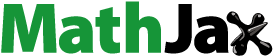
ABSTRACT
Gold nanoparticles (AuNPs) have a wide range of applications due to their optoelectronic properties. Particularly, biological synthesis of AuNPs highlights due to the advantages related to the environmental care and the stabilization provided by capping biomolecules during biosynthesis. The antimicrobial activity of AuNPs was reported, however, their activity against resistant structures such as bacterial endospores have not been evaluated. This work shows the extracellular biosynthesis of AuNPs using Epicoccum nigrum (Au1-8) throughout an ecofriendly, simple, and efficient method. Physicochemical characterization revealed that stable quasi-spherical AuNPs in a range size of 2 to 30 nm, with crystal structure and metallic Au0 composition were obtained. The AuNPs sporicidal activity against Bacillus subtilis endospores was evaluated by its exposure to 300 mg/L AuNPs during 48 h, which produced a decrease of 90.6% of the endospore germination. The analysis of the endospore-AuNP interaction by scanning electron microscopy (SEM) and infrared (IR) spectroscopy revealed injury of the endospore coat and lysis. These results are promising for the advance in the development of low-aggressive agents used for environmental decontamination regimens against pathogenic bacterial spores, or even as an alternative for the treatment of illnesses produced by endospore-former causative agents of food spoilage/poisoning and human diseases.
1. Introduction
Metal nanoparticles (MNPs) are defined as particles with diameter of 1 to 100 nanometers that exhibit unique properties that differ from those of the same bulk metals (Citation1). In particular, gold nanoparticles (AuNPs) are highlighted by its biocompatibility, strong adsorption ability, optoelectronic and chemical properties which make it easy for surface modification (Citation2). The AuNPs properties are highly correlated to the shape and size, which determine a wide variety of applications in life sciences, detection of microtargeted drug delivery, medical diagnoses, images, and therapy (Citation3–6). A variety of techniques have been adopted for AuNPs synthesis, these include physical, chemical and biological methods to obtain AuNPs with different dimensions, stability and surface properties for functionalization (Citation7, Citation8). Particularly, the biological synthesis of AuNPs using different biological organisms (also called biosynthesis) has emerged as an eco-friendly, low-energy consumer and cost-effective method to obtain AuNPs with high stability and a diversity of sizes and shapes (Citation4, Citation9, Citation10). An outstanding method for AuNPs biosynthesis is fungal biosynthesis, a process with advantages such as the high metal tolerance of fungi, the extracellular redox protein secretion for reduction of metal ions to insoluble forms, easy scale-up, and the production of capping/stabilizing agents (i.e. proteins, peptides, ligninases/laccases, polysaccharides, organic acids, amino acids), without the needed of exogenous chemicals (Citation9, Citation11, Citation12).
The antibacterial activity of AuNPs has been widely reported (Citation13); the most of evidence suggests that whereas bare AuNPs have no significant antibacterial effect, the AuNPs conjugated with antibiotics or drugs are effective against bacterial cell growth (Citation14, Citation15). However, some studies indicated that non-conjugated AuNPs chemically or biologically synthesized, possess antibacterial activity against a variety of both Gram-positive and Gram-negative bacteria and bacterial biofilms (Citation16, Citation17). These contradictory results seem to be related to differences of the bacterial cell wall structure and composition, and the size, shape and dose of AuNPs used (Citation17). The mechanisms proposed for the antibacterial activity of AuNPs include: (1) disruption of the membrane potential through the inhibition of the ATP synthase activity and the interaction with thiol groups of enzymes of the respiratory chain, (2) inhibition of the tRNA binding site in ribosomes, (3) pore formation during translocation across bacterial cell membrane, and (4) mechanical deformation and rupture of the bacterial membrane (Citation18, Citation19). Interestingly, unlike it has been documented for most bactericidal nanomaterials, the generation of ROS or ROS-related process seems to be unrelated with the antibacterial effect of AuNPs (Citation18). Although the antibacterial activity of AuNPs has been widely evaluated, studies about its efficacy against highly resistant bacterial structures such as endospores, to our knowledge, have not been evaluated. To this respect, a few studies were carried out with MNPs and suggest that their sporicidal activity strongly depends on the MNPs properties and the method used for their synthesis. For example, Tehri et al. (Citation21), determined that 5–15 nm silver nanoparticles (AgNPs) obtained by biosynthesis with leaves extract of Litchi chinensis inhibit 61.6% of the endospore viability of Bacillus subtilis at 200 mg/mL after 8 h of the exposition to AgNPs, whereas Au/CuS NPs were non-effective against Bacillus anthracis endospores (Citation20, Citation21). This unexplored field opens the opportunity for the study of the MNPs activity against bacterial endospores and its application as alternative sporicidal agents to deal with resistant and prevalent bacterial endospores, especially those of pathogenic species (i.e. B. anthracis, Bacillus cereus, Clostridium tetani, Clostridium botulinum, Clostridium perfringens) which have the ability to germinate to produce infections and/or poisoning/intoxications (Citation22). In this work, a fungal-biosynthetic method to obtain stable AuNPs effective against B. subtilis endospores is described. The possible mechanism involved in damage produced by AuNPs is discussed.
2. Materials and methods
2.1. Fungi and bacterial strains
The fungal strain Au1-8 used in this work was isolated in the presence of AuCl3 at 1.0 mM from mining tails located at Zacatecas, México (22°47′01.4″ N 102°36′21″ W and 2426 m.a.s.l.). The spore-former B. subtilis 168 reference strain was used for the sporicidal assays.
2.2. Identification and morphological characterization of the AuNPs producer fungi
The phenotypic characterization of the Au1-8 fungal isolate was based on description of colony and morphology characteristics in three different culture media: PDA (Potato Dextrose Agar) (BD DifcoTM); SDA (Sabouraud Dextrose Agar) (BD DifcoTM) and Czapek Dox Agar (BD DifcoTM). The cell morphology was observed in fungal microcultures by light microscopy using a cotton blue solution (1%) as a contrast dye. The genus of the Au1-8 strain was established accordingly to morphological characteristics previously described (Citation23). The phenotypic identification was complemented by a molecular approach by sequencing of the internal transcribed spacers (ITSs) of the rDNA genes. Briefly, 100 ng of genomic DNA was used for amplification of the ITSs regions by PCR under previously reported conditions with primers ITS1 (5’ TCCGTAGGTGAACCTGCGG 3’ corresponding to the end of the 18S gen) and ITS4 (5’ TCCTCCGCTTATTGATATGC 3’ corresponding to the initial region of the 28S gene) (Citation24). The PCR products were purified with the Wizard SV Gel and PCR Clean-Up System Kit (Promega, Madison, U.S.A.), and sequenced using the same primers used in PCR. The sequences were assembled and edited using the DNASTAR Lasergene v.7.1 software and compared to the ITSs rDNA of fungal sequences deposited in the GeneBank database of the National Center for Biotechnology Information (NCBI) server. Sequence alignments were performed using the BLASTN program of the NCBI server, using the MEGABLAST algorithm for highly similar sequences and default parameters to determine the sequence identity percentages.
2.3. Fungal Au-tolerance and biosynthesis of the AuNPs
To test the fungal tolerance to gold ions (Au + 3), the minimum inhibitory concentration (MIC) was determined. Briefly, an agar plug of a fungal colony was inoculated onto PDA plates supplemented with increased concentrations of AuCl3 (0, 1.5, 3.0, 4.5 and 6.0 mM) and incubated at 28°C for 7 days. The lowest concentration of metal salt that prevented the growth was recorded as MIC. The fungal extracellular biosynthesis of AuNPs, was established based on previously described protocols for metal-NPs biosynthesis and optimized by modification of biosynthesis parameters (Citation25). Briefly, the mycelium of a PDA culture with seven days of growth was collected with 2 mL of sterile water, and 0.2 mL of the water-suspended mycelium was used to inoculate 50 mL of PDB (Potato Dextrose Broth)(BD DifcoTM) and incubated at 28°C and 180 rpm for 72 h. The mycelium was separated from PDB media by vacuum filtration under sterile conditions and the free-fungal PDB filtrate was supplemented with 1.0 mM of AuCl3 and incubated in dark conditions at 28°C and 180 rpm for 24 h. Simultaneously, media culture without fungi inoculant was treated under the above conditions described and used as a control of AuNPs synthesis, whereas a free-fungal PDB filtrate without the addition of the metal salt was obtained at the same conditions to be used as blank in the AuNPs UV-Vis and as a control for the IR-spectroscopy analyses. Bioreduction of gold ions was monitored spectroscopically in aliquots obtained from the fungal filtrates containing the biosynthesized AuNPs and in the control sample (PDB media without fungi inoculant), by measurement of light absorption at the UV-Vis range using a spectrophotometer (Perkin Elmer, model Lambda 35) at a wavelength range between 200 and 900 nm. The data were processed in the Origin 8.0 software and the absorption spectra were obtained.
The gold concentration associated with the biosynthesized AuNPs was estimated by measuring the concentration of the non-reduced gold ions in AuNPs that remained soluble in the supernatant of the free-fungal PDB-filtrate. The AuNPs suspension was centrifuged (12,000 rpm, 30 min) to pellet the AuNPs and the supernatant was analyzed by Energy dispersive X-Ray fluorescence in an EDXRF Spectrometer (Rigaku® NEX QC + QuantEZ) to determine the concentration of non-reduced gold ions. The concentration of gold associated with AuNPs was calculated with the equation:
where CAuNPs is the gold concentration associated with the AuNPs in the suspension, CAu ions are the total concentration of gold ions used for the biosynthesis (1 mM), and CAuSN is the concentration of non-reduced gold ions that remained in the supernatant after the centrifugation of AuNPs (determined by EDXRF).
2.4. Purification, stability and recycle of biosynthesized AuNPs
Biosynthesized AuNPs were separated from the media used for biosynthesis (free fungal PDB filtrate), roughly water washed, and resuspended in water for subsequent physicochemical characterization and for the assays to evaluate its sporicidal activity. Briefly, 50 mL of AuNPs suspensions were centrifuged at 12,000 rpm for 30 min, the supernatant was eliminated and the pellet containing AuNPs was resuspended in 25 mL of sterile deionized water and centrifugated at 12,000 rpm for 30 min until complete three washes. Finally, the AuNPs were resuspended in 10 mL of sterile deionized water for utilization in the sporicidal assays, analysis of the stability and recyclability of AuNPs. To evaluate the stability of AuNPs over time, a water-suspension of AuNPs was analyzed by UV-Vis spectroscopy after 12 months of its biosynthesis. The stability was also evaluated by UV-Vis spectroscopy in AuNPs suspensions treated under stream-sterilization conditions (15 min at 121°C and 15 psi). To evaluate the recyclability of the AuNPs, the gold recovery from a 12-months AuNPs suspension was carried out using a previously described method (Citation26). Briefly, 1 mL of the AuNPs suspension was treated for precipitation of AuNPs with NaOH to a final concentration of 1M. After 24 h a black precipitate was formed and the supernatant was discarded. The black solid was washed and added to 1 mL of H2O2 (6M) and HCl (1M) solution. After 15 min, the solid was dissolved and the solution became light yellow indicative of the formation of HAuCl4, finally, the concentration of gold ions was determined by EDXRF.
2.5. Characterization of AuNPs synthesized by fungi
The functional groups of the fungal biomolecules associated with the biosynthesis process of AuNPs were analyzed by Infrared (IR) spectroscopy. The fungal filtrates containing the biosynthesized AuNPs and the control (PDB-filtrate without metallic salt), were analyzed in an IRTracer-100 (Shimadzu) Spectrophotometer using PDB media as a blank to subtract those signals produced by PDB-media. The data were recorded for wavenumbers ranging from 400 to 4000 cm−1, with a scan rate of 0.2 cm/sec and resolution of 4 cm−1. The data were processed in the Origin 8.0 software for obtaining the IR spectra.
To reveal the crystalline structure, size, and elemental chemical composition of the biosynthesized AuNPs, the purified AuNPs suspension was analyzed by UV-Vis, X-ray Diffraction (XRD), Scanning Transmission Electron Microscopy (STEM), high-resolution Transmission Electron Microscopy (HRTEM), and Energy-dispersive X-ray Spectroscopy (EDX). The TEM and STEM analyses were performed in a JEM-2200FS microscope that operates at 200 kV of acceleration voltage and has attached a NORAN spectrometer. For the XRD analysis, a purified AuNPs water-suspension and the fungal PDB-filtrate without the addition of metal (as a negative control of biosynthesis) were freeze-dried lyophilized and ground to obtain a dry/fine powder of the samples that were analyzed in a diffractometer SmartLab (Rigaku) with a diffracted beam of CuKα, λ = 1.54060 Å at 40 Kv and 44 mA. The diffraction patterns were obtained with an angular range from 10 to 80° and 2°/min. The code numbers of the crystalline phases detected in the samples were identified using the X’Pert HighScore Plus software (Rigaku PDXL 2.4.2.0 version). For TEM, samples were prepared by placing a drop of purified AuNPs water-suspension on a carbon-coated copper grid, dried at room temperature, and observed. The nanoparticle size was determined by the measurement of 377 individual AuNPs from TEM micrographs. The mean particle size was determined and the size distribution was presented as a histogram plot.
2.6. Preparation of endospore suspension
B. subtilis was grown and sporulated at 37°C in 2xSG medium. The culture containing the endospores was washed six times by repeated centrifugation and suspension in sterile and deionized water at 4°C. To eliminate mother cells, the endospores were suspended in 10 mL of the lysis buffer (EDTA 0.05 M, NaCl 0.1M, pH 7.5) added with 0.1 mL de lysozyme (1 mg/mL), and incubated at 37°C for 30 min. Endospore suspension was centrifuged and washed five times by repeated centrifugation and suspension in cold (4°C) sterile and deionized water. Finally, endospores were resuspended in 1 mL of PBS and the number of endospores obtained was calculated by optical density at 600 nm (1.0 O.D. 600 nm approximately contains 1.5 × 108 endospores/mL) and counted in a Neubauer chamber and stored at 4°C. The cleaned spore preparations were free of vegetative cells and visible cell debris. Germination of endospores was carried out in a 2xSG medium containing 4 mM L-alanine (Citation27).
2.7. Sporicidal effect of fungal biosynthesized AuNPs on bacterial endospores
The effect produced by biogenic AuNPs over B. subtilis endospores was determined quantitatively by viability assays of the endospores exposed to different AuNPs concentrations. Briefly, 1 × 108 endospores were exposed to 0, 100, 200 and 300 mg/L of AuNPs in a final volume of 1 mL deposited by triplicate in 24-wells polystyrene plates (Nunclon Delta Surface, NuncTM), and incubated at room temperature with shaking. The ability of endospores to germinate was evaluated at different times (6, 12, 24 and 48 h) throughout the serial dilution method. Briefly, a volume of 0.1 mL of each dilution of the spore suspensions exposed to the AuNPs, was plated onto 2xSG medium containing 4 mM L-alanine, and incubated at 37°C for 24 h. The colonies formed were counted and colony-forming units per milliliter (CFU/mL) were determined as a measure of endospore germination. The sporicidal effect of AuNPs over bacterial spores was reported as germination percentage concerning the germination percentage of the non-exposed spores to AuNPs. Each condition evaluated was tested in triplicate and experiments were repeated three times.
2.8. Effect of AuNPs over the endospore morphology
For qualitative analysis, the morphology of endospores exposed to the AuNPs was analyzed by Scanning Electron Microscopy (SEM). A suspension containing 1 × 108 endospores/mL was exposed to 100 mg/L of AuNPs and incubated at 200 rpm for 6 h in dark conditions and at room temperature. A volume of 5 mL of this spore suspension was centrifugated at 3000 rpm for 10 min and the spore pellet was fixed in 3% glutaraldehyde in a sodium phosphate buffer 100 mM, at pH 7.4 and 4°C for 2 h; the samples were washed three times with the buffer for 15 min each time. Afterward, the samples were postfixed with 2% OsO4 in buffer for 1 h at 4°C. The samples were gradually dehydrated with absolute ethanol from 30% to 100% for 10 min with each concentration, and washed twice with 100% absolute ethanol, each washing lasting 15 min. The critical point drying process was performed in a Tousimis Samdri-PVT-3D, and the dry samples were mounted to perform the gold sputter coating in a Cressington apparatus model 108 auto and examined in a Quanta 250 FEG Scanning Electron Microscopy. The SEM was adjusted at 10 kV, spot 4, and WD 10 mm, taking the micrographs with an Everhart Thornley Detector (ETD).
2.9. Interaction of endospores with AuNPs by FTIR analysis
wEndospores exposed to purified AuNPs were analyzed by FTIR to evaluate the changes in the endospore’s surface produced by its interaction with the AuNPs. To do this, a suspension containing 1 × 108 endospores/mL was exposed to 100 mg/L of AuNPs and incubated at 200 rpm for 6 h in dark conditions and at room temperature. Volumes of 0.1 mL of both exposed and unexposed endospore suspension to AuNPs, were analyzed in an IRTracer-100 (Shimadzu) Spectrophotometer and data were recorded for wavenumbers ranging from 1000 to 1800 cm−1, with a scan rate of 0.2 cm/sec and resolution of 4 cm−1. Due to the proximity of the amide I band (1640 cm−1) to the signal of the O–H bending vibration modes (located between 1600 and 1680 cm−1), the FTIR spectra were obtained after distilled water was measured as a background, so that automatically the O-H background signal was subtracted from the measured spectrum of the samples. Moreover, dry samples of Bacillus subtilis endospores exposed and not exposed to fungal-biosynthesized AuNPs were prepared over aluminum substrates and characterized by FTIR spectroscopy, under the same conditions and parameters mentioned above. The data were processed in the Origin 8.5 software for obtaining the IR spectra.
2.10. Statistical analysis
For determination of the sporicidal effect of biogenic AuNPs over bacterial endospores, differences between the germination percentage of spores exposed to different concentrations of AuNPs were calculated by performing a one-way ANOVA analysis in the Excel program of the Microsoft software package by comparison of each germination percentage value obtained for each concentration, with the germination percentage value obtained for the untreated endospores and each of the concentrations analyzed. The significance was set at a P < 0.05. An asterisk was used to indicate statistical differences between the AuNPs concentrations tested.
3. Results
3.1. Identification and morphological characterization of the AuNPs producer fungi
The morphological characterization was based on the description of the fungal colonies and microscopic structures of the Au1-8 isolate in different media cultures. In Sabouraud Dextrose Agar, a velvety and wrinkled colony grows within 7 to 10 days of incubation to 28°C. A flower-shaped colony with pink grooves, white irregular edges, and red exudate was observed in the obverse, whereas a flower-shaped colony with irregular and yellow edges was observed in the reverse side of the colony ((A)). In PDA media, a velvety and flat colony with irregular edges was formed in the obverse face, the color of the colony varied from white, gray and brownish according to the maturity time. In the reverse face, the colony showed a radial pattern of growth with irregular edges and a deep red exudate ((B)). In Czapeck Dox Agar, a pale yellow, cotton and flat colony with a regular edge was observed in the obverse, whereas a white and cotton colony with a regular edge was observed in the reverse side of the colony ((C)). Growth of the colony was slow (10 days) and radial with scarce mycelia. No exudates or reproductive structures were observed. The exposition of PDA cultures to low temperatures for two weeks induced the development of conidiophores, production of a deep red exudate and conglomerate or dispersed brown/black sporodochia over the colony surface ((G)).
Figure 1. Colony and microscopic characteristics of the Epicoccum nigrum Au1-8 isolate. Left panel: Colonies (obverse and reverse) in different media cultures are shown: (A) Sabouraud Dextrose Agar, (B) PDA and (C) Czapek Dox Agar. Right panel: PDA microcultures of the isolate were prepared and the cellular structure of fungi was observed microscopically: (D) Mycelium and dicthiochlamydospores (Bright field 20X). (E) Young dichtyochlamydospore (a), conidiophore (b) and septate hyphae (c) (Bright field 40X). (F) Mature dichtyochlamidospores (Bright field 40X). (G) Mature sporodochia (stereoscopically obtained).
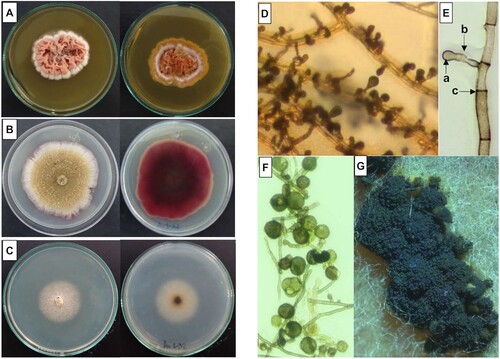
A microscopic analysis of fungi cells revealed that in PDA, septate and branched basal mycelia with brownish/red and short conidiophores were grown ((D,E)). Terminal dictiochlamydospores (a kind of asexual resistance spores) were present in conidiophores ((E,F)). The mature conidiophores grouped to form sporodochia were visible on the surface of the fungal colonies ((G)).
Taken together, all the characteristics, including the morphology of colonies and the reproduction structures microscopically observed in the microcultures, correlate with those previously described for Epicoccum nigrum, specifically with the colonial morphology described for Fávaro et al. (Citation23) for group 1 of E. nigrum.
3.2. Molecular identification of the AuNPs producer fungi
Accordingly, to the morphological characteristics, the molecular approach based on analysis of the Au1-8 ITSs rRNA sequence (GenBank, access number PP215817), revealed that the Au1-8 strain belongs to the Epicoccum genus (Ascomycota phylum, Dothideomycetes class, Pleosporales order). This classification is supported by results of the sequence alignment obtained using the nucleotide collection of the Genebank database of the NCBI, which showed a 100% identity and 99% coverage with sequences of the internal transcribed spacer 1, 5.8S ribosomal RNA gene, and internal transcribed spacer 2 of Epicoccum sp. Whereas 99.8% of identity and 98% of coverage with rDNA sequences of Epicoccum nigrum and 99.8% of identity and 98% of coverage with sequences of rDNA of Epicoccum layuense and Epicoccum dendrobii were encountered. However, the morphology was consistent with the phenotypic characteristics of Epicoccum nigrum.
3.3. Au-tolerance of the Au1-8 isolate, biosynthesis, stability and recycling of AuNPs
The tolerance of the fungal strain Au1-8 to the Au ions was established in 6 mM of AuCl3 (). The biosynthesis of AuNPs using the free-fungal PDB-filtrate ((B)) obtained from a fungal culture of 72 h in PDB ((A)), was successfully achieved by the addition of 1 mM of AuCl3, and verified by a color change from a translucent yellow pale color ((B)) to dark purple after 24 h of incubation with the metal solution ((C)). As can be observed in (D), the incubation of sterile PDB media with 1 mM of AuCl3 used as a negative control of AuNPs synthesis in media culture, also produces a color change from translucent yellow-pale to a red-brownish color after 24 h of incubation as indicative of AuNPs synthesis. The color change observed in (C) (fungal synthesis) and 3D (PDB synthesis), correlates with a maximum absorption peak in the UV-Visible spectra observed at 550 nm in (A) (blue line) and (A) (green line), respectively, which is a property of the AuNPs suspensions that is produced by the surface plasmon resonance (SPR) of the AuNPs. The color change and the absorption peak at 550 nm were not observed in the negative control of free-fungal PDB-filtrate incubated without the addition of metallic salt ((B) and (A), red line).
Figure 2. Gold tolerance of the Au1-8 Epicoccum nigrum strain. MIC (Minimal Inhibitory Concentration) of AuCl3 was determined in fungal cultures of PDA agar supplemented with increasing concentrations of AuCl3.
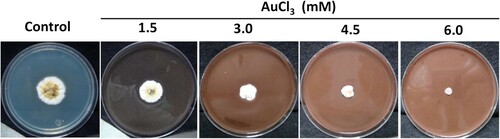
Figure 3. Extracellular synthesis of gold nanoparticles (AuNPs) by the Au1-8 Epicoccum nigrum strain. AuNPs were synthesized using the free-cell PDB-filtrate of fungal culture. (A) Fungal biomass incubated for 72 h, 28°C and 180 rpm in PDB media. (B) Free-cell PDB-filtrate without AuCl3. (C) Free-cell PDB-filtrate with 1.0 mM of AuCl3 incubated at 24 h, 28°C and 180 rpm. (D) PDB media incubated with 1.0 mM of AuCl3 for 24 h.
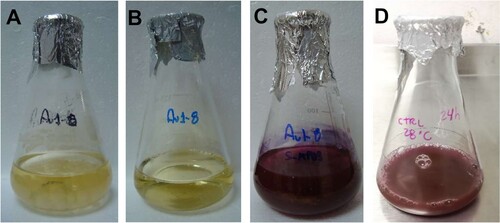
Figure 4. UV-Vis spectroscopy of AuNPs extracellularly synthesized by the Au1-8 Epicoccum nigrum strain. (A) Absorption spectra of AuNPs formed in the free-cell PDB-filtrate of Epicoccum nigrum Au1-8 (blue line). The absorbance of free-cell fungal filtrate without the addition of AuCl3 (red line) and PDB media culture with the addition of 1 mM of AuCl3 (green line) were analyzed as controls. (B) Absorption spectra of water-washed AuNPs suspensions obtained from the fungal synthesis (blue line) and PDB synthesis (green line). The UV-Vis spectra of a 12-months AuNPs suspension (brown line), a wet-sterilized AuNPs suspension (yellow line) and a PDB-synthesized AuNPs suspension (red line) were also obtained.
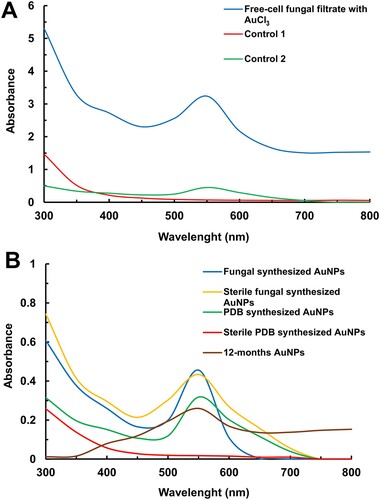
The AuNPs in the suspensions obtained by both the fungal-biosynthesis and PDB-synthesis were washed for purification. The UV-Vis spectra of purified AuNPs suspensions ((B), blue line) revealed a sharpened absorption peak compared with no purified AuNPs shown in (A) (blue line), the maximum absorption peak was observed at 550 nm for fungal biosynthesis, and a wider absorption peak (between 500 and 700 nm) with a maximum absorption peak at 550 nm for the PDB synthesis ((B), green line).
The stability of the AuNPs was assessed by wet sterilization of the AuNPs purified suspensions. The UV-Vis analysis of the fungal-biosynthesized AuNPs revealed that sterilization produce a broadening of the absorption peak (between 450 nm and 700 nm) with a maximum absorption at 550 nm ((B), yellow line), whereas the absence of absorption peak was evident in the PDB-synthesized AuNPs after its sterilization ((B), red line). Additionally, the UV-Vis spectra of a 12-months AuNPs suspension revealed a broadening and reduction of the maximal absorption peak at 550 nm, which suggests AuNPs aggregation over time ((B) brown line).
The concentration of gold associated with the AuNPs in the suspension obtained by fungal biosynthesis was calculated at about 194 mg L−1 using the equation CAuNPs = CAu ions - CAuSN (C = 196.97 mg L−1 – 2.93 mg L−1) as described in materials and methods. Where, CAu ions is the total concentration of gold ions used for the biosynthesis, in this case, 196.97 mg L−1 corresponding to 1 mM, and CAuSN is the concentration determined by EDXRF of the non-reduced gold ions that remain in the supernatant after centrifugation of the AuNPs suspension. This concentration indicates that about 98.5% of the gold ions added to the fungal filtrate were reduced to AuNPs during the biosynthesis process.
Regarding the gold recovery for recycling, an HAuCl4 solution with a concentration of 80.5 mg L−1 was easily obtained from a 12-months AuNPs suspension used as nanowaste (Figure S1). This result indicates that nanowaste AuNPs-suspensions generated by the biosynthesis method described in this work could be recycled using a simple method.
3.4. Characterization of the AuNPs synthesized by fungi
The AuNPs suspension was analyzed by STEM and HRTEM, and the results corroborate the presence of quasi-spherical AuNPs in a size range from 2 to 50 nm ((A,B,D-F), and Figure S2) and a mean particle size of 18 nm ± 3 nm (Figure S2). The AuNPs were absent in the fungal water-filtrate without metal used as a control, on which an amorphous material was observed ((C)). The elemental chemical analysis by EDX and the Fast Fourier Transform (FFT) analysis ((B,D), respectively) of areas including individual nanoparticles observed by HRTEM ((A,C)), revealed the crystal structure and metallic Au0 composition of the AuNPs. As shown in (B), the FTT patterns indicate that the d-spacing and Miller indices (0.232 nm (1 1 1), 0.221 nm (
1), 0.208 nm (0 0 2)) correspond to the crystallographic planes of the face-centered cube (fcc) crystal structure of metallic gold (Au0) when is viewed along to the [110] zone axis. Moreover, the EDX analysis detected strong signals of gold (Au) ((D)) that were absent in the amorphous material observed in the control ((E,F)). Additionally, moderate signals of elements such as oxygen (O), silicon (Si), sodium (Na), chlorine (Cl), potassium (K), sulfur (S), carbon (C) and copper (Cu), were detected in the areas of both the AuNPs and control suspensions analyzed, the two latest due to the carbon-coated copper grid used for this analysis ((D,G)). The elemental composition of the AuNPs was corroborated by an elemental chemical mapping of an AuNP cluster, which revealed the single presence of Au signal in the merged AuNPs area, whereas other elements as silicon (Si) and oxygen (O) were detected in the overall area analyzed ((G)).
Figure 5. Electron microscopy analysis of fungal synthesized AuNPs. Annular dark field ADF-STEM images (upper panel, A–C) and HRTEM images (lower panel, D–F). Purified water-suspensions of AuNPs formed by the Au1-8 strain of Epicoccum nigrum (A and B) and the fungal free-cell PDB-filtrate without the addition of metal used as control (C) were deposited over copper grids. Brighter dots with different sizes of metal AuNPs are displayed in the ADF-STEM images (A and B) and atomic resolution images of single nanoparticles are shown in the lower panel (D–F).
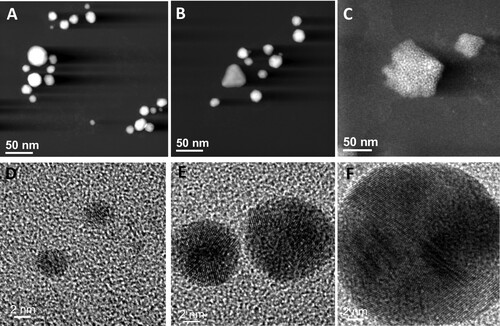
Figure 6. Crystalline structure and elemental chemical composition of AuNPs biosynthesized with free-cell PDB-filtrate of the Epicoccum nigrum Au1-8 strain. (A) and (C) HRTEM images that show individual AuNPs. (B) FFT pattern of the atomic resolution image in A. (D) Energy dispersive X-ray (EDX) spectrum obtained from the AuNP selected area in C. (E) ADF-STEM image that shows the amorphous material in the negative control sample and the area selected for EDX analysis. (F) EDX spectrum of the negative control selected area. (G) Elemental chemical mapping of an AuNP cluster.
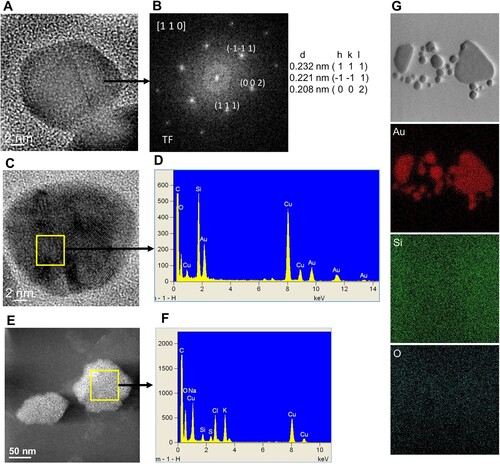
In the XRD pattern obtained from sample analysis of the AuNPs powder (, blue line), several peaks were observed at 2θ = 38.20°, 44.53°, 64.73°, 77.74°, which correspond to (111), (200), (220) and (311) Bragg’s reflections of the fcc phase of gold (Au0) according with the database Rigaku PDXL software version 2.4.2.0 (PDF card, No. 03-0658601). In the XRD pattern obtained from the analysis of the control sample (fungal PDB-filtrate), no evident diffraction peaks were observed (, red line).
Figure 7. X-ray diffraction pattern of the fungal biosynthesized AuNPs powder. Suspension of the fungal biosynthesized AuNPs was freeze-dried lyophilized and ground to prepare the samples for XRD analysis. The X-ray diffraction pattern obtained from the AuNPs powder (blue line) and the fungal PDB-filtrate used as a negative control (red line) are shown.
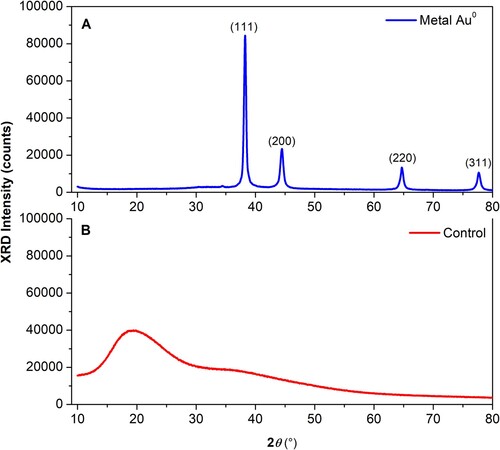
Using the highest intensity diffraction peak centered at 2θ = 38.25173°, and the full-width at half-maximum (FWHM) β with the value of 0.38493° for metallic gold patterns, the average of the crystallite diameter D was obtained by the Scherrer formula D = (0.9)λ / (β cosθβ), where λ is the wavelength (1.5406 Å), θβ is the Bragg angle. The average D values obtained were approximately 21.84 nm for the AuNPs. These D values are in good concordance with the AuNPs dimensions observed by TEM ( and Figure S1).
To investigate whether fungal-derived molecules are present during the biosynthesis process of AuNPs, an analysis by FTIR spectroscopy of both the fungal-synthesized AuNPs and the fungal PDB-filtrate without AuCl3 used as control, was performed. The results indicate the presence of bands at wavenumbers corresponding to three functional groups (). A broad band with a wavenumber around 3330 cm−1 was assigned to the stretching vibration of the O-H group. The subtle bands with wavenumbers at 2120, 2112 and 2110 cm−1 correspond to the stretching vibration of a C–H bond, and the stretching band with wavenumber at 1641 cm−1 was assigned to the asymmetric stretching vibration of the C = O group (Citation28, Citation29). Subtle displacements were detected in bands corresponding to the stretching vibration of the O-H (3330 to 3326 cm−1) and the C–H bonds (2120–2112 to 2110 cm−1) in the AuNPs samples (, blue line) compared to the control (, red line).
3.5. Effect of biosynthesized AuNPs over endospore germination, morphology and surface interactions
The germination of Bacillus subtilis endospores exposed to AuNPs was evaluated to determine the potential of AuNPs against the endospore germination. The germination percentages of the endospores were decreased after the exposition to 100 to 300 mg/L of AuNPs during different times (). These results indicate that in all the AuNPs concentrations tested the percentage of endospore germination was reduced in a dose-dependent manner. A concentration of 100 mg/L of AuNPs was sufficient to reduce 40% of the endospore germination after an exposition time of 6 h, whereas a concentration of 300 mg/L of AuNPs was necessary to decrease the spore germination between 80 to 90% after exposition times of 6 to 48 h, respectively.
Figure 9. Sporicidal effect of fungal-biosynthesized AuNPs over Bacillus subtilis endospores. The inhibition of the endospore germination at different times of treatment with increasing concentrations (100, 200 and 300 mg/L) of AuNPs was used as a measure of the sporicidal activity of AuNPs. The percentage of germination of AuNPs-treated endospores was calculated concerning germinated untreated endospores (established as control). Asterisk (*) indicates significant differences determined by ANOVA (p < 0.05).
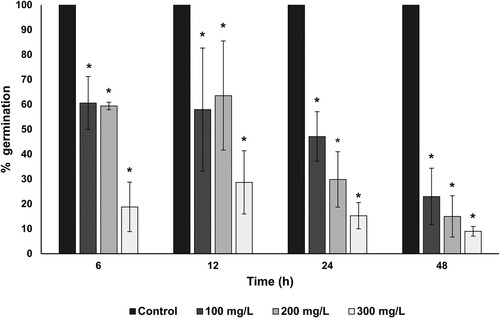
The morphology of the endospores after their exposition to the AuNPs was analyzed by SEM. In the endospore suspension exposed to the AuNPs, wrinkled shapes and disrupted endospores were observed ((A)), whereas whole endospores were observed in the untreated endospores ((B)). Accordingly, to these results, differences were observed in the FTIR spectra obtained with exposed and unexposed endospores to AuNPs (). The FTIR spectrum of the unexposed B. subtilis endospores (control) showed two major bands with wavenumbers at 1644 and 1540 cm−1, assigned to the signals from amide I and amide II groups of proteins, respectively, and a subtle band at 1402 cm−1 corresponding to carboxylic group of proteins ((B)) (Citation29–31). By contrast, in the FTIR spectrum of the AuNPs-exposed endospores the band corresponding to amide I group was absent and the corresponding to amide II is slightly shifted to 1544 cm−1 and with lower intensity in comparison with the rest of the bands present in the spectra, whereas the bands at 1742 and 1713cm−1 assigned to vibrational signals of the functional groups carbonyl and ketone present in ester groups of lipids and nucleic acids, were also detected (Citation31). A band with a wavenumber at 1172 cm−1 was assigned to the S = O stretching sulfone group (Citation28, Citation31). Along with this band associated with the sulfone, another band appears in the spectrum centered at 1060 cm−1, this band corresponds to vibrational modes associated with ring vibrations and the coupling between the S−O stretching vibration of the (SO3)2− groups (Citation32) and the R-SOH sulfenic group (Citation33). A band with a wavenumber at 1458 cm−1 was assigned to the pyridine ring bending of dipicolinic acid (DPA) reported at wavenumbers between 1427 and 1458 cm−1 ((A)) (Citation29, Citation34).
Figure 10. Effect of fungal-biosynthesized AuNPs over the structure of Bacillus subtilis endospores. (A) SEM micrograph of Bacillus subtilis endospores exposed to 100 mg/L for 6 h to fungal-synthesized AuNPs. Disrupted (red arrows) and shrinked (yellow arrows) spores are pointed out. (B) SEM micrograph of untreated Bacillus subtilis endospores (control).
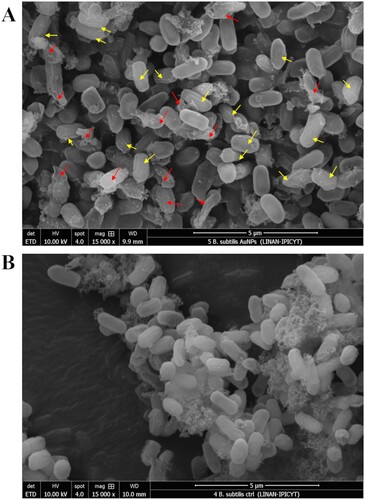
Figure 11. FTIR Spectroscopy of Bacillus subtilis endospores exposed to fungal-biosynthesized AuNPs. (A) IR spectrum of B. subtilis endospores exposed to 100 mg/L for 6 h to fungal-synthesized AuNPs. Functional group assignment is indicated in the graphs. (B) FTIR spectrum of B. subtilis endospores unexposed to AuNPs (control).
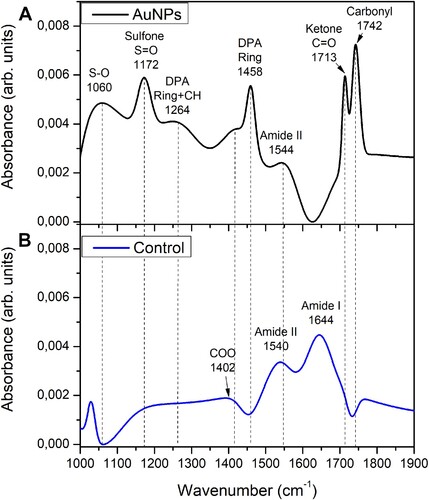
Furthermore, to corroborate that the signal, identified for amide I, does not correspond to the vibration of O-H bonds of water in this region, dry samples of Bacillus subtilis endospores exposed and not exposed to fungal-biosynthesized AuNPs prepared over aluminum substrates were characterized by FTIR spectroscopy, under the same conditions and parameters exposed in Section 2.9. The obtained vibrational bands shown in Figure S3, correspond to those previously identified for the FTIR spectra of Bacillus subtilis endospores exposed and not exposed to fungal-biosynthesized AuNPs in . With this result, it is possible to determine that the signal, identified for amide I, does not correspond to the vibration of OH bonds and corresponds to amide I, as it is labeled in .
4. Discussion
The antimicrobial properties of metal-based NPs have been widely studied (Citation7). Specifically, silver and gold NPs have been extensively considered as a good alternative to be used as antimicrobial agents for the control of both planktonic and biofilm-former pathogenic bacteria (Citation35–42). Particularly, the study of the antibacterial activity of AuNPs has gained attention since this material is less toxic to mammalian cells compared with AgNPs, which are commonly used as antimicrobial agents (Citation43). The antimicrobial activity of AuNPs seems to be dependent on their structure, composition, size, shape and dose, which can greatly vary in function of the methods used for their synthesis, as well as the microbial cell characteristics (Citation44). The antimicrobial use of AuNPs could be limited by the high costs for the production of purified AuNPs suspensions in comparison with other metal-based NPs, however, the biological methods of synthesis known as biosynthesis, have been explored as eco-friendly alternatives for the AuNPs production at lower costs concerning other methods (Citation4, Citation10, Citation45). The fungal-biosynthesis has attracted attention due to the feasibility of using extracellular fungal molecules secreted as intermediaries for nucleation, growth and stabilization of the AuNPs (Citation12, Citation46, Citation47). In this work, AuNPs were obtained by a simple, cost-effective and ‘green’ method using the Au-tolerant isolate identified as Epicoccum nigrum (Au1-8). The fungal biosynthesis was carried out using the molecules secreted by E. nigrum Au1-8 into the media culture, which contributes to gold ions reduction, growth and stabilization of the AuNPs. This suggestion is supported by the detection of FTIR vibrational signals corresponding to biomolecule-functional groups in the AuNPs suspensions, its crystalline Au0 nature determined by XRD and the stability that they showed over time and even after the sterilization process ((B)), which produced a widening of the absorption peak in the UV-Vis spectrum as indicative of AuNPs cluster formation (Citation48). Interestingly, the AuNPs synthesis was also achieved in the PDB media used as negative control, indicating that media culture PDB components can also promote the nucleation and synthesis of AuNPs, however, the wide absorption peak observed in the UV-Vis spectrum indicate a wide size distribution of AuNPs (Citation48). Moreover, the disappearance of the absorption peak after the sterilization treatment of PDB-synthesized AuNPs indicates its low stability. Together these results suggest that fungal-derived molecules are necessary to obtain stable AuNPs suspension with narrow-size distribution as previously has been reported (Citation9, Citation11).
Although the sporicidal activity of some metal NPs against bacterial endospores has been evaluated, the reports are still scarce. For example, the activity of biogenic AgNPs against B. anthracis endospores was demonstrated, whereas the gold-based NPs (Au/CuS) did not show sporicidal activity (Citation20, Citation21). The results obtained in this work revealed that AuNPs biosynthesized using E. nigrum Au1-8 showed sporicidal activity and were effective in producing a viability reduction of B. subtilis 168 endospores. A maximal reduction of 85 to 90% of the endospore viability was achieved after its exposition to 300 mg/L of AuNPs for 24 to 48 h, respectively. Based on the antimicrobial mechanisms of action proposed for the AuNPs, two mechanisms are consistent with the results obtained in this work, these include the interaction with the thiol groups of proteins and the mechanical rupture of bacterial membrane (Citation18, Citation19). The results obtained by SEM support that a mechanical rupture was caused by the AuNPs to the endospore, which consists of highly resistant cells with a well-structured surface of protein layers called spore coats (Citation49). This result is supported by the FTIR analysis on which two characteristic bands of DPA were detected in endospores exposed to AuNPs indicating the liberation of DPA from the endospore core.
The spore rupture caused by AuNPs could be produced by a disruption of the spore coats with the consequent loss of the internal content (wrinkled spores/yellow arrows, ), which eventually produces the rupture of the endospore (broken spores/red arrows, ). This notion is consistent with the absence of the amide I band and the shift and low intensity of the amide II band of proteins in FTIR spectrum of endospores exposed to the AuNPs, which indicates an interaction with the coat proteins and suggests a modification of the protein structure. This is supported by previous reports on which vibrational frequency of the amide bands shifts and changes as indicative of secondary structure conversions of proteins to intermolecular β-sheet, due to the protein denaturing and subsequent aggregation (Citation31). Interestingly, the presence of sulfone and sulfenic groups in samples of endospores exposed to AuNPs, strongly suggests that AuNPs produce the oxidation of thiol groups of the coat spore proteins, which are reported to be rich in tyrosine and cysteine (Citation49).
To our knowledge, this is the first report of the evaluation of the sporicidal activity of fungal-biosynthesized AuNPs against bacterial endospores. This few explored field opens the opportunity to know the mechanisms of action involved in AuNPs sporicidal activity, to find new alternatives of sporicidal agents to deal with the highly resistant and prevalent bacterial endospores, especially to those of pathogenic species (i.e. B. anthracis, B. cereus, C. tetani, C. botulinum, C. perfringens) which have the ability to germinate and produce infections and/or poisoning/intoxications (Citation22).
Supplemental Material
Download MS Word (723.3 KB)Acknowledgements
We gratefully acknowledge to Ph.D. Mario Pedraza Reyes (Biology Department of University of Guanajuato, México) for the kindly donation of the B. subtilis 168 strain. We also thank the National Laboratory for Research in Nanosciences and Nanotechnology, LINAN-IPICYT for the Microscopy facilities.
Disclosure statement
No potential conflict of interest was reported by the author(s).
Data availability statement
All data of gene sequence will be available at the NCBI Genebank database (Accession number PP215817).
Additional information
Funding
References
- Li, N.; Zhao, P.; Astruc, D. Angew. Chem. Int. Ed. 2014, 53 (7), 1756–1789. doi:10.1002/anie.201300441.
- Sangwan, S.; Seth, R. J. Clust. Sci. 2021, 33 (2), 749–764. doi:10.1007/s10876-020-01956-8.
- Arvizo, R.R.; Bhattacharyya, S.; Kudgus, R.A.; Giri, K.; Bhattacharya, R.; Mukherjee, P. Chem. Soc. Rev. 2012, 41 (7), 2943–2970. doi:10.1039/c2cs15355f.
- Castro, L.; Blázquez, M.L.; González, F.G.; Ballester, A. Rev. Adv. Sci. Eng. 2014, 3 (3), 199–216. doi:10.1166/rase.2014.1064.
- Bansal, S.A.; Kumar, V.; Karimi, J.; Singh, A.P.; Kumar, S. Nanoscale Adv. 2020, 2 (9), 3764–3787. doi:10.1039/D0NA00472C.
- Hu, X.; Zhang, Y.; Ding, T.; Liu, J.; Zhao, H. Front. Bioeng. Biotechnol. 2020, 8, 990. doi:10.3389/fbioe.2020.00990.
- Sánchez-López, E.; Gomes, D.; Esteruelas, G.; Bonilla, L.; Lopez-Machado, A.L.; Galindo, R.; Souto, E.B. Nanomaterials 2020, 10 (2), 292. doi:10.3390/nano10020292.
- Amina, S.J.; Guo, B. Int. J. Nanomed. 2020, 15, 9823–9857. doi:10.2147/IJN.S279094.
- Mikhailova, E.O. J. Funct. Biomat. 2021, 12 (4), 70. doi:10.3390/jfb12040070.
- Santhosh, P.B.; Genova, J.; Chamati, H. Chemistry 2022, 4 (2), 345–369. doi:10.3390/chemistry4020026.
- Rao, Y.; Inwati, G.K.; Singh, M. Future Sci. OA 2017, 3 (4), FSO239. doi:10.4155/fsoa-2017-0062.
- Gu, X.; Xu, Z.; Gu, L.; Xu, H.; Han, F.; Chen, B.; Pan, X. Environ. Chem. Lett. 2021, 19, 167–187. doi:10.1007/s10311-020-01071-0.
- Okkeh, M.; Bloise, N.; Restivo, E.; De Vita, L.; Pallavicini, P.; Visai, L. Nanomaterials 2021, 11 (2), 312. doi:10.3390/nano11020312.
- Pissuwan, D.; Cortie, C.H.; Valenzuela, S.M.; Cortie, M.B. Trends Biotechnol. 2010, 28 (4), 207–213. doi:10.1016/j.tibtech.2009.12.004.
- Zhang, Y.; Shareena Dasari, T.P.; Deng, H.; Yu, H. J. Environ. Sci. Health C 2015, 33 (3), 286–327. doi:10.1080/10590501.2015.1055161.
- Yu, Q.; Li, J.; Zhang, Y.; Wang, Y.; Liu, L.; Li, M. Sci. Rep. 2016, 6 (1), 26667. doi:10.1038/srep26667.
- Penders, J.; Stolzoff, M.; Hickey, D.J.; Andersson, M.; Webster, T.J. Int. J. Nanomed. 2017, 12, 2457–2468. doi:10.2147/IJN.S124442.
- Cui, Y.; Zhao, Y.; Tian, Y.; Zhang, W.; Lü, X.; Jiang, X. Biomaterials 2012, 33 (7), 2327–2333. doi:10.1016/j.biomaterials.2011.11.057.
- Linklater, D.P.; Baulin, V.A.; Le Guével, X.; Fleury, J.B.; Hanssen, E.; Nguyen, T.H.P.; Ivanova, E.P. Adv. Mater. 2020, 32 (52), 2005679. doi:10.1002/adma.202005679.
- Addae, E.; Dong, X.; McCoy, E.; Yang, C.; Chen, W.; Yang, L. J. Biol. Eng. 2014, 8 (1), 1–11. doi:10.1186/1754-1611-8-11.
- Tehri, N.; Kaur, R.; Maity, M.; Chauhan, A.; Hooda, V.; Vashishth, A.; Kumar, G. Prep. Biochem. Biotechnol. 2020, 50 (9), 865–873. doi:10.1080/10826068.2020.1762212.
- Tehri, N.; Kumar, N.; Raghu, H.V.; Vashishth, A. Ann. Microbiol. 2018, 68, 513–523. doi:10.1007/s13213-018-1361-z.
- Fávaro, L.C.D.L.; de Melo, F.L.; Aguilar-Vildoso, C.I.; Araujo, W.L. PLoS One 2011, 6 (8), e14828. doi:10.1371/journal.pone.0014828.
- White, T. J.; Bruns, T.; Lee, S. J. W. T.; Taylor, J. In PCR Protocols: A Guide to Methods and Applications; Innis, M.A., Gelfand, D.H., Sninsky, J.J., White, T.J., Eds.; Academic: New York, 1990; pp 315–322.
- Guilger-Casagrande, M.; Lima, R.D. Front. Bioeng. Biotechnol. 2019, 7, 287. doi:10.3389/fbioe.2019.00287.
- Oestreicher, V.; García, C.S.; Soler-Illia, G.J.; Angelomé, P.C. ChemSusChem. 2019, 12 (21), 4882–4888. doi:10.1002/cssc.201901488.
- Nicholson, W.L.; Setlow, P.; Harwood, C.R.; Cutting, S.M. Molecular Biological Methods for Bacillus; John Willey & Sons: New York, 1990; 391–451.
- Socrates, G. Infrared and Raman Characteristic Group Frequencies: Tables and Charts; John Wiley & Sons: New York, 2004.
- Bombalska, A.; Mularczyk-Oliwa, M.; Kwaśny, M.; Włodarski, M.; Kaliszewski, M.; Kopczyński, K.; Trafny, E.A. Spectrochim. Acta A Mol. Biomol. Spectrosc. 2011, 78 (4), 1221–1226. doi:10.1016/j.saa.2010.10.025.
- Perkins, D.L.; Lovell, C.R.; Bronk, B.V.; Setlow, B.; Setlow, P.; Myrick, M.L. Appl. Spectrosc. 2004, 58 (6), 749–753. doi:10.1366/000370204873079.
- Alvarez-Ordóñez, A.; Mouwen, D.J.M.; López, M.; Prieto, M. J. Microbiol. Methods 2011, 84 (3), 369–378. doi:10.1016/j.mimet.2011.01.009.
- Gao, K.; Jiang, M.; Guo, C.; Zeng, Y.; Fan, C.; Zhang, J.; Dang, Z. Sci. Total Environ. 2019, 690, 1100–1109. doi:10.1016/j.scitotenv.2019.06.483.
- Parker, F.S. Applications of Infrared Spectroscopy in Biochemistry, Biology and Medicine; Plenum Press: New York, 1971; 104–226.
- Goodacre, R.; Shann, B.; Gilbert, R.J.; Timmins, E.M.; McGovern, A.C.; Alsberg, B.K.; Logan, N.A. Anal. Chem. 2000, 72 (1), 119–127. doi:10.1021/ac990661i.
- Singh, P.; Pandit, S.; Beshay, M.; Mokkapati, V.R.S.S.; Garnaes, J.; Olsson, M.E.; Mijakovic, I. Artif. Cells Nanomed. Biotechnol. 2018, 46 (sup3), 886–899. doi:10.1080/21691401.2018.1518909.
- Gondil, V.S.; Kalaiyarasan, T.; Bharti, V.K.; Chhibber, S. Biotechnology 2019, 9, 1–13.
- Dong, Y.; Zhu, H.; Shen, Y.; Zhang, W.; Zhang, L. PLoS One 2019, 14 (9), e0222322. doi:10.1371/journal.pone.0222322.
- Oves, M.; Rauf, M.A.; Hussain, A.; Qari, H.A.; Khan, A.A.P.; Muhammad, P.; Ismail, I.I. Front. Pharmacol. 2019, 10, 801. doi:10.3389/fphar.2019.00801.
- Hossain, M.M.; Polash, S.A.; Takikawa, M.; Shubhra, R.D.; Saha, T.; Islam, Z.; Sarker, S.R. Front. Bioeng. Biotechnol. 2019, 7, 239. doi:10.3389/fbioe.2019.00239.
- Figueiredo, E.P.; Ribeiro, J.M.; Nishio, E.K.; Scandorieiro, S.; Costa, A.F.; Cardozo, V.F.; Nakazato, G. Int. J. Nanomed. 2019, 14, 7975–7985. doi:10.2147/IJN.S211756.
- Tanase, C.; Berta, L.; Coman, N.A.; Roșca, I.; Man, A.; Toma, F.; Mare, A. Nanomaterials 2019, 9 (11), 1541. doi:10.3390/nano9111541.
- Rodríguez-Serrano, C.; Guzmán-Moreno, J.; Ángeles-Chávez, C.; Rodríguez-González, V.; Ortega-Sigala, J.J.; Ramírez-Santoyo, R.M.; Vidales-Rodríguez, L.E. PLoS One 2020, 15 (3), e0230275. doi:10.1371/journal.pone.0230275.
- Verma, S.K.; Jha, E.; Panda, P.K.; Thirumurugan, A.; Suar, M. Adv. Nanostruct. Mater. Environ. Remed. 2019, 80, 145–171. doi:10.1007/978-3-030-04477-0_6.
- Tiwari, P.M.; Vig, K.; Dennis, V.A.; Singh, S.R. Nanomaterials 2011, 1 (1), 31–63. doi:10.3390/nano1010031.
- Lee, K.X.; Shameli, K.; Yew, Y.P.; Teow, S.Y.; Jahangirian, H.; Rafiee-Moghaddam, R.; Webster, T.J. Int. J. Nanomed. 2020, 15, 275–300. doi:10.2147/IJN.S233789.
- Siddiqi, K.S.; Husen, A. Nanoscale Res. Lett. 2016, 11, 1–15. doi:10.1186/s11671-015-1209-4.
- Kalimuthu, K.; Cha, B.S.; Kim, S.; Park, K.S. Microchem. J. 2020, 152, 104296. doi:10.1016/j.microc.2019.104296.
- Shokri, E.; Hosseini, M.; Davari, M.D.; Ganjali, M.R.; Peppelenbosch, M.P.; Rezaee, F. Sci. Rep. 2017, 7 (1), 458. doi:10.1038/srep45837.
- Driks, A. Microbiol. Mol. Biol. Rev. 1999, 63 (1), 1–20. doi:10.1128/MMBR.63.1.1-20.1999.