Abstract
Tibetan chicken (TC) is a unique chicken breed that has inhabited the Tibetan Plateau for thousands of years and has adapted to the harsh environment of an altitude of over greater than 4000 m. The changes in blood characteristics that occur in high-altitude rearing of TCs are unknown. To obtain a deeper insight into the blood characteristics of chickens in low and high altitudes, we focussed on four chicken populations to compare the variations among them. In the present study, we found significant differences in white blood cell (WBC) count, red blood cell (RBC) count and haematocrit (HCT) values between TCs and other chicken populations. When TCs were reared at low altitude, there was a significant (p < .05) decrease in the WBC, RBC and HCT values compared with the TCs reared at high altitude; however, whether the mean corpuscular volume (MCV) value is related to oxygen transport and hypoxia adaptation requires further study. It is likely that haemoglobin (HGB), mean corpuscular haemoglobin (MCH) and MCH concentration (MCHC) values are decrease at high-altitude hypoxic environments. Therefore, the increase in WBC, RBC and HCT values may be the common haematological mechanism for TCs to adapt to high-altitude hypoxia. These research results provide the scientific basis and theory to explain how TCs are able to adapt to high-altitude hypoxia.
Introduction
Birds arose from their dinosaur ancestors and took to the skies over 225 million years ago. Since that time, birds have become abundant at high elevations. High elevation indicates low atmospheric pressure, hypoxia and alpine conditions, which are likely to have acted as agents of natural selection to ultimately lead to specific adaptations. A constant supply of sufficient oxygen is a prerequisite to sustain normal metabolism and survival for most aerobic organisms in their natural environment (Liu et al. Citation2013). Hypoxia plays a significant role in the pathogenesis of major causes of mortality, including cancer, cerebral and myocardial ischaemia, and chronic heart and lung diseases (Semenza Citation2000). High-altitude environments are particularly challenging for animals due to their harsh extremes. The hypoxia at high altitudes can be particularly debilitating for lower-altitude species, such that an organism’s normal metabolism is a challenge to sustain. However, high-altitude mammals and birds have developed genetic adaptations to allow their survival in extremely hypoxic environments (Chappell and Snyder Citation1984; Beall Citation2007; Storz and Moriyama Citation2008; Lorenzo et al. Citation2014). Therefore, it is of vital importance to investigate hypoxia adaptation.
Many physiological traits in the O2 transport pathway (such as enhanced gas-exchange efficiency in the lungs, augmented O2 diffusion capacity in peripheral tissues and a high aerobic capacity, maintenance of O2 delivery and oxygenation in the brain during hypoxia) distinguish birds from other vertebrates, and interestingly, these traits are not high-altitude adaptations because they are also characteristics of lowland birds (Scott Citation2011). The mechanisms for mammal high-altitude adaptation have been widely explored in humans (Huerta-Sánchez et al. Citation2014), pigs (Li et al. Citation2013), dogs (Gou et al. Citation2014) and horses (Hendrickson Citation2013). Many previous studies found that high-altitude adaptations have been well documented in birds in the past. Scott et al. (Citation2011) reported that adaptations in mitochondrial enzyme kinetics and O2 transport capacity may, therefore, contribute to the exceptional ability of Bar-headed geese (Anser indicus) to fly at high altitudes. In addition, a recent study showed that Bar-headed geese can reach high altitudes during their migration across the Himalayas and Tibetan Plateau because they depend on the unique cardiorespiratory physiology of birds along with several evolved specialisations across the O2 transport cascade (Scott et al. Citation2015). Certainly, the ability of Bar-headed geese to conserve energy during Himalayan migrations is very important (Bishop et al. Citation2015). The distinctive features of birds that fly at high altitudes include an enhanced hypoxic ventilatory response, an effective breathing pattern, larger lungs, haemoglobin (HGB) with a higher O2 affinity, further augmentation of O2 diffusion capacity in the periphery and multiple alterations in the metabolic properties of cardiac and skeletal muscle (Scott Citation2011). However, the scientific basis of high-altitude adaptation is unclear in chickens. Chickens (Gallus gallus domesticus) are important avian model organisms that bridge the evolutionary gap between mammals and other vertebrates; thus, it is necessary to explore the blood characteristics of chickens in low and high altitudes to better understand chicken adaptations to high altitude.
The Tibetan chicken (TC, Gallus gallus) is a unique chicken breed that has inhabited the Tibetan Plateau for thousands of years and has adapted to the harsh environment of altitudes greater than 4000 m, especially chronic hypoxia and low pressure (Sun et al. Citation2013). TCs have lived in a relatively isolated environment after entering into high altitudes and thus developed unique genetic characters and several productive parameters, as follows. The TC feather colour can generally be divided into golden, red, black and blue colouration, and the skin colour is white. At 180 d of age, the male and female TC weight is 1225.22 g and 1085.52 g, respectively. At 300 d of age, the egg number and the egg weight of TCs is 42.75 and 34.97 g, respectively. The whole net carcase rate of male chickens (73.18%) is 1.11% greater than female chickens (72.17%). The first egg appears at 232.06 d of age, and the body weight is 1120.45 g at that time. During adulthood, the male and the female TC weight is 1490 g and 1150 g, respectively. The male and the female TC body slanting length is 21.2 cm and 19.2 cm, respectively. The male and the female TC pelvis width is 7.6 cm and 7.3 cm, respectively. In addition, the male and the female TC Shank length is 9.5 cm and 8.5 cm, respectively (data source: Breeds of Domestic Poultry in China). A previous study demonstrated that hypoxia increased embryo mortality (Villamor et al. Citation2004). TCs have 80% hatchability in hypoxia conditions (oxygen partial pressure: 12.78 kPa) (Zhang et al. Citation2004), while lowland chicken breeds only have 30% hatchability in the same hypoxia conditions (oxygen partial pressure: 12.78 kPa) (Hao et al. Citation2006). Therefore, TC is a good representative breed to investigate high-altitude adaptations.
TCs living in the low-pressure hypoxia environment of the plateau are part of a complex process. Previous studies have shown that there is a significant difference in chicken blood characteristics at low and high altitudes (Carey and Martin Citation1997; Gou et al. Citation2005; Zhang et al. Citation2007). TCs inhabiting high-attitudes have an increased number of RBC, oxygen affinity in blood and HGB concentrations and a decreased MCV to overcome an environment of harsh extremes (Zhang et al. Citation2007). In addition, Bar-headed geese, a migratory species that inhabits altitudes of 9200 m, had a high oxygen affinity and invariable values of RBC, HCT, HGB, and MCV at sea-level and high-altitude conditions (Black and Tenney Citation1980). Evidence from a number of studies suggests that modifications of HGB function may often play a key role in mediating an adaptive response to high-altitude hypoxia (Storz and Moriyama Citation2008). Certainly, the exposure of lowland biology to high-altitude hypoxia leads to an increased number of erythrocytes. To investigate the blood characteristics (WBC, RBC, HGB, MCH MCHC, MCV, HCT and RDWc) in low-and high-altitude chickens, we focussed on four chicken populations (TC, LTC, LC, LTC) to compare the variations among them.
Materials and methods
Experimental animals and management
TCs were procured from local conservation farms in Xiangcheng, Batang Counties in Ganzi Tibetan Autonomous Prefecture, Sichuan Province, China. One hundred eggs were collected from the TC, an indigenous domesticated breed that is typically reared at high altitudes (approximately 3100 m). Each 50 TC eggs were incubated, and chicks were reared at Ganzi (3100 m) (TC) and Ya’an (600 m) (LCT), respectively. Each 50 lowland chicken eggs were also incubated and chicks were reared at Ya’an (600 m) (LC) and Baoxing (2500 m) (HLC), respectively (the sampling details are listed in Table ). We randomly selected 20 chickens from the four populations (TC, LTC, LC, HLC). All 80 birds were housed in the room until 24 weeks of age. The management and feeding regimens were the same for all of the birds.
Table 1. Sample information used in present study.
Blood collection and analyses
On the final day of the experiments, six chickens per treatment with medium body weight were selected from each group, and a 2-mL sample of blood was collected in plastic tubes with anticoagulants via the wing vein of each bird. After collecting blood samples, we gently mixed the blood samples. Finally, we used an XFA6000 (Nanchang, Beijing. China) automatic blood cell analyser to measure the parameters of the blood. Several blood indices including white blood cell (WBC) counts, red blood cell (RBC) counts, HGB, mean corpuscular haemoglobin (MCH), MCH concentration (MCHC), haematocrit (HCT), mean corpuscular volume (MCV) and variable coefficients of red cell distribution width (RDWc) in four populations were measured. No bird was injured or slaughtered unexpectedly in the process of sampling. The protocol was approved by the Committee on the Care and Use of Laboratory Animals of the State-level Animal Experimental Teaching Demonstration Center of Sichuan Agricultural University.
Statistical analysis
Statistical analysis was performed using one-way ANOVA and run with SAS v9.0 (SAS Institute Inc., Cary, NC) to test for differences among different groups. All data are expressed as the mean ± standard error (SE), and p values < .05 were considered statistically significant.
Results
Blood cell count in four chicken populations
In this study, the relative expression levels of WBC (A) and RBC (B) in four chicken populations are presented in Figure The effects of altitude on the WBC and RBC values were significant (p < .05; Figure ) in the present study. It is obvious that the mean WBC and RBC values for TC were significantly higher than those of other chickens (LTC and LC) reared at low altitudes. TC had significantly higher WBC and RBC values than HLC when they were both reared at high altitudes (p < .05), but there was no significant difference in the WBC and RBC values between LTC and LC when they were both reared at low altitudes (p > .05). The other two chicken populations (HLC and LC) showed no significant difference in the mean WBC and RBC values (p > .05).
Figure 1. Relative expression levels of white blood cell (WBC) count (A) and red blood cell (RBC) count (B) in four chicken populations. Data are shown as the mean ± SE from six independent experiments. The bars without the same letter indicate differences significant at p < .05. TC: Tibetan chicken; LTC: Tibetan chicken reared at low altitude; LC: Peng County yellow chicken; HLC: Baoxing native chicken reared at high altitude.
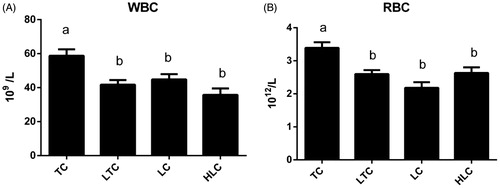
Effect of altitude on HGB, MCH and MCHC
In this study, the relative expression levels of HGB (A), MCH (B) and MCHC (C) in four chicken populations are shown in Figure . The effect of altitude on HGB was significant (p < .05; Figure ). There was a significant decrease in the HGB, MCH and MCHC values in the TC compared with those chickens (LTC, LC) reared at low altitudes (p < .05). The TC HGB, MCH and MCHC values increased significantly from high-altitude (TC) to low-altitude (LTC) (p < .05) conditions, while LTC had no significant difference when compared with LC (p > .05). The difference between the two populations (HLC and LC) was not significant when they were reared at low altitude (p > .05).
Figure 2. Relative expression levels of haemoglobin (HGB) (A), mean corpuscular haemoglobin (MCH) (B) and MCH concentration (MCHC) (C) in four chicken populations. Data are shown as the mean ± SE from six independent experiments. The bars without the same letter indicate differences significant at p < .05. TC: Tibetan chicken; LTC: Tibetan chicken reared at low altitude; LC: Peng County yellow chicken; HLC: Baoxing native chicken reared at high altitude.
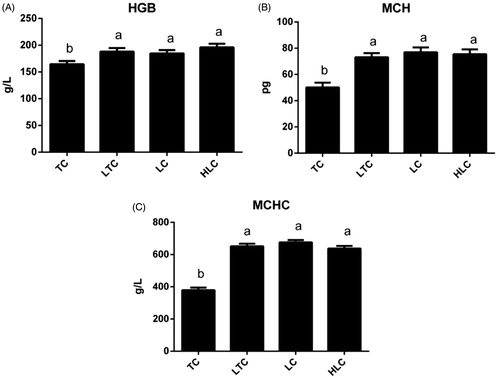
Other blood physiological parameters of the four chicken populations
A summary of the effects of altitude on MCV (A), HCT (B) and RDWc (C) is presented in Figure . TC had higher HCT and MCV values than chickens (LTC and LC) reared at low altitude (p < .05) but had similar changes as the HLC (p > .05). When both chickens were reared at low altitude, there was no significant difference in the HCT and MCV values between the two populations (HLC and LC) (p > .05). As shown in Figure , there was a significant increase in the RDWc value in the HLC compared with the other chickens (TC, LTC and LC) (p < .05). However, the RDWc values for the three chickens (TC, LTC and LC) were not significantly different (p > .05).
Figure 3. Relative expression levels of haematocrit (HCT) (A), mean cell volume (MCV) (B) and variable coefficient of red cell distribution width (RDWc) (C) in four chicken populations. Data are shown as the mean ± SE from six independent experiments. The bars without the same letter indicate differences significant at p < .05. TC: Tibetan chicken; LTC: Tibetan chicken reared at low altitude; LC: Peng County yellow chicken; HLC: Baoxing native chicken reared at high altitude.
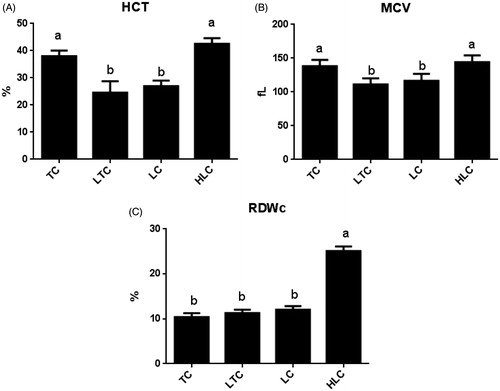
Discussion
The inhabitants adapted to high altitudes, i.e. Bar-headed goose (Anser indicus), plateau pika (Ochotona curzoniae) and yak (Bos grunniens), in the Tibetan Plateau have survived harsh natural selection, have evolved specific mechanisms to address lower oxygen pressure and have obtained genetic adaptation traits to hypoxia from generation to generation (Beall Citation2006; Scott and Milsom Citation2007; Ji et al. Citation2012). Each group carried a subset of the genetic variation from its ancestral population, and then spread to other regions of the world by migration. The high-altitude adaptation birds’ offspring inherited most of genetic material from their ancestors. Ancestral inheritance provided an important foundation to adapt to high altitude. The available research suggested that birds are generally more tolerant than mammals to acclimatisation. For example, at an altitude of 6100 m, house sparrows are alert and active and exhibit normal behaviour. In contrast, mice exposed to the same altitude are comatose (Tucker Citation1968). Bar-headed geese normally migrate over the Himalayan mountains and have been sighted flying over the summit of Mt. Everest (8848 m) (Swan Citation1961; Swan Citation1970). These birds may begin their migration near sea-level and reach altitudes near 9000 m in less than one day, allowing essentially no time for acclimatisation. In addition, the changes of blood characteristics play a key role in mediating an adaptive response to high-altitude hypoxia. During continuous exposure to high-altitude, animals make several physiological changes to survive in hypoxic environments (Zhang et al. Citation2007). In a previous study, an increase in RBC numbers is one of the most important haematological acclimatizations appearing after 1–2 weeks (Monge and Leon-Velarde Citation1991). When lowland animals enter the plateau, hypoxia causes the number of RBC and HGB content to increase (Wideman et al. Citation2002). A previous study showed that native white-tailed ptarmigan reared at high altitude had higher HCT than white-tailed ptarmigan reared at low altitude (Carey and Martin Citation1997).
In the present study, we found significant differences in the WBC and RBC values between TC and other chicken populations. When TCs were reared at low altitude, there was a significant (p < .05) decrease in the WBC and RBC values compared with TCs reared at high altitude. WBCs are part of the body’s immune system and help fight infection, thus protecting against infection and disease. High-altitude environments pose numerous challenges to animal life. The physical environment changes dramatically on ascent, with declines in oxygen availability, temperature, air density and humidity (Scott Citation2011). TC had higher levels of WBC than other chicken populations to resist the severe environmental conditions in order to survive. The results indicated that in a hypoxic environment, TC has higher WBC and RBC values to resist the harsh environment of the plateau. However, TCs reared at low altitude had lower levels of WBC and RBC than TC. The results showed that TCs do not need to increase WBC and RBC values to resist insufficient oxygen and low pressure in blood in a normoxic environment, and these results were consistent with the previous study.
HGB is the protein in RBC that delivers oxygen to all tissues. This process is possible through RBC containing HGB, which combines easily with oxygen. With respect to physiology, the oxygen affinities of HGB change in response to barometric pressure and hypoxia, but high-elevation taxa such as the TC possess HGB with a higher baseline affinity for oxygen. Species that are well-adapted to high altitude, such as the TC, Bar-headed goose and the Andean goose, which may reside permanently at 6000 m, possess HGB with a relatively high oxygen affinity compared with lowland animals (Petschow et al. Citation1977; Hiebl et al. Citation1987). Above all else, the oxygen affinity characteristics of the HGB molecule appear to be one of the most important factors enabling species adapted to high altitudes to transport an adequate supply of oxygen during acute hypoxia. When TCs were reared at low altitude, they also decreased oxygen flux to the mitochondria as a result of physical conditioning. In a previous study, researchers found that when the chickens were maintained at high altitude, they all had increases in RBC, HCT and HGB induced by hypobaric hypoxia. An increase in RBC and HGB increases the oxygen-carrying capacity of blood and acts as a compensatory mechanism to the stimulus of reduced oxygen saturation (Yersin et al. Citation1992). However, we found that there was a significant (p < .05) decrease in the HGB, MCH and MCHC values in TC compared with other chickens (TC, LTC and LC). The oxygen delivery system of chickens functions well across a broad range of oxygen partial pressures and exhibits considerable adaptive plasticity when rapidly exposed to normoxic and hypoxia conditions (Shams and Scheid Citation1993). Usually, if chickens or any non-adapted animals moved to high altitude, they would increase their HGB levels to compensate for the low amount of oxygen. Elevated HGB levels thicken the blood, and the heart struggles to pump the blood around the body; high HGB levels have been linked to complications such as hypertension and chronic mountain sickness. The results showed that HGB, MCH and MCHC values of TC were not high; however, the ability to adapt to low oxygen environments by increasing blood flow velocity is a higher level of adaptation.
One previous study found that the HCT of young adults increased with the increase in altitude, and the correlation was quite obvious (Ge Citation1998). The research results that TC had higher levels of HCT than chickens reared at high altitude (LTC and LC) support this finding. Ye et al. reported that highland native animals had higher RBC numbers and smaller MCV, which are advantageous for oxygen transport. In this study, we found that there was a significant difference in the MCV value between chickens reared at high altitude (TC, HLC) and chickens reared at low altitude (LTC, LC), which was not consistent with previous reports (Bunn and Poyton Citation1996; Wu et al. Citation2005). Whether the MCV value is related to oxygen transport and hypoxic adaptation requires further study. There was a significant increase in the RDWc values in the HLC compared with other chickens (TC, LTC and LC) (p < .05). Computationally, RDWc is the coefficient of variation of MCV; therefore, higher RDWc values reflect greater heterogeneity in MCV (anisocytosis), which is usually caused by perturbation in erythrocyte maturation or degradation.
Conclusions
In conclusion, the results demonstrate that these blood characteristics may be related to the adaptability required in high-altitude environments. In other words, it is likely that the HGB, MCH and MCHC values decrease in high-altitude hypoxic environments. Thus, the increase in WBC, RBC and HCT values may be a common haematological mechanism for TCs to adapt to high-altitude hypoxia. These research results provide the scientific basis for the adaptation of TCs to high-altitude hypoxia.
Disclosure statement
No potential conflict of interest was reported by the authors.
Additional information
Funding
References
- Beall CM. 2006. Andean, Tibetan, and Ethiopian patterns of adaptation to high-altitude hypoxia. Integr Comp Biol. 46:18–24.
- Beall CM. 2007. Two routes to functional adaptation: Tibetan and Andean high-altitude natives. Proc Natl Acad Sci. 104:8655–8660.
- Bishop CM, Spivey RJ, Hawkes LA, Batbayar N, Chua B, Frappell PB, Milsom WK, Natsagdorj T, Newman SH, Scott GR, et al. 2015. The roller coaster flight strategy of bar-headed geese conserves energy during Himalayan migrations. Science. 347:250–254.
- Black CP, Tenney S. 1980. Oxygen transport during progressive hypoxia in high-altitude and sea-level waterfowl. Respir Physiol. 39:217–239.
- Bunn HF, Poyton RO. 1996. Oxygen sensing and molecular adaptation to hypoxia. Physiol Rev. 76:839–885.
- Carey C, Martin K. 1997. Physiological ecology of incubation of ptarmigan eggs at high and low altitudes. Wildlife Bio. 3:210–218.
- Chappell MA, Snyder L. 1984. Biochemical and physiological correlates of deer mouse alpha-chain hemoglobin polymorphisms. Proc Natl Acad Sci USA. 81:5484–5488.
- Ge M. 1998. Hematocrit of young adults and relation of altitude. Acta Acad Med Bengbu. 24:287–288.
- Gou X, Li N, Lian L, Yan D, Zhang H, Wu C. 2005. Hypoxia adaptation and hemoglobin mutation in Tibetan chick embryo. Sci China C Life Sci. 48:616–623.
- Gou X, Wang Z, Li N, Qiu F, Xu Z, Yan D, Yang S, Jia J, Kong X, Wei Z, et al. 2014. Whole-genome sequencing of six dog breeds from continuous altitudes reveals adaptation to high-altitude hypoxia. Genome Res. 24:1308–1315.
- Hao Z, Changxin W, Yangzom C, Yao L, Zhang L. 2006. Adaptability to high altitude and NOS activity of lung in Tibetan chicken. J China Agric Univ. 11:35.
- Hendrickson SL. 2013. A genome wide study of genetic adaptation to high altitude in feral Andean Horses of the páramo. BMC Evol Biol. 13:273.
- Hiebl I, Braunitzer G, Schneeganss D. 1987. The primary structures of the major and minor hemoglobin-components of adult Andean goose (Chloephaga melanoptera, Anatidae): the mutation Leu-Ser in position 55 of the beta-chains. Biol Chem Hoppe Seyler. 368:1559–1569.
- Huerta-Sánchez E, Jin X, Bianba Z, Peter BM, Vinckenbosch N, Liang Y, Yi X, He M, Somel M, Ni P. 2014. Altitude adaptation in Tibetans caused by introgression of Denisovan-like DNA. Nature. 512:194–197.
- Ji LD, Qiu YQ, Xu J, Irwin DM, Tam SC, Tang NL, Zhang YP. 2012. Genetic adaptation of the hypoxia-inducible factor pathway to oxygen pressure among Eurasian human populations. Mol Boil Evol. 29:3359–3370.
- Li M, Tian S, Jin L, Zhou G, Li Y, Zhang Y, Wang T, Yeung CK, Chen L, Ma J, et al. 2013. Genomic analyses identify distinct patterns of selection in domesticated pigs and Tibetan wild boars. Nat Genet. 45:1431–1438.
- Liu C, Zhang LF, Li N. 2013. The specific expression pattern of globin mRNAs in Tibetan chicken during late embryonic stage under hypoxia. Comp Biochem Phys A. 164:638–644.
- Lorenzo FR, Huff C, Myllymäki M, Olenchock B, Swierczek S, Tashi T, Gordeuk V, Wuren T, Ri-Li G, McClain DA. 2014. A genetic mechanism for Tibetan high-altitude adaptation. Nat Genet. 46:951–956.
- Monge C, Leon-Velarde F. 1991. Physiological adaptation to high altitude: oxygen transport in mammals and birds. Physiol Rev. 71:1135–1172.
- Petschow D, Wurdinger I, Baumann R, Duhm J, Braunitzer G, Bauer C. 1977. Causes of high blood O2 affinity of animals living at high altitude. J Appl Physiol Respir Environ Exerc Physiol. 42:139–143.
- Scott GR. 2011. Elevated performance: the unique physiology of birds that fly at high altitudes. J Exp Biol. 214:2455–2462.
- Scott GR, Hawkes LA, Frappell PB, Butler PJ, Bishop CM, Milsom WK. 2015. How bar-headed geese fly over the Himalayas. Physiology (Bethesda). 30:107–115.
- Scott GR, Milsom WK. 2007. Control of breathing and adaptation to high altitude in the bar-headed goose. Am J Physiol Regul Integr Comp Physiol. 293:R379–R391.
- Scott GR, Schulte PM, Egginton S, Scott ALM, Richards JG, Milsom WK. 2011. Molecular evolution of cytochrome c oxidase underlies high-altitude adaptation in the bar-headed goose. Mol Biol Evol. 28:351–363.
- Semenza GL. 2000. HIF-1: mediator of physiological and pathophysiological responses to hypoxia. J Appl Physiol. 88:1474–1480.
- Shams H, Scheid P. 1993. Effects of hypobaria on parabronchial gas exchange in normoxic and hypoxic ducks. Respir Physiol. 91:155–163.
- Storz JF, Moriyama H. 2008. Mechanisms of hemoglobin adaptation to high altitude hypoxia. High Alt Med Biol. 9:148–157.
- Sun J, Zhong H, Chen SY, Yao YG, Liu YP. 2013. Association between MT-CO3 haplotypes and high-altitude adaptation in Tibetan chicken. Gene. 529:131–137.
- Swan LW. 1961. The ecology of the high Himalayas. Sci Am. 205:68–78.
- Swan LW. 1970. Goose of the Himalayas. Nat Hist. 79:68–75.
- Tucker VA. 1968. Respiratory physiology of house sparrows in relation to high-altitude flight. J Exp Biol. 48:55–66.
- Villamor E, Kessels CG, Ruijtenbeek K, Van Suylen, RJ, Belik J, De Mey JG, Blanco CE. 2004. Chronic in ovo hypoxia decreases pulmonary arterial contractile reactivity and induces biventricular cardiac enlargement in the chicken embryo. Am J Physiol-Reg I. 287:R642–R651.
- Wideman R, Erf G, Chapman M, Wang W, Anthony N, Xiaofang L. 2002. Intravenous micro-particle injections and pulmonary hypertension in broiler chickens: acute post-injection mortality and ascites susceptibility. Poult Sci. 81:1203–1217.
- Wu T, Wang X, Wei C, Cheng H, Wang X, Li Y, Zhao H, Young P, Li G, Wang Z. 2005. Hemoglobin levels in Qinghai-Tibet: different effects of gender for Tibetans vs. Han. J Appl Physiol. 98:598–604.
- Yersin A, Huff W, Kubena L, Elissalde M, Harvey R, Witzel D, Giroir L. 1992. Changes in hematological, blood gas, and serum biochemical variables in broilers during exposure to simulated high altitude. Avian Dis. 36:189–196.
- Zhang H, Wu C, Chamba Y, Ling Y. 2007. Blood characteristics for high altitude adaptation in Tibetan chickens. Poult Sci. 86:1384–1389.
- Zhang H, Wu C, Qiangba Y, Ma X, Tang X, Pu B. 2004. Curve analysis of embryonic mortality in chickens incubation at high altitude. J China Agric Univ. 10:109–114.