Mol. Biomol. Spectrosc. 2012, 98, 86–90.
- Rajakumar, G.; Rahuman, A.A.; Priyamvada, B.; Khanna, V.G.; Kumar, D.K.; Sujin, P.J. Eclipta Prostrata Leaf Aqueous Extract Mediated Synthesis of Titanium Dioxide Nanoparticles. Mater. Lett. 2012, 68, 115–117.
- Velayutham, K.; Rahuman, A.A.; Rajakumar, G.; Santhoshkumar, T.; Marimuthu, S.; Jayaseelan, C.; Bagavan, A.; Kirthi, A.V.; Kamaraj, C.; Zahir, A.A. Evaluation of Catharanthus Roseus Leaf Extract-Mediated Biosynthesis of Titanium Dioxide Nanoparticles Against Hippobosca Maculata and Bovicola Ovis. Parasitol. Res. 2012, 111, 2329–2337.
- Shanavas, S.; Priyadharsan, A.; Karthikeyan, S.; Dharmaboopathi, K.; Ragavan, I.; Vidya, C.; Acevedo, R.; Anbarasana, P.M. Green Synthesis of Titanium Dioxide Nanoparticles Using Phyllanthus niruri Leaf Extract and Study on its Structural, Optical and Morphological Properties. Mater. Today Proc. 2020, 26, 3531–3534.
- Nabi, G.; Raza, W.; Tahir, M.B. Green Synthesis of TiO2 Nanoparticle Using Cinnamon Powder Extract and the Study of Optical Properties. J. Inorg. Organomet. Polym. Mater. 2020, 30, 1425–1429.
- Maurya, I.C.; Singh, S.; Senapati, S.; Srivastava, P.; Bahadur, L. Green Synthesis of TiO2 Nanoparticles Using Bixa orellana Seed Extract and its Application for Solar Cells. Sol. Energy 2019, 194, 952–958.
- Senthamarai, R.; Madurai Ramakrishnan, V.; Palanisamy, B.; Kulandhaivel, S. Synthesis of TiO2 Nanostructures by Green Approach as Photoanodes for Dye-Sensitized Solar Cells. Inter. J. Energy Res. 2021, 45, 3089–3096.
- Isnaeni, I.N.; Sumiarsa, D.; Primadona, I. Green Synthesis of Different TiO2 Nanoparticle Phases Using Mango-Peel Extract. Mater. Lett. 2021, 294, 129792.
- Rajendhiran, R.; Deivasigamani, V.; Palanisamy, J.; Pitchaiya, S.; Eswaramoorthy, N.; Masan, S. Plectranthus amboinicus Leaf Extract Synthesized Spherical Like-TiO2 Photoanode for Dye-Sensitized Solar Cell Application. Silicon 2021, 1–8.
- Pushpamalini, T.; Keerthana, M.; Sangavi, R.; Nagaraj, A.; Kamaraj, P. Comparative Analysis of Green Synthesis of TiO2 Nanoparticles Using Four Different Leaf Extract. Mater. Today Proc. 2021, 40, S180–S184.
- Singh, S.; Maurya, I.C.; Tiwari, A.; Srivastava, P.; Bahadur, L. Green Synthesis of TiO2 Nanoparticles Using Citrus Limon Juice Extract as a Bio-Capping Agent for Enhanced Performance of Dye-Sensitized Solar Cells. Surf. Interf. 2022, 28, 101652.
- Navaneethan, M.; Nithiananth, S.; Abinaya, R.; Harish, S.; Archana, J.; Sudha, L.; Ponnusamy, S.; Muthamizhchelvan, C.; Ikeda, H.; Hayakawa, Y. Hydrothermal Growth of Highly Monodispersed TiO2 Nanoparticles: Functional Properties and dye-Sensitized Solar Cell Performance. Appl. Surf. Sci. 2017, 418, 186–193.
- González-Molina, E.; Domínguez-Perles, R.; Moreno, D.A.; García-Viguera, C. Natural Bioactive Compounds of Citrus Limon for Food and Health. J. Pharm. Biomed. Anal. 2010, 51, 327–345.
- Andrews, J.M. Determination of Minimum Inhibitory Concentrations. J. Antimicrob. Chemother. 2001, 48, 5–16.
- Kashale, A.A.; Gattu, K.P.; Ghule, K.; Ingole, V.H.; Dhanayat, S.; Sharma, R.; Chang, J.-Y.; Ghule, A.V. Biomediated Green Synthesis of TiO2 Nanoparticles for Lithium ion Battery Application. Compos. Part B Eng. 2016, 99, 297–304.
- Sun, Y.; Wang, S.; Zheng, J. Biosynthesis of TiO2 Nanoparticles and Their Application for Treatment of Brain Injury-An in-Vitro Toxicity Study Towards Central Nervous System. J. Photochem. Photobiol. B Biol. 2019, 194, 1–5.
- Alamelu, K.; Raja, V.; Shiamala, L.; Ali, B.J. Biphasic TiO2 Nanoparticles Decorated Graphene Nanosheets for Visible Light Driven Photocatalytic Degradation of Organic Dyes. Appl. Surf. Sci. 2018, 430, 145–154.
- Ganesh, I.; Gupta, A.K.; Kumar, P.P.; Sekhar, P.C.; Radha, K.; Padmanabham, G.; Sundararajan, G. Preparation and Characterization of Co-Doped TiO2 Materials for Solar Light Induced Current and Photocatalytic Applications. Mater. Chem. Phys. 2012, 135, 220–234.
- Erdem, B.; Hunsicker, R.A.; Simmons, G.W.; Sudol, E.D.; Dimonie, V.L.; El-Aasser, M.S. XPS and FTIR Surface Characterization of TiO2 Particles Used in Polymer Encapsulation. Langmuir 2001, 17, 2664–2669.
- McCafferty, E.; Wightman, J.P. Determination of the Concentration of Surface Hydroxyl Groups on Metal Oxide Films by a Quantitative XPS Method. Surf. Interf. Anal. Inter. J. Dev. Develop. Applic. Techniq. Anal. Surf. Interf. Thin Films 1998, 26, 549–564.
- Jnido, G.; Ohms, G.; Viöl, W. Deposition of TiO2 Thin Films on Wood Substrate by an Air Atmospheric Pressure Plasma Jet. Coatings 2019, 9, 441.
- Olayo, M.G.; González-Salgado, F.; Cruz, G.J.; Gómez, L.M.; García-Rosales, G.; Gonzalez-Torres, M.; Lopez-Gracia, O.G. Chemical Structure of TiO Organometallic Particles Obtained by Plasma. Adv. Nanopart. 2013, 2, 229.
- Vetrivel, V.; Rajendran, K.; Kalaiselvi, V. Synthesis and Characterization of Pure Titanium Dioxide Nanoparticles by Sol-Gel Method. Int. J. Chem. Tech. Res 2015, 7, 1090–1097.
- Al-Taweel, S.S.; Saud, H.R. New Route for Synthesis of Pure Anatase TiO2 Nanoparticles via Utrasound-Assisted Sol-Gel Method. J. Chem. Pharm. Res 2016, 8, 620–626.
- Piddock, L.J. Techniques Used for the Determination of Antimicrobial Resistance and Sensitivity in Bacteria. J. Appl. Bacteriol. 1990, 68, 307–318.
- J.H. Jorgensen, J.D. Turnidge, Susceptibility test methods: dilution and disk diffusion methods, Manual of Clinical Microbiology. 1253–1273, 2015.
- Jamil, B.; Bokhari, H.; Imran, M. Mechanism of Action: How Nano-Antimicrobials Act? Curr. Drug Targ. 2017, 18, 363–373.
- Kheiri, S.; Liu, X.; Thompson, M. Nanoparticles at Biointerfaces: Antibacterial Activity and Nanotoxicology. Colloids Surf. B Biointerf. 2019, 184, 110550.
- Luttrell, T.; Halpegamage, S.; Tao, J.; Kramer, A.; Sutter, E.; Batzill, M. Why is Anatase a Better Photocatalyst Than Rutile?-Model Studies on Epitaxial TiO2 Films. Sci. Rep. 2014, 4, 1–8.
- LeBel, M. Ciprofloxacin: Chemistry, Mechanism of Action, Resistance, Antimicrobial Spectrum, Pharmacokinetics, Clinical Trials, and Adverse Reactions. Pharmacother. J. Hum. Pharmacol. Drug Ther. 1988, 8, 3–30.