ABSTRACT
Polyesters are important materials with a wide range of applications, but there has been increasing concern over their sustainability. One example is the need for safer, bio-derived solvents to replace those currently in use for the polymer’s synthesis and processing. In this work, several variants of the bio-based cellulose/levoglucosenone derived solvent Cyrene, namely the ketal derivatives dioxolane Cygnet, dioxane Cygnet and dioxepane Cygnet were synthesized and tested as media for enzymatic polycondensation reactions using bio-based building blocks. Dioxolane Cygnet and dioxepane Cygnet were found to be suitable solvents for enzymatic polycondensation reactions, with dioxolane Cygnet being the preferred solvent, yielding polymers with a Mn >22 kDa. In addition, these solvents were tested in the biocatalyzed synthesis of levoglucosenone-based polyesters. The alternative solvents gave superior yields to those previously observed, demonstrating the versatility of these solvents in enzymatic polycondensation reactions, representing the first synthetic polymer-solvent system fully derived from cellulose.
GRAPHICAL ABSTRACT
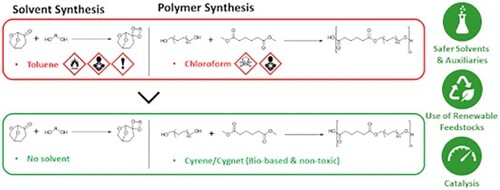
Introduction
Polymers are ubiquitous in the modern world, being both incredibly useful and causing a huge environmental impact. Most polymers are petroleum-derived and do not biodegrade, leaving behind a large amount of waste if not properly disposed and recycled. In 2015 it was estimated that in total, 4.9 billion tons of plastic waste had been discarded in landfill or the natural environment (Citation1), and the detrimental effects of this to wildlife (Citation2), human health, and the larger ecosystems (Citation3) are well documented. Making all stages of the lifecycle of a polymer more sustainable has been the focus of many research groups, whether that is testing alternative bio-based feedstocks (Citation4, Citation5), eliminating traditional organic solvents (Citation6, Citation7), or designing for recyclability (Citation8–11). Environmental sustainability is underpinned by the principles of green chemistry, which provide a framework for ‘greening’ chemical processes (Citation12).
Important in the field of polymer synthesis is principle 9 of Green Chemistry, which aims to use catalysts whenever possible (Citation13). Catalysts are commonly used in polymer synthesis, with Ziegler–Natta catalysts being the most popularly employed for polyolefin production (Citation14). For polyester synthesis, biocatalysts are particularly attractive from a sustainable perspective; they are derived from renewable resources and often require mild reaction conditions (Citation15). Another is principle 5 of Green Chemistry, the use of safer solvents and auxiliaries. Solvents represent a significant portion of the environmental impact of a reaction, and ionic liquids, supercritical fluids and bio based solvents have all been tested in polymer synthesis (Citation14).
Evaluating the ‘greenness’ of a solvent is a complex process. Several companies and organizations have published guides, which evaluate health, safety and environmental criteria of a solvent and provide a ranking or recommendation. Judgement is subjective and there is no singular established list of recommended solvents. Sanofi for example labeled acetonitrile as recommended, whereas GlaxoSmithKline concluded it had major issues (Citation16). Despite this, there is generally a good consensus between the different guides. These guides, coupled with environmental legislation such as REACH (Citation17) have increased pressure on chemists to find alternative solvents that are more benign.
Polyesters are usually synthesized in organic solvents such as diphenyl ether (DPE). This was found to be an excellent solvent for the synthesis of polyesters, achieving polymers of up to 10 kDa for polyesters containing diethyl succinate and 1,4-butanediol as the monomers (Citation18). Furthermore, more complex polymers containing the diester derivatives of pyridine dicarboxylic acids have also been synthesized in DPE, and very successfully; 2,4-diethyl pyridine dicarboxylate and 1,8-octanediol produced a polymer with a number average molecular weight (Mn) of 14.3 kDa (Citation19). Yet its use is problematic as it is extremely toxic to aquatic life and classed as highly hazardous due to its low flash point, auto-ignition temperature, and ability to form peroxides. Several alternative solvents have been investigated, such as short chain oxymethylene dimethyl ethers (OMEs). These gave a consistently higher yield when compared to DPE in the condensation of dimethyl adipate and various diols, but a lower Mn (Citation20). Another previous work tested various alternative solvents in several polycondensation reactions of which pinacolone was the best performing, although only produced polymers with Mn of up to 2.5 kDa (Citation21), which still falls short of DPE.
Dihydrolevoglucosenone, or CyreneTM, has become a molecule of increasing interest since its use as a solvent was reported in 2014 (Citation22). Currently synthesized commercially from cellulose in a two-step process – flash catalytic pyrolysis to give levoglucosenone as an intermediate followed by either a palladium-catalyzed hydrogenation (Citation22) or an OYE 2.6-mediated bioreduction (Citation23). Cyrene’s low toxicity makes it an attractive alternative for those researchers looking into replacements for traditional dipolar aprotic solvents (Citation24). Cyrene is a multi-functional molecule with the potential for countless derivatives. As of 2016, there were at least 164 molecules derived from levoglucosenone available in the literature (Citation25) with a current number of over 350 available molecules according to Scifinder. One promising group are the Cygnets, ketals of Cyrene that could potentially be good replacements for dichloromethane (Citation25). There are few examples of using Cyrene in biocatalysis (Citation26, Citation27), but to the best of our knowledge, only one concerning polyester synthesis, which also tests the Cygnet incorporating a dioxolane moiety (previously referred to as Cygnet 0.0) (Citation28). In this previous work, Cyrene, dioxolane Cygnet, and mixtures of the two were tested as a solvent for the polycondensation of various diesters and diols. This study found dioxolane Cygnet performed very well for every monomer combination. The condensation of dimethyl adipate and 1,8-octanediol was particularly successful, giving a yield of 91% and polymers with a Mn of up to 20.5 kDa (Citation28). The focus of this work is the testing and characterization of Cyrene (hereafter referred to as (1) and three of its derivatives, dioxolane Cygnet, dioxane Cygnet and dioxepane Cygnet) (hereafter referred to as cygnets (2), (3) and (4) respectively, according to the number of carbon atoms in the secondary ring), the latter two previously unreported, for enzymatic-catalyzed polycondensation reactions.
Materials & methods
Materials
Cyrene™ (≥98.5%), 1,4-butanediol (ReagentPlus® 99%), 1,8-octanediol (98%), dimethyl sebacate (99%), dimethyl succinate (98%) and Candida antarctica lipase B (CaLB, code: L4777) were purchased from Sigma Aldrich. Ethylene glycol (EMSURE® Reag. Ph Eur,Reag. USP ≥99.5%) was purchased from Merck. Dimethyl adipate (99%) was purchased from Alfa Aesar. Chloroform (≥99.8%) and ethyl acetate (HiPerSolv CHROMANOFORM) were purchased from VWR. Methanol was purchased from Carl Roth.
(S)-5-(hydroxymethyl)-3-[(2S,3S)-2-(hydroxymethyl)-5-oxotetrahydrofuran-3-yl]furan-2(5H)-one (2H-HBO-HBO) was synthesized according to the procedure described by Diot-Néant et al. (Citation29).
Cygnet derivatives synthesis
Cyrene (25.6 g, 0.2 mol) with an excess of the corresponding diol; ethylene glycol (24.8 g, 0.4 mol) for dioxolane Cygnet, 1,3-propanediol (30.4 g, 0.4 mol) for dioxane Cygnet and 1,4-butanediol (36.0 g, 0.4 mol) for dioxepane Cygnet, was placed in a 250 mL round bottom flask with a stirrer bar. 3 drops (approx. 250–300 mg) of phosphoric acid was added and the reaction was heated to 105°C at 400 rpm until conversion was shown to be >90% (1H-NMR). Liquid–liquid extraction was performed to remove excess diol. 100 mL of ethyl acetate was added to the reaction medium, and washed with water (3 × 20 mL) using a separation funnel. The organic layer was dried with magnesium sulfate and filtered through paper (150 mm 595 1/2). Ethyl acetate was subsequently removed via rotary evaporation. Dioxolane cygnet required no further purification, as analysis showed >99% purity (1H-NMR).
Synthesis of dioxepane cygnet required further purification through recrystallisation. Ethyl acetate was added to the crude dioxepane Cygnet/Cyrene mix and the solution was heated until full dissolution was observed. Solution was left to cool to room temperature, covered and then placed in an ice bath. When crystals formed, the solution was left to rest for 1 h and was subsequently vacuum filtrated to obtain a white crystalline solid. This procedure was repeated until analysis showed dioxepane cygnet to be >99% pure (1H-NMR).
Optimization of polymerization procedure
In the first iteration of this procedure, dimethyl adipate (139.4 mg, 8 × 10−4 mol) and either 1,4-butanediol (BDO) (72.1 mg, 8 × 10−4 mol) or 1,8-octanediol (ODO) (117.0 mg, 8 × 10−4 mol) were added to a 25-mL round bottom flask with 2 g or 4 g of Cyrene, and 10% by weight of monomers of CaLB. The flask was heated to 85°C and stirred at 400 rpm for 6 h, at which time the system, was placed under vacuum (20 mbar) for a further 18 h. Aside from mass of solvent and time under vacuum, all parameters remained the same in subsequent reactions.
Work-up 1: Used in the original procedure. The polymers were washed with approx. 5 mL of CHCl3 and filtered through cotton to remove the enzyme. The flask was washed with approx. 1 mL of CHCl3 a further three times and all fractions were collected. The solvent was removed from the mixture via a rotary evaporator and transferred to a 50 mL Falcon tube. Ice cold methanol (35 mL) were added to precipitate the polymer. The mixture was vortexed and then centrifuged at 3700 rpm for 10 min at 4°C. The supernatant was removed, and 20 mL of ice-cold methanol was added, repeating the washing step a further two times.
Work-up 2: Used when using CHCl3 as the work-up solvent and water as the anti-solvent. Polymers were washed with approx. 5 mL of CHCl3 and filtered through cotton to remove the enzyme. The flask was washed with approx. 1 mL of CHCl3 a further three times and all fractions were collected. The mixture was rotary evaporated to remove the solvent and then transferred to a 50-mL Falcon tube. Cold water (35 mL) was added to precipitate the polymer. The mixture was vortexed and filtered through a 150 mm 595 1/2 filter paper. The polymer was washed with water three times.
Work-up 3: Used when CHCl3 was replaced with Cyrene. The polymers were filtered through cotton and the round bottom flask washed with 2 × 1 mL Cyrene. To this was added 35 mL cold anti-solvent (water or methanol). If the anti-solvent used was methanol, then the centrifugation procedure in work up 1 was followed. If the anti-solvent used was water, the filtration procedure in work-up 2 was followed.
Characterization
Nuclear magnetic resonance (NMR) spectroscopy
All 1H-NMR spectra were recorded on a Bruker Avance II 400 (resonance frequencies 400.13 MHz for 1H) equipped with a 5 mm N2-cooled cryo probe (Prodigy) with z–gradients at room temperature with standard Bruker pulse programs. The samples were dissolved in 0.6 mL of CDCl3 or DMSO-d6 (98% D, Eurisotop, Saint-Aubin, France). Chemical shifts are given in ppm, referenced to residual solvent signals (7.26 ppm for CDCl3, 2.49 ppm for DMSO-d6).
Gel permeation chromatography (GPC)
BDO and ODO based polymers were dissolved in CHCl3 to a concentration between 2 and 2.5 mg/mL and filtered through cotton wool packed into a 150 mm glass Pasteur pipette. The analysis was performed at 30 °C on an Agilent Technologies HPLC System (Agilent Technologies 1260 Infinity) connected to a 17,369 6.0 mm ID × 40 mm LHHR-H, 5 μm Guard column and a 18,055 7.8 mm ID × 300 mm L GMHHR-N, 5 μm TSK gel liquid chromatography column (Tosoh Bioscience, Tessenderlo, Belgium) using CHCl3 as an eluent (at a flow rate of 1 mL min−1 for 20 min). An Agilent Technologies G1362A refractive index detector was employed for detection. Linear polystyrene calibration standards (250–70,000 Da) purchased from Sigma-Aldrich were used to calculate the molecular weights of the polymers.
2H-HBO-HBO-based polymers were dissolved in DMF (10 mM LiBr) at a concentration between 2.5 and 3 mg/mL and filtered through syringe filters (RC 0.20 µm). The analysis was performed at 50°C using an Agilent Technologies 1260 Infinity Series liquid chromatography system with an internal differential refractive index detector, a viscometer detector, a laser, UV lamp and two PLgel columns (5 µm MIXED-D 300 × 7.5 mm) using 10 mM Lithium Bromide in HPLC grade DMF as the mobile phase at a flow rate of 1.0 mL min−1. Calibration was performed with poly(methyl methacrylate) standards from Agilent Technologies.
Thermogravimetric analysis (TGA)
TGA was performed on a Netzsch TG 209 F1 instrument. Samples (3-8 mg) were analyzed in an Al2O3 crucible from 20 to 700°C, with a heating rate of 10 K/min and a cooling rate of 30 K/min under a N2 atmosphere.
Differential scanning calorimetry (DSC)
Samples between 2 and 5 mg were weighed out into concave aluminum pans and measurements were performed on a Netzsch DSC 214 polyma instrument. A heating rate of 10 K/min was used and the temperature range analyzed was -40–250 °C. Two cycles were completed under a N2 atmosphere.
X-ray crystallography
Diffraction data were collected at 110 K on an Oxford Diffraction SuperNova diffractometer with Cu-Kα radiation (λ = 1.54184 Å) using an EOS CCD camera. The crystal was cooled with an Oxford Instruments Cryojet. Diffractometer control, data collection, initial unit cell determination, frame integration and unit-cell refinement were carried out with CrysAlisPro (Citation30). Face-indexed absorption corrections were applied using spherical harmonics, implemented in SCALE3 ABSPACK (Citation31) scaling algorithm within CrysAlisPro. OLEX2 (Citation32) was used for overall structure solution, refinement and preparation of computer graphics and publication data. Within OLEX2, the algorithms used for structure solution were ShelXS direct methods, (Citation33) for (3) and ShelXT dual-space (Citation34) for (4). Refinement by full-matrix least-squares used the ShelXL (Citation35) algorithm within OLEX2 (Citation32). All non-hydrogen atoms were refined anisotropically. Hydrogen atoms were placed by difference map and allowed to refine. CCDC numbers are: (3): 2163960 (4): 2163961.
Conductor-like screening model for realistic solvation (COSMO-RS) computational studies
Solvent conformers and surface energies were calculated with COSMOconfX (version 4.0; COSMOlogic GmbH & Co. KG, Leverkusen, Germany, 2015) using Turbomole at the BP (B88-VWN-P86) density functional theory level and triple-z valence polarized (TZVP) basis set. COSMOthermX (version C30_1705; COSMOlogic GmbH & Co. KG, 2017) was used to visualize the molecular surface charges, σ-profiles, and σ-potentials. COSMO-RS calculations are courtesy of, and authorized for the purposes of, Circa Group Pty Ltd.
Results and discussion
Optimization of the enzymatic polycondensation reactions
Hydrophobic, high boiling point solvents are the classic solvent choice for enzymatic polycondensation reactions (Citation36). Diphenyl ether (DPE) in particular is noted for yielding polymers with high molecular weights and has been used for the synthesis of both aliphatic (Citation37, Citation38) and aromatic (Citation39) polyesters, despite its status as a non-green solvent. More recently, oxymethylene dimethyl ethers have been used to enzymatically synthesize polyesters using dimethyl adipate and diols of varying length (Citation20). Greener solvents such as TMO and pinacolone have also been tested in this reaction with success (Citation21). However, all previous studies have the disadvantage of using toxic solvents such as CHCl3 during the work-up stage, which were eliminated from the procedure developed in the present study.
The enzymatic polycondensation of dimethyl adipate with either 1,4-butanediol (BDO) or 1,8-octanediol (ODO) was carried out firstly using Cyrene (1) as the reaction solvent and a ‘green’ alternative to DPE. This was successful (Figures S29–S30), albeit initial yields for the BDO-based polymers were markedly low (reactions 1 and 3, ). The reaction protocol was then optimized by varying (1) reaction time, (2) amount of reaction solvent, and (3) choice of work-up solvent ( and Table S1 in ESI). The choice of work-up solvent and anti-solvent has a large influence on the final state of the isolated polymer, with the workup solvent that must be able to dissolve the polymer sufficiently so that minimal amounts are lost during filtration, and subsequent addition of the anti-solvent must alter the solubility parameters enough that the polymer crashes out in the solvent blend. Here, (1) was chosen as an alternative workup solvent to chloroform as its success as a reaction solvent showed it had a compatible solubility profile with both our model polymers.
Figure 1. Overview of all reactions performed during the optimization stage. Percentage yield of (a) BDO-based polymers and (b) ODO-based polymers. Number average (Mn) and weight average (Mw) molecular weight of (c) BDO-based polymers and (d) ODO-based polymers. A complete Figure legend can be found in the ESI as Table S1.
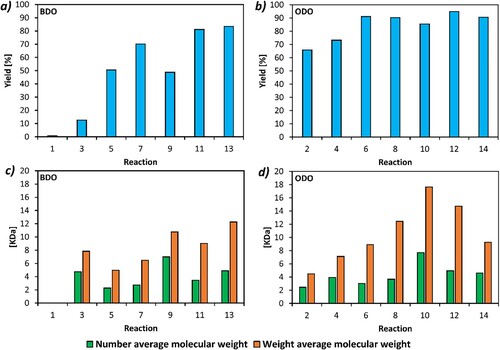
When looking to optimize this reaction, increasing the reaction time from 24 to 96 h increased the reaction yield by ∼10% for both the BDO and the ODO-based polymers (reaction 3 and 4, ). Although CHCl3 was replaced by (1) mainly due to its issues with toxicity, when (1) was paired with water as an anti-solvent, it also resulted in the obtainment of higher polymer yields. The highest yield reported was 95% (reaction 12, ), from the ODO polymer synthesis using a (1)/water solvent/anti-solvent workup, and repetition of this experiment gave similar yields (Table S8 and Figure S31). Decreasing the solvent mass from 4 g to 2 g resulted in a drastic increase in yield for both reactions (up to 51% for the BDO based polymer, and 91% for the ODO based), showing that a higher monomers/solvent ratio greatly increases the polymer yield (reaction 5 and 6, ). In fact, ODO-based polymers had a consistently higher yield compared to BDO-based polymers, which has previously been attributed to both higher solubility of the shorter chain polymer products in the anti-solvent and preference of the immobilized CaLB for the more hydrophobic 1,8-octanediol(Citation20). Aside from reaction 1 in , there was little difference in monomer conversion, all having reported values >95% (Figure S7).
(1) was generally a superior work-up solvent compared to CHCl3. The only observed exception was when CHCl3 was combined with methanol as an anti-solvent where a slightly higher yield (an increase of 6%) was observed for the ODO-based polymer while no differences were observed for the BDO-based polymer (reactions 5 and 6 compared to reactions 9 and 10, ). Despite this, when CHCl3 was substituted with (1), there was always an increase in weight average molecular weight (Mw) and number average molecular weight (Mn), and often a decrease in dispersity (Đ) making the polymers more interesting for further applications.
Using water as an anti-solvent gave a higher yield when compared to methanol. However, when the Mn and Mw are considered, although the polymers worked up in CHCl3/H2O have much higher molecular weights than the CHCl3/MeOH polymers, this trend reversed when the work-up was performed in (1). A possible explanation for this trend is that the addition of water to (1) forms a geminal diol through hydration of the ketone moiety (Citation40), which increases the overall polarity of the mixture (Citation41). In our system, the wt% of (1) is approx. 11%, and previous studies have found the majority of (1) to be converted into its geminal diol at this concentration (Citation42). The (1)/H2O antisolvent system is therefore very different when compared to the (1)/MeOH one. When comparing the two solvent systems, a blend of Cyrene and water (in the appropriate ratio) assuming all Cyrene has been converted to the geminal diol has a δP of 38.7 compared to 21.4 for the Cyrene/MeOH system (Table S9). As the Cyrene/H2O system is much more polar, more polymer crashes out, including lower molecular weight oligomers. This both increases dispersity and decreases Mn and Mw for this system. Using a MeOH-based anti-solvent system resulted in higher Mw polymers, which can be seen in (c and d), when comparing the Mw of reactions 9 and 11, and reactions 10 and 12. The ODO-based polymer worked-up in (1)/MeOH gave the highest Mn/Mw in this data set; 7.7/17.6 kDa respectively.
2-methyl tetrahydrofuran (MeTHF) was also tested as a work-up solvent due to its low boiling point; if the reaction solvent wanted to be recovered for recycling, this could easily be removed via evaporation. A MeTHF/MeOH work-up procedure was focused on due to the miscibility of both these solvents. For the ODO-based polymer, the molecular weights produced by MeTHF/MeOH were lower than those using (1) in the work-up, although the yield had a slight increase (reactions 10 and 14, Table S1). For the BDO-based polymer, the MeTHF/MeOH work-up had the highest Mw (12.3 kDa) and the second highest Mn (4.9 kDa). Yield was also considerably higher for this polymer, an increase to 83% compared to the 49% obtained by (1)/MeOH.
Synthesis and structure of Cygnets
When first synthesized in the literature, Cygnet 0.0 (2) formation was acid catalyzed by KSF-200 (Citation25, Citation28). This catalytic system functioned well but if the heterogeneous catalyst was not freshly prepared, the product would be slightly discolored, presumably from leaching in the system. Discolored (2) gave poorer yields and conversions as compared to that prepared with fresh catalyst. To negate this, phosphoric acid was selected as a weak homogeneous acid to facilitate acetal formation over the ketone (a). Due to the high boiling point of the diols (which acted as both solvent and reactant), the reactions were carried out in an open vessel and at elevated temperature to drive off water produced. The excess diol and removal of water helped push the equilibrium towards the right (a) and favor the formation of the cygnet acetal. Cygnets (2), (3) and (4) were successfully synthesized using this method (Figures S1–S6). Upon cooling (2) crystallized instantaneously, (3) within minutes and (4) required hours (b). The crystal structure of the novel (3) and (4) was also resolved (c and Tables S4–S5).
Enzymatic polycondensations with Cygnets
DSC analysis was performed on all three Cygnets (Figure S9 in ESI). Dioxane Cygnet (3) exhibited a melting point of 86.2°C and experimental testing showed it did not completely melt in the reactor when set at 85°C, the optimal temperature for the enzymatic polycondensation reaction (Citation43). Only dioxolane (2) and dioxepane Cygnet (4) were therefore tested in the model polycondensation reaction. (1) and (2) have been previously used in enzymatic polycondensations (Citation28), while (3) and (4) have never been reported before. The polarity of ketals (2) – (4) were calculated as lower than (1) (Citation44) and more similar to the conventional solvent diphenyl ether.
Several workup procedures were tested. All experiments had >94% conversion (Figure S7), and those experiments that were repeated showed similar yields (Table S8 and Figure S31). In all conditions, use of (2) as the reaction solvent gave higher yields for the BDO-based polymers, as seen in (and Table S2 in ESI), while it gave similar yields to (1) for the ODO-based polymers. The only exception to this was when the workup solvent was changed from (1) to MeTHF; when using this workup, the BDO-based polymer synthesized in (1) had a superior yield to the one synthesized in (2), at 83% vs 73% respectively (reactions 13 and 19 in Table S1 and S2). ODO polymers in general gave good yields (>80%). This is in line with data collected from the optimization of the reaction. Moreover, as it is possible to notice from (and Table S1 in ESI), the (1)/water workup gave the highest yields for all three solvents for both polymers. Each solvent gave very similar yields for ODO but decreased from (2) to (1) and then (4) for the BDO-based polymer, which can be seen in (a) comparing reactions 11, 17 and 23. Overall, (1) and (2) seemed to be the best solvents for these reactions. When comparing equivalent conditions, (4) never outperformed (1) or (2).
Figure 3. Overview of all reactions performed using the Cygnets (2) and (4) as the reaction solvents and CaLB as the biocatalyst. Percentage yield of (a) BDO-based polymers and (b) ODO-based polymers. Number average (Mn) and weight average (Mw) molecular weight of (c) BDO-based polymers and (d) ODO-based polymers. A complete Figure legend can be found in the ESI as Table S2.
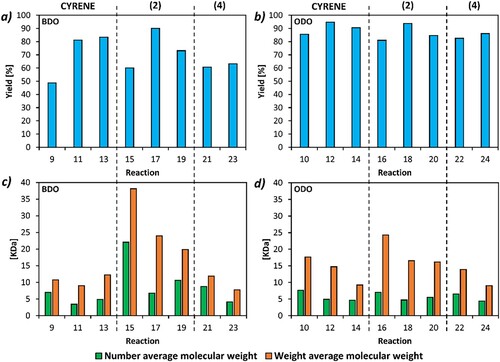
Although the use of MeTHF was solely to test if the reaction solvent could be recovered, polymer yield was also high. 1H-NMR of (2), showed no significant differences when compared to unused solvent (Figure S8 in ESI) demonstrating the recyclability of the reaction solvent.
For those polymers synthesized in (2), the BDO-based polymers always had a higher Mw and Mn compared to the ODO-based polymers. This can be explained through the computational analysis published in a previous paper (Citation21). It was calculated that the lowest energy conformation of BDO has an intramolecular hydrogen bond, unlike ODO which may form two intermolecular hydrogen bonds. COSMO-RS calculations indicated that ODO was more stable in solution than BDO at 85°C, and therefore less reactive. The electronic properties of the Cygnet solvents were calculated using the COSMO-RS method to assist in the understanding of polarity and solubility. This is a continuum solvation model combined with statistical thermodynamics to describe the energy and charge densities of molecules in a (virtual) conductor. From this information, chemical potentials and other thermodynamic properties can be calculated.
Amongst the tested solvents, we observed that (2) performed much better in these reactions when compared to (4) and (1) It is known that polarity affects the activity of CaLB activity (Citation45) which is consistent with the ketal (2) (δP value of 8.2) yielding polymers with higher Mw when compared to the more polar ketone (1) (δP value of 12.4). Solvent (4) with a δP value of 6.6 is only slightly less polar than (2), but the yield and molecular weights of these polymers were consistently significantly lower than (2), meaning that either δP (a function of dipole moment and molar volume) is an insufficient measure of polarity in this case or there are other factors affecting the resulting polymer yield and molecular weight.
A previous study indicates a log P/log KOW between 2 and 4.5 is optimal for efficient polymerization reactions with CaLB and that a lower log P value corresponds to a slow polymerization (Citation46). The biocatalyst CaLB is well documented to prefer less polar solvents as media, as solvents with high polarity disrupt water molecules bound to the enzyme, in a process called water stripping (Citation47). However, the calculated log P values of (2) and (4) fall outside this ‘optimal’ range and (2), despite producing polymers with both a higher yield and Mw, has a lower log P compared to (4).
Iemhoff and co-workers argue that the log P is insufficient to determine the effect a solvent will have on enzymatic reactions, and used the Kamlet–Taft solvatochromic scales instead, more specifically the H-bond accepting (β) parameter along with molar volume (Vm) (Citation48). When looking at enzymatic reactions, they found the highest initial rates of reaction for solvents which are bulky with low β values. This was attributed to (1) bulky solvents are detrimental to the formation of a structured solvation environment, which encourages the substrate to move into the enzyme environment and (2) a high β (hydrogen bond accepting ability) means the solvent will interact with the water molecules in the enzyme environment, and potentially strip them from the biocatalyst. A comparison of these values (Table S3) shows (2) to have a much lower β value compared to (1), similar to that of diphenyl ether which may explain why this solvent produced polymers with the highest molecular weights. However, (2) is a solid at 25°C and the KAT parameters had to be measured at 60°C (Citation25), making it difficult to compare to other β values.
The Cygnet class of solvents (2–4) differs from precursor (1) due to the functional group interconversion from ketone to ketal. Ketals generally possess a smaller dipole moment than ketones. The Cygnets are more electronegative than (1), as calculated with σ-profiles using the COSMO-RS method (a). Compound (4) has a greater charge density (e/Å2) on the ketal oxygen atoms than (2), and both have more lipophilic surface area than (1) due to the introduction of ketal methylene groups. For reference, the work up solvent MeTHF has less negatively charged surface area than (2) or (4) by virtue of only having a single oxygen atom, but the charge density is greater. The Cygnet solvents have a ketal and an acetal moiety (4 oxygen atoms in total) that compete to withdraw electron density from the molecule, resulting in lower charge density than an ether. The σ-profile of DPE indicates a different character to the Cygnet solvents (a). DPE is in fact more similar to toluene than an ether due to electron donation into the π-electron system. Hansen Solubility Parameters (HSP, see Figure S28 in ESI) imply that compounds (2) & (4) are more similar to DPE than they are to (1). HSP relate to solubility, and dissolving the reactants is essential. Keeping the polymer in solution will help produce longer polymers as it was observed in this work. The surface charge information contained in a σ-profile can be used to calculate the chemical potential arising from the introduction of a charge into solution. The resulting σ-potential can be compared between solvents to identify similar behaviors (b). There is a favorable interaction between positive charges and Cygnets (2) and (4), greater than for (1). Conversely, DPE is not a good medium for charged species. This general stabilization of electronic charges does not transfer to specific interactions (which may be more relevant), for the Kamlet–Taft parameters indicate (2) forms weaker hydrogen bonds than (1) (since it has a smaller β value). Again, this makes (2) more similar to DPE which benefits the enzymatic synthesis process. The surface charges of each solvent used to carry out the reactions are given in (c) where red indicates negative surface charges with yellow representing a weak negative charge. Blue portions of the molecules represent positive surface charges, and green is a neutral surface charge.
Figure 4. Determination of the electronic properties of the reaction solvents using the COSMO-RS software. (a) Sigma-profiles of the solvents; (b) Sigma-potentials of the solvents; (c) COSMO-surfaces of the solvents.
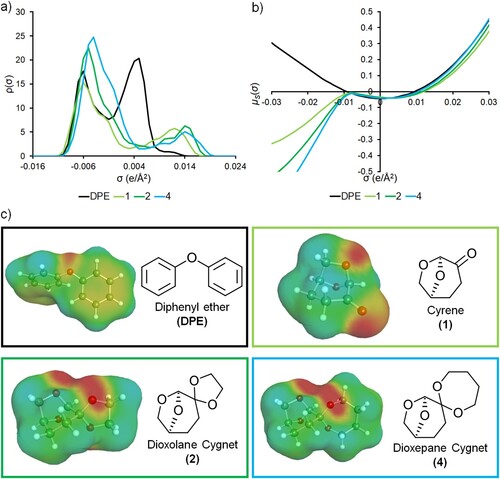
Overall, although (1) and (2) were similar in terms of percentage yield, use of (2) in synthesis gave a much higher Mw (Table S2 and Figures S10–S15). The use of methanol as an anti-solvent instead of water also increased Mw and Mn. The BDO based polymer synthesized in (2) and worked up in (1) and methanol gave a Mw/Mn of 38.1/22.1 kDa, the highest observed in this work. While (4) could not compare with the Mw/Mn yielded by (2), use of MeTHF as a workup solvent gave the highest Mn and Mw seen for this solvent.
Thermal analysis of polymers
DSC analysis (Figures S18–S27) show that all polymers have a crystalline character. BDO-based polymers have a double melting peak on their second heating step only (a), which has been observed before for many different polyesters (Citation49–51). This multiple melting behavior has a number of explanations, but the one most commonly accepted is the melting of smaller crystals, followed by recrystallisation and subsequent melting of these larger crystals at a higher temperature(Citation52), proof of which has been seen in poly(butylene naphthalate) (Citation53). In the Tm of BDO and ODO-based polymers were plotted for comparison, showing (1) produced polymers with the highest Tm while (4) produced polymers with the lowest (43 °C for BDO-based and 61 °C for ODO-based). Despite these little variations, there was not a huge range in the ΔTm, as all polymers were within 6°C and 11°C of each other for ODO and BDO-based polymers respectively (Table S7).
Figure 5. Thermal analysis of those polymers worked up using Cyrene (1)/water in all three reaction solvents. Graphed is the DSC analysis for (a) BDO based polymers and (b) ODO based polymers (second heating cycle). Also shown is TGA analysis for (c) BDO based polymers, and (d) ODO based polymers. (1) is show in light green, (2) in dark green and (4) in blue.
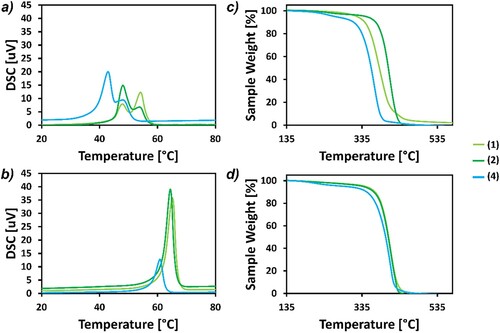
Looking at the TGA data, ODO-based polymers had less variation in Td compared to BDO based polymers (Figures S16 and S17), possibly because the molecular weights of these polymers were more consistent (). ODO based polymers generally had higher Td with the exception of those BDO based polymers synthesized in (2), which may again be due to the high molecular weights obtained for these polymers. Reaction 15, which had the highest molecular weight seen in this work, also had the highest Td5 and Td10 out of all polymers tested at 362°C and 376°C respectively (Table S6). A previous work observed very low decomposition temperatures for BDO-based polymers (although using a different diester) which was attributed to lower molecular weights (Citation19). Following the trend observed for the DSC data, polymers worked up in methanol always had a higher Td5. The largest difference was in the BDO based polymer synthesized in (1), comparing the methanol and water workups resulted in a difference of 23°C. (4) produced polymers had the lowest Td5 for both diols used (Table S6).
Polycondensations with levoglucosenone-based monomers
To test that these solvents are applicable to a whole range of enzymatic polycondensations, other monomers were tested under the best conditions found above.
The bio-derived (S)-5-(hydroxymethyl)-3-[(2S,3S)-2-(hydroxymethyl)-5-oxotetrahydrofuran-3-yl]furan-2(5H)-one monomer (also named 2H-HBO-HBO) (a) was selected, as a sustainable alternative to fossil-based monomers and to show the versatility of the Cyrene precursor levoglucosenone as a platform molecule. 2H-HBO-HBO was condensed with acyl chlorides in a previous paper to yield several polyesters with different carbon chain lengths () (Citation29). In this work, we describe for the first time its enzymatic polycondensation in a sustainable system composed of solvent and monomer, that were both obtained from levoglucosenone (c).
Figure 6. (a) Method of synthesizing 2H-HBO-HBO through dimerization of levoglucosenone and the following Baeyer-Villiger oxidation. (b) Reaction of 2H-HBO-HBO with acyl chloride to form corresponding polyester (Citation29). (c) Enzymatic polycondensation with diesters performed in this work.
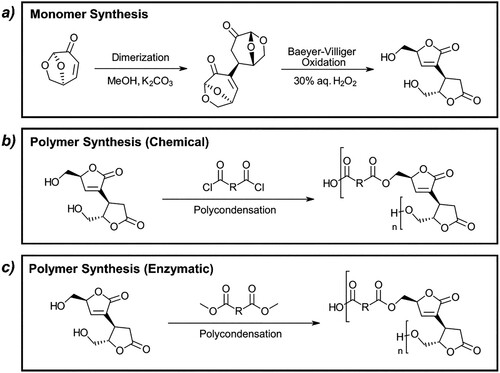
In the aforementioned previous work on the poly(2H-HBO-HBO adipate) polymer, a yield of 38% was obtained, and the Mn was also rather limited, reaching molecular weights of around 3.4 kDa. Attempts to improve this reaction with CaLB as the biocatalyst, dicarboxylic acids methyl esters as an alternative to acyl chlorides and reactions in bulk or using (1) as the solvent were performed. As it is possible to observe from , use of (2) and (4) were successful yielding some polymers, albeit the yield from (2) is extremely low. Cyrene underwent side reactions in the presence of 2H-HBO-HBO, presumably through its ketone group, as (2) and (4) did not.
Figure 7. (a) The yield, and (b) the number average and weight average molecular weights, of poly(2-HBO-HBO adipate), enzymatically synthesized using Cyrene, (2) and (4) as the reaction solvent and precipitated in water. * = indicates polymerization reaction did not occur.
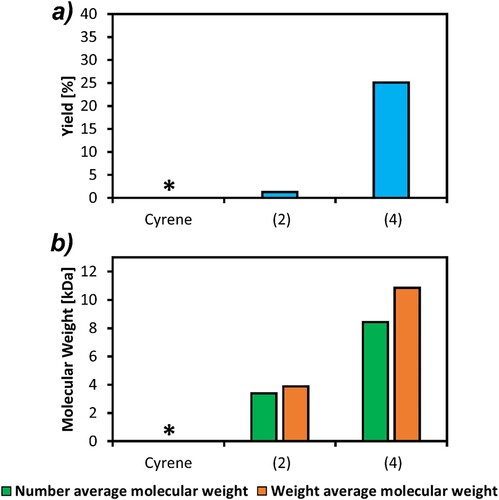
In contrast to the results obtained from the octanediol and butanediol based polymers, (4) was by far the best solvent for the synthesis of poly(2H-HBO-HBO adipate), in terms of both yield and molecular weight. The highest yield obtained in this work was 25% (a), which falls short of the 38% obtained when reacting with acyl chlorides. On the other hand, there is a huge improvement in the molecular weight, as a Mn of 8.4 kDa was achieved, the highest seen for this polymer.
This could be because poly(2HBO adipate) polymer is more soluble in (4) compared to (2). Using the DIY function in HSPiP software, the Hansen solubility parameters of several poly(2HBO adipate) oligomers of various molecular weights were calculated (Table S10). The 2H-HBO-HBO monomer is closer to (2) than (4) in HSPiP space; it has a calculated relative energy distance (RED) of 1.66 versus 1.9 (Table S11). However, when the monomer reacts with dimethyl adipate to form short chain oligomers, the RED from (4) decreases to 1.87, while the RED from (2) increases to 2.08. This trend continues as the molecular weight of the oligomer increases, which can be seen in Table S11, although the difference between the REDs is slight.
This is the first report of a fully Cyrene-based system where both the polymer and the reaction media are derived from the cellulose-based levoglucosenone therefore consisting in an extremely novel and promising green chemistry approach for the synthesis of bio-based polyesters.
Conclusions
Novel Cyrene derivatives developed in this study are extremely promising candidates as solvents for enzymatic polycondensation reactions. The solvents synthesis method has been improved through the use of phosphoric acid as an alternative to KSF 200 and the elimination of toluene from the work up procedure. Enzymatic synthesis reactions were also greened up completely removing chloroform from the work up procedure and substituting it with water or the green solvent methyl-tetrahydrofuran. In fact, in these model reaction, dioxolane Cygnet has been shown to preserve enzyme activity that allowed the obtainment of polymers with a Mw of up to 38 kDa, a vast improvement when compared to the more polar Cyrene. Finally, as a completely environmentally friendly system where all reaction components are obtained from Nature (biocatalyst, solvent and polymer), the enzymatic polycondensation of 2H-HBO-HBO, a bicyclic diol synthesized from levoglucosenone, has been successfully demonstrated in Cyrene as the first fully cellulose-derived polymer-solvent synthetic system.
Author’s contributions
C.M.W. and A.P. conducted the solvents and polymer synthesis experiments and the characterizations. R.M.E. conducted the solvents synthesis and characterization. A.C.W. ran the XRD characterization of the solvents. J.S. ran the COSMOtherm solvent simulations. L.M.M.M. and F.A. designed and optimized the sustainable synthetic procedure to 2H-HBO-HBO; S.F. performed the synthesis of the 2H-HBO-HBO monomer and the characterization of the resulting polymers. C.M.W., R.M.E. and A.P. wrote the manuscript. All authors corrected the manuscript. G.M.G. and A.P. acquired the funding. A.P. and R.M.E. designed and supervised the work.
Acknowledgments
Thanks to Mr Karl Heaton of the Mass Spectrometry Service, Department of Chemistry, University of York for obtaining ESI data.
Supplemental Material
Download MS Word (8.3 MB)Data availability statement
The authors confirm that the data supporting the findings of this study are available within the article or its supplementary materials.
Disclosure statement
No potential conflict of interest was reported by the author(s).
Additional information
Funding
References
- Geyer, R.; Jambeck, J.R.; Law, K.L. Production, Use, and Fate of All Plastics Ever Made. Sci. Adv. 2017, 3 (7), e1700782. doi:10.1126/sciadv.1700782.
- Sigler, M. The Effects of Plastic Pollution on Aquatic Wildlife: Current Situations and Future Solutions. Water Air Soil Pollut. 2014, 225 (11), 2184. doi:10.1007/s11270-014-2184-6.
- Sharma, S.; Chatterjee, S. Microplastic Pollution, a Threat to Marine Ecosystem and Human Health: A Short Review. Environ Sci Pollut Res 2017, 24 (27), 21530–21547. doi:10.1007/s11356-017-9910-8.
- Hwang, K.-R.; Jeon, W.; Lee, S.Y.; Kim, M.-S.; Park, Y.-K. Sustainable Bioplastics: Recent Progress in the Production of Bio-Building Blocks for the Bio-Based Next-Generation Polymer PEF. Chem. Eng. J. 2020, 390, 124636. doi:10.1016/j.cej.2020.124636.
- Nakajima, H.; Dijkstra, P.; Loos, K. The Recent Developments in Biobased Polymers Toward General and Engineering Applications: Polymers That Are Upgraded from Biodegradable Polymers,: Analogous to Petroleum-Derived Polymers, and Newly Developed. Polymers. (Basel) 2017, 9, 523. doi:10.3390/polym9100523.
- Roda, A.; Matias, A.A.; Paiva, A.; Duarte, A.R.C. Polymer Science and Engineering Using Deep Eutectic Solvents. Polymers. (Basel) 2019, 11 (5), 912. doi:10.3390/polym11050912.
- Boyère, C.; Jérôme, C.; Debuigne, A. Input of Supercritical Carbon Dioxide to Polymer Synthesis: An Overview. Eur. Polym. J. 2014, 61, 45–63. doi:10.1016/j.eurpolymj.2014.07.019.
- DiLauro, A.M.; Robbins, J.S.; Phillips, S.T. Reproducible and Scalable Synthesis of End-Cap-Functionalized Depolymerizable Poly(Phthalaldehydes). Macromolecules 2013, 46 (8), 2963–2968. doi:10.1021/ma4001594.
- Polgar, L.M.; van Duin, M.; Broekhuis, A.A.; Picchioni, F. Use of Diels–Alder Chemistry for Thermoreversible Cross-Linking of Rubbers: The Next Step Toward Recycling of Rubber Products? Macromolecules 2015, 48 (19), 7096–7105. doi:10.1021/acs.macromol.5b01422.
- Schneiderman, D.K.; Vanderlaan, M.E.; Mannion, A.M.; Panthani, T.R.; Batiste, D.C.; Wang, J.Z.; Bates, F.S.; Macosko, C.W.; Hillmyer, M.A. Chemically Recyclable Biobased Polyurethanes. ACS Macro Lett. 2016, 5 (4), 515–518. doi:10.1021/acsmacrolett.6b00193.
- Shieh, P.; Zhang, W.; Husted, K.E.L.; Kristufek, S.L.; Xiong, B.; Lundberg, D.J.; Lem, J.; Veysset, D.; Sun, Y.; Nelson, K.A.; Plata, D.L.; Johnson, J.A. Cleavable Comonomers Enable Degradable,: Recyclable Thermoset Plastics. Nature 2020, 583 (7817), 542–547. doi:10.1038/s41586-020-2495-2.
- Anastas, P.; Eghbali, N. Green Chemistry: Principles and Practice. Chem. Soc. Rev. 2010, 39 (1), 301–312. doi:10.1039/B918763B.
- Saleh, H. E.-D. M.; Koller, M. Introductory Chapter: Principles of Green Chemistry; IntechOpen, 2018. doi:10.5772/intechopen.71191.
- Dubé, M.A.; Salehpour, S. Applying the Principles of Green Chemistry to Polymer Production Technology. Macromol. React. Eng. 2014, 8 (1), 7–28. doi:10.1002/mren.201300103.
- Sheldon, R.A.; Woodley, J.M. Role of Biocatalysis in Sustainable Chemistry. Chem. Rev 2018, 118 (2), 801–838. doi:10.1021/acs.chemrev.7b00203.
- Byrne, F.P.; Jin, S.; Paggiola, G.; Petchey, T.H.M.; Clark, J.H.; Farmer, T.J.; Hunt, A.J.; Robert McElroy, C.; Sherwood, J. Tools and Techniques for Solvent Selection: Green Solvent Selection Guides. Sustain Chem Process 2016, 4 (1), 7. doi:10.1186/s40508-016-0051-z.
- Understanding REACH - ECHA. https://echa.europa.eu/regulations/reach/understanding-reach (accessed August 19, 2021).
- Azim, H.; Dekhterman, A.; Jiang, Z.; Gross, R.A. Candida Antarctica Lipase B-Catalyzed Synthesis of Poly(Butylene Succinate): Shorter Chain Building Blocks Also Work. Biomacromolecules 2006, 7 (11), 3093–3097. doi:10.1021/bm060574h.
- Pellis, A.; Comerford, J.W.; Weinberger, S.; Guebitz, G.M.; Clark, J.H.; Farmer, T.J. Enzymatic Synthesis of Lignin Derivable Pyridine Based Polyesters for the Substitution of Petroleum Derived Plastics. Nat. Commun. 2019, 10 (1), 1762. doi:10.1038/s41467-019-09817-3.
- Zhenova, A.; Pellis, A.; Milescu, R.A.; McElroy, C.R.; White, R.J.; Clark, J.H. Solvent Applications of Short-Chain Oxymethylene Dimethyl Ether Oligomers. ACS Sustainable Chem. Eng 2019, 7 (17), 14834–14840. doi:10.1021/acssuschemeng.9b02895.
- Pellis, A.; Byrne, F.P.; Sherwood, J.; Vastano, M.; Comerford, J.W.; Farmer, T.J. Safer Bio-Based Solvents to Replace Toluene and Tetrahydrofuran for the Biocatalyzed Synthesis of Polyesters. Green Chem. 2019, 21 (7), 1686–1694. doi:10.1039/C8GC03567A.
- Sherwood, J.; De bruyn, M.; Constantinou, A.; Moity, L.; McElroy, C.R.; Farmer, T.J.; Duncan, T.; Raverty, W.; Hunt, A.J.; Clark, J.H. Dihydrolevoglucosenone (Cyrene) as a Bio-Based Alternative for Dipolar Aprotic Solvents. Chem. Commun 2014, 50 (68), 9650–9652. doi:10.1039/C4CC04133J.
- Mouterde, L.M.M.; Allais, F.; Stewart, J.D. Enzymatic Reduction of Levoglucosenone by an Alkene Reductase (OYE 2.6): A Sustainable Metal- and Dihydrogen-Free Access to the Bio-Based Solvent Cyrene®. Green Chem. 2018, 20 (24), 5528–5532. doi:10.1039/C8GC03146K.
- Circa Group. Technology. Circa Group. https://circa-group.com/technology (accessed August 18, 2021).
- Alves Costa Pacheco, A.; Sherwood, J.; Zhenova, A.; McElroy, C.R.; Hunt, A.J.; Parker, H.L.; Farmer, T.J.; Constantinou, A.; De bruyn, M.; Whitwood, A.C.; Raverty, W.; Clark, J.H. Intelligent Approach to Solvent Substitution: The Identification of a New Class of Levoglucosenone Derivatives. ChemSusChem. 2016, 9 (24), 3503–3512. doi:10.1002/cssc.201600795.
- Guajardo, N.; Domínguez de María, P. Assessing Biocatalysis Using Dihydrolevoglucosenone (CyreneTM) as Versatile Bio-Based (Co)Solvent. Molecular Catalysis 2020, 485, 110813. doi:10.1016/j.mcat.2020.110813.
- Gonzalo, G. de. Biocatalysed Reductions of α-Ketoesters Employing CyreneTM as Cosolvent. Biocatal. Biotransform. 2021, 1–6. doi:10.1080/10242422.2021.1887150.
- Milescu, R.A.; Zhenova, A.; Vastano, M.; Gammons, R.; Lin, S.; Lau, C.H.; Clark, J.H.; McElroy, C.R.; Pellis, A. Polymer Chemistry Applications of Cyrene and Its Derivative Cygnet 0.0 as Safer Replacements for Polar Aprotic Solvents. ChemSusChem. 2021, 14, 3367. doi:10.1002/cssc.202101125.
- Diot-Néant, F.; Mouterde, L.; Fadlallah, S.; Miller, S.A.; Allais, F. Sustainable Synthesis and Polycondensation of Levoglucosenone-Cyrene-Based Bicyclic Diol Monomer: Access to Renewable Polyesters. ChemSusChem. 2020, 13 (10), 2613–2620. doi:10.1002/cssc.202000680.
- CrysAlisPro, Oxford Diffraction Ltd. CrysAlisPro, Oxford Diffraction Ltd.
- Empirical absorption correction using spherical harmonics, implemented in SCALE3 ABSPACK scaling algorithm within CrysAlisPro software, Oxford Diffraction Ltd.: Empirical Absorption Correction Using Spherical Harmonics, Implemented in SCALE3 ABSPACK Scaling Algorithm within CrysAlisPro Software, Oxford Diffraction Ltd.
- “Olex2” crystallography software, J. Appl. Cryst., 2009, 42, 339–341. “Olex2” crystallography software, J. Appl. Cryst., 2009, 42, 339–341
- “SHELXS-97” - program for structure solution. G.M. Sheldrick,: Acta Cryst. 2008, A64, 112-122. “SHELXS-97” - Program for Structure Solution. G.M. Sheldrick, Acta Cryst. 2008, A64, 112-122.
- SHELXT – Integrated space-group and crystal-structure determination G. M. Sheldrick,: Acta Cryst. 2015, A71, 3-8. SHELXT – Integrated Space-Group and Crystal-Structure Determination G. M. Sheldrick, Acta Cryst. 2015, A71, 3-8.
- “Crystal structure refinement with SHELXL” G.M. Sheldrick,: Acta Cryst. 2015, C71, 3-8. “Crystal Structure Refinement with SHELXL” G.M. Sheldrick, Acta Cryst. 2015, C71, 3-8.
- Zhao, H. Enzymatic Polymerization to Polyesters in Nonaqueous Solvents. Methods Enzymol. 2019, 627, 1–21. doi:10.1016/bs.mie.2019.03.002.
- Feder, D.; Gross, R.A. Exploring Chain Length Selectivity in HIC-Catalyzed Polycondensation Reactions. Biomacromolecules 2010, 11 (3), 690–697. doi:10.1021/bm901272r.
- Mahapatro, A.; Kalra, B.; Kumar, A.; Gross, R.A. Lipase-Catalyzed Polycondensations: Effect of Substrates and Solvent on Chain Formation,: Dispersity, and End-Group Structure. Biomacromolecules 2003, 4 (3), 544–551. doi:10.1021/bm0257208.
- Pellis, A.; Weinberger, S.; Gigli, M.; Guebitz, G.M.; Farmer, T.J. Enzymatic Synthesis of Biobased Polyesters Utilizing Aromatic Diols as the Rigid Component. Eur. Polym. J. 2020, 130, 109680. doi:10.1016/j.eurpolymj.2020.109680.
- Bousfield, T.W.; Pearce, K.P.R.; Nyamini, S.B.; Angelis-Dimakis, A.; Camp, J.E. Synthesis of Amides from Acid Chlorides and Amines in the Bio-Based Solvent CyreneTM. Green Chem. 2019, 21 (13), 3675–3681. doi:10.1039/C9GC01180C.
- Milescu, R.A.; Segatto, M.L.; Stahl, A.; McElroy, C.R.; Farmer, T.J.; Clark, J.H.; Zuin, V.G. Sustainable Single-Stage Solid–Liquid Extraction of Hesperidin and Rutin from Agro-Products Using Cyrene. ACS Sustainable Chem. Eng 2020, 8 (49), 18245–18257. doi:10.1021/acssuschemeng.0c06751.
- De bruyn, M.; Budarin, V.L.; Misefari, A.; Shimizu, S.; Fish, H.; Cockett, M.; Hunt, A.J.; Hofstetter, H.; Weckhuysen, B.M.; Clark, J.H.; Macquarrie, D.J. Geminal Diol of Dihydrolevoglucosenone as a Switchable Hydrotrope: A Continuum of Green Nanostructured Solvents. ACS. Sustain. Chem. Eng. 2019, 7 (8), 7878–7883. doi:10.1021/acssuschemeng.9b00470.
- Pellis, A.; Comerford, J.W.; Maneffa, A.J.; Sipponen, M.H.; Clark, J.H.; Farmer, T.J. Elucidating Enzymatic Polymerisations: Chain-Length Selectivity of Candida Antarctica Lipase B Towards Various Aliphatic Diols and Dicarboxylic Acid Diesters. Eur. Polym. J. 2018, 106, 79–84. doi:10.1016/j.eurpolymj.2018.07.009.
- Abbott, S.; Yamamoto, H. Hansen Solubility Parameters in Practice (HSPiP); 5th ed.; Hansen-Solubility.com
- Li, C.; Tan, T.; Zhang, H.; Feng, W. Analysis of the Conformational Stability and Activity of Candida Antarctica Lipase B in Organic Solvents. J. Biol. Chem. 2010, 285 (37), 28434–28441. doi:10.1074/jbc.M110.136200.
- Kumar, A.; Gross, R.A. Candida Antartica Lipase B Catalyzed Polycaprolactone Synthesis: Effects of Organic Media and Temperature. Biomacromolecules 2000, 1 (1), 133–138. doi:10.1021/bm990510p.
- Gorman, L.A.S.; Dordick, J.S. Organic Solvents Strip Water off Enzymes. Biotechnol. Bioeng. 1992, 39 (4), 392–397. doi:10.1002/bit.260390405.
- Iemhoff, A.; Sherwood, J.; McElroy, C.R.; Hunt, A.J. Towards Sustainable Kinetic Resolution, a Combination of Bio-Catalysis,: Flow Chemistry and Bio-Based Solvents. Green Chem. 2018, 20 (1), 136–140. doi:10.1039/C7GC03177G.
- Tan, S.; Su, A.; Li, W.; Zhou, E. New Insight Into Melting and Crystallization Behavior in Semicrystalline Poly(Ethylene Terephthalate). J. Polym. Sci., Part B: Polym. Phys. 2000, 38 (1), 53–60. doi:10.1002/(SICI)1099-0488(20000101)38:1<53::AID-POLB6>3.0.CO;2-G.
- Yasuniwa, M.; Satou, T. Multiple Melting Behavior of Poly(Butylene Succinate). I. Thermal Analysis of Melt-Crystallized Samples. Journal of Polymer Science Part B: Polymer Physics 2002, 40 (21), 2411–2420. doi:10.1002/polb.10298.
- Yasuniwa, M.; Tsubakihara, S.; Murakami, T. High-Pressure DTA of Poly(Butylene Terephthalate),: Poly(Hexamethylene Terephthalate), and Poly(Ethylene Terephthalate). J. Polym. Sci., Part B: Polym. Phys. 2000, 38 (1), 262–272. doi:10.1002/(SICI)1099-0488(20000101)38:1<262::AID-POLB28>3.0.CO;2-5.
- Yasuniwa, M.; Tsubakihara, S.; Sugimoto, Y.; Nakafuku, C. Thermal Analysis of the Double-Melting Behavior of Poly(L-Lactic Acid). J. Polym. Sci., Part B: Polym. Phys. 2004, 42 (1), 25–32. doi:10.1002/polb.10674.
- Yasuniwa, M.; Tsubakihara, S.; Fujioka, T. X-Ray and DSC Studies on the Melt-Recrystallization Process of Poly(Butylene Naphthalate). Thermochim. Acta 2003, 396 (1), 75–78. doi:10.1016/S0040-6031(02)00531-2.