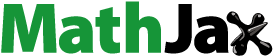
ABSTRACT
The mechanical properties of living cells reflect their propensity to migrate and respond to external forces. Both cellular and nuclear stiffnesses are strongly influenced by the rigidity of the extracellular matrix (ECM) through reorganization of the cyto- and nucleoskeletal protein connections. Changes in this architectural continuum affect cell mechanics and underlie many pathological conditions. In this context, an accurate and combined quantification of the mechanical properties of both cells and nuclei can contribute to a better understanding of cellular (dys-)function. To address this challenge, we have established a robust method for probing cellular and nuclear deformation during spreading and detachment from micropatterned substrates. We show that (de-)adhesion kinetics of endothelial cells are modulated by substrate stiffness and rely on the actomyosin network. We combined this approach with measurements of cell stiffness by magnetic tweezers to show that relaxation dynamics can be considered as a reliable parameter of cellular pre-stress in adherent cells. During the adhesion stage, large cellular and nuclear deformations occur over a long time span (>60 min). Conversely, nuclear deformation and condensed chromatin are relaxed in a few seconds after detachment. Finally, our results show that accumulation of farnesylated prelamin leads to modifications of the nuclear viscoelastic properties, as reflected by increased nuclear relaxation times. Our method offers an original and non-intrusive way of simultaneously gauging cellular and nuclear mechanics, which can be extended to high-throughput screens of pathological conditions and potential countermeasures.
Introduction
The mechanical properties of the extracellular matrix (ECM) regulate important physiological processes in eukaryotic cells, such as spreading, differentiation, division or motility. An important factor in this mechanical-functional interplay is the reorganization of the actin cytoskeleton, which controls intracellular pre-stress.Citation1 However, the exact molecular mechanisms that underlie substrate-dependent mechanotransduction remain elusive. Recently, Swift and co-workers have shown that the matrix stiffness increases nuclear lamin-A levels, thereby stabilizing the nucleus while also contributing to lineage determination.Citation2 A-type lamins and the linker of the nucleoskeleton and cytoskeleton (LINC) complex directly transmit forces from the extracellular matrix into the nucleus. Mechanical forces lead to changes in the molecular structure and assembly of A-type lamins that in turn activate a tension-induced “inside-out signaling.” This process allows feedback from the nucleus to the cytoskeleton and the extracellular matrix in order to balance ‘outside-in’ forces. Citation3 This finding suggests that the LMNA gene is mechanoresponsive to ECM elasticity and underlines the importance of studying the relationship between the cytoskeletal organization and the nucleoskeletal homeostasis.
The ability to measure mechanical properties of adherent cells relies on a toolbox of cell mechanical measurement techniques that can apply forces or deformations on adherent cells (e.g. cell-stretchers,Citation4 atomic force microscopy,Citation5 magnetic tweezers,Citation6 parallel plates,Citation7 microfluidic devices,Citation8 optical tweezersCitation9), whereas the characterization of the mechanical properties of the nucleus requires local mechanical techniques (i.e. endogenous particlesCitation10 or a micropipette aspiration methodCitation10,11). While all of these methods have proven extremely powerful in characterizing cellular or nuclear viscoelastic properties, none of these techniques allows to probe simultaneously and non-invasively the mechanical properties of the cell and the nucleus.Citation12
To address this challenge, we propose to use cell-ECM adhesion and detachment (in other terms (de-)adhesion) kinetics, for characterizing combined cellular and nuclear mechanical properties. In line with work from Wildt and coworkers, who have developed surfaces composed of RGD-functionalized arrays of microscale gold strips for studying the detachment dynamics of fibroblasts,Citation13,14 we used culture substrates of different rigidities patterned with protein microfeatures. Our strategy allows to overcome many of the limitations associated with existing methods by controlling the matrix stiffness, the cellular morphology and the spreading area, as they are known to modulate the intracellular force balance andCitation15 the nuclear homeostasis.Citation16,17 Using standardized (de-)adhesion assays, we investigate how changes in matrix stiffness affect the cellular pre-stress and we show that (de-)adhesion dynamics on micropatterned surfaces can be used to investigate the modifications of nuclear mechanics.
Results and discussion
Cell spreading dynamics is determined by matrix stiffness
Individual primary endothelial cells (HUVECs) were deposited on fibronectin (FN)-coated rectangular micropatterns with a 1:10 aspect ratio and a surface area of 1200 µm2. The entire spreading process, from the contact of the cell with the adhesive micropattern (t = t0) to the maximum cell spreading state (t = tf), was followed with time-lapse microscopy (, Supplementary Movie S1). In their final state, completely spread endothelial cells were characterized by focal adhesions localized at both extremities of the FN micropattern,Citation16 and thick actin stress fibers parallel to the long axis of the rectangular pattern, as evidenced by phalloidin and vinculin stainings, respectively ().
Figure 1. Cell spreading on elongated adhesive patterns. (A) Side and (B) top views of a cell spreading on a rectangular fibronectin micropattern of 1:10 aspect ratio. (C) Side and (D) top views of an immunostained endothelial cell spread on a rectangular micropattern (1:10 aspect ratio) deposited on a stiff (E = 3MPa) PDMS substrate. The actin cytoskeleton (phalloidin) is labeled in green, focal adhesions (vinculin) in red and the nucleus (DAPI) in blue. Scale bars are 10 µm. (E) Temporal evolution of the normalized cell spreading area on rectangular adhesive micropatterns (aspect ratio 1:10) deposited on 5 kPa (in blue, n = 9), 9 kPa (in red, n = 8) and 3 MPa (in black, n = 11) culture substrates. (F) Mean characteristic spreading times, ts, on 5kPa (in blue, n = 9), 9kPa (in red, n = 8) and 3 MPa (in black, n = 11) substrates. Data are mean ± SD, *p ≤ 0 .05, ** p ≤ 0 .01 and *** p ≤ 0 .001.
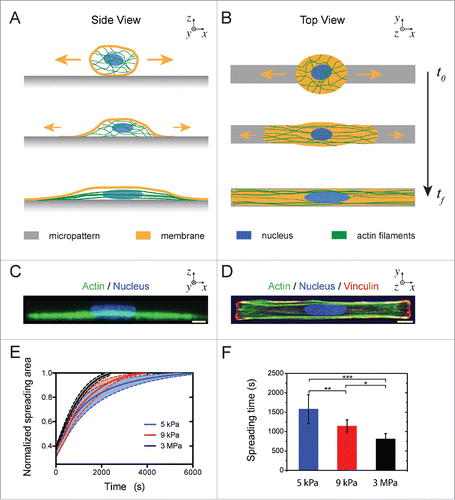
Using culture substrates of varying stiffnesses, we studied the effect of the matrix rigidity on the dynamics of cell spreading on 1:10 rectangular micropatterns (Supplementary Movie S2). As shown in , the spreading process followed a sigmoidal behavior and was longer on 5 kPa (tf = 5940 ± 170 s) than on 3 MPa (tf = 3230 ± 210 s) substrates. The cellular deformation, ϵs(t), during the spreading stage was recorded by optical microscopy in DIC mode and spreading curves for different matrix stiffnesses were fitted with a sigmoidal function (EquationEq. 2(2)
(2) ) to estimate the characteristic spreading time, ts. We found that ts decreased with increasing matrix rigidity and was 2 times higher on 5 kPa substrates (1582 ± 434 s) than on 3 MPa (814 ± 136 s) substrates (). Together, our results demonstrate that the spreading process of endothelial cells is significantly affected by the matrix stiffness. These findings are in agreement with the recent observations of Nisenholz et al. that claimed that both the initial spreading rate and steady state of fibroblasts increase on substrates with increasing stiffness.Citation18
Cellular relaxation dynamics is modulated by matrix stiffness
Endothelial cells spread on adhesive micropatterns undergo a natural strain in order to adopt the 1:10 aspect ratio imposed by the pattern geometry (). Assuming that the spatial organization of the actin cytoskeleton in elongated endothelial cells () leads to a large amount of cell contractility,Citation16 we investigated whether the matrix stiffness can modulate the tension in contractile actomyosin filaments by quantifying the relaxation dynamics after cell detachment. To do this, endothelial cells were grown 24 hours on FN-coated micropatterns and then detached by adding the proteolytic enzyme Accutase. Cell detachment leads to a fast cellular relaxation (Supplementary Movie S3), as monitored by time-lapse microscopy in DIC mode ().
Figure 2. Cell relaxation dynamics. (A) Time-lapse sequence in DIC mode of the cell relaxation process after detachment with Accutase (t = 0) of an endothelial cell spread on an elongated micropattern (1:10 aspect ratio, depicted in white) deposited on a stiff (E = 3 MPa) substrate. The scale bar is 10 µm. (B) Evolution of the normalized cell deformation as a function of time after initiation of the detachment from an elongated pattern on a stiff substrate. The red curve corresponds to a sigmoidal fit with Eq 4. DIC kymographs of the relaxation of an endothelial cells detached from a (C) 5 kPa and a (D) 3 MPa micropatterned substrates. The slopes on kymographs represent cell relaxation velocity after detachment on 5 kPa (blue line) and 3 MPa (black line), respectively. (E) Evolution of the characteristic cell relaxation time as a function of the matrix stiffness (n = 13 for 5 kPa; n = 18 for 9 kPa; and n =15 for 3MPa). Data are expressed as mean ± SD, *p ≤ 0 .05, ** p ≤ 0 .01 and n.s. non significant.
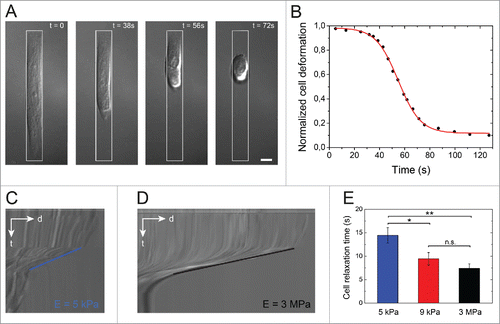
By fitting cell relaxation curves () with a sigmoidal function (EquationEq. 4(4)
(4) ), we found that for a given substrate stiffness the characteristic relaxation time, tR, was 2 orders of magnitude lower than the characteristic spreading time. The slopes on DIC kymographs showed clearly that the detachment kinetics on soft 5 kPa matrix (∼0.26 µm/s, ) was slower than on stiff 3 MPa substrates (∼0.73 µm/s, ). In line with this observation, we found that the characteristic relaxation time decreased significantly with increasing matrix stiffness, from tR ∼ 15 s for cells spread on a 5 kPa matrix, to tR ∼10 s for cells spread on 9 kPa and tR ∼7 s MPa substrate (). These results suggest that cell relaxation dynamics can be considered as an indirect readout for the rigidity of the cell microenvironment, especially in the low rigidity range. Taken together, these results suggest that the relaxation dynamics relies on internal modifications of the cytoskeletal organization, i.e., a form of pre-stress, which were driven by changes in the matrix stiffness during the spreading stage.
Cell relaxation dynamics reflects the initial cellular pre-stress
To assess whether endothelial relaxation dynamics was related to the tensile state of the cytoskeleton, we decreased the intracellular pre-stress by depolymerizing the actin network with Latrunculin A (Lat-A).Citation19 Our findings show that Lat-A-treated cells relaxed more than 15 times slower than control cells (), supporting our hypothesis that the relaxation process reflects the state of tensile stress. In addition, micropatterned endothelial cells were treated with Y-27632, a selective inhibitor of Rho-associated kinase (ROCK).Citation20 The ROCK family of kinases has been shown to be involved in Rho-induced formation of actin stress fibers and focal adhesionsCitation21 and in down-regulation of myosin phosphatase.Citation22 As shown in , Y-27632-treated cells exhibited a significant slowing down of their relaxation dynamics. Taken together, our results suggest that the contractile actomyosin cytoskeleton () is the driving force of the cell relaxation process.
Figure 3. Role of the actomyosin cytoskeleton on cell relaxation dynamics. (A) Cellular relaxation times (mean ± SD) for control endothelial cells (black bar, n = 18), Latrunculin A-treated cells (white bar, n = 14) and Y-27632-treated cells (dashed bar, n=15) deposited on 3 MPa micropatterned substrates. Data are expressed as mean ± SD and ***p ≤ 0 .001. (B) Typical fluorescent images of the spatial distribution of the actin cytoskeleton (in green), the nucleus (in blue) and the myosin (in white) of endothelial cells plated on 1200 µm2 and 1:10 aspect ratio rectangular micropatterns deposited on 3MPa substrates. Scale bars are 10 µm. (C) Semi-logarithmic evolution of the cell elastic modulus of endothelial cells spread on elongated patterns deposited on substrates with increasing stiffnesses (5 kPa, 9 kPa, 45 kPa, 110 kPa and 3 MPa). (D) Evolution of the characteristic relaxation time as a function of the cell elastic modulus.
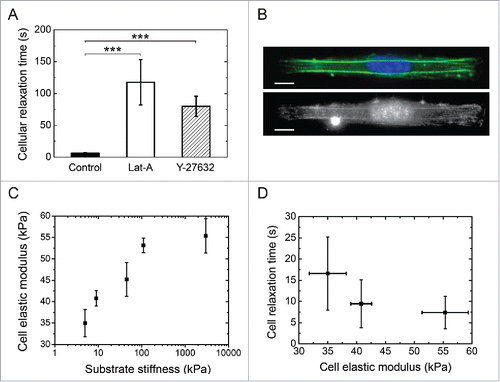
To directly assess the intracellular pre-stress, we determined the elastic modulus (E) of micropatterned endothelial cells grown on micropatterned substrates ranging from 5 kPa to 3 MPa with magnetic tweezers.Citation6,23 Briefly, magnetic beads were coated with fibronectin and incubated with patterned cells in order to bind the magnetic beads to the cytoskeleton through formation of focal adhesions.Citation6 A constant magnetic pulling force was applied to the beads during 12 s and the elastic modulus of living micropatterned cells was estimated with EquationEq. 6(6)
(6) by using bead displacements that were recorded with time-lapse microscopy. As presented in , our findings demonstrated that the elastic modulus of patterned endothelial cells increased with increasing matrix rigidity, in agreement with previous reports on fibroblasts.Citation24,25 By combining these results, we show that the cell relaxation time decreased with the cell elastic modulus (), confirming that the cell relaxation dynamics reflects the internal tension of endothelial cells and can be used as a reliable parameter to characterize the pre-stress in adherent cells.
Cell shape changes induce instantaneous and reversible adaptations of the nuclear shape
Next, we investigated the dynamics of nuclear deformation by following H2B-GFP nuclei during spreading () and relaxation () stages with time-lapse microscopy (see Methods section). As shown in , the nucleus changes its shape during the cell spreading stage, from a spherical form to an elongated shape, as a result of intracellular forces acting on the nucleus due to the remodeling of the spatial organization of the actin cytoskeleton. The nuclear deformation followed a similar temporal evolution as the cellular deformation (), suggesting that nuclear and cellular deformations are coupled processes. Similarly, cellular relaxation after cell detachment is also associated with a rapid nuclear relaxation leading to nuclear rounding. As shown in , the nuclear deformations that occur during the cellular relaxation stage (Supplementary Movie S4) were at least one order of magnitude faster than the nuclear deformations during the spreading stage. Changes in H2B-GFP intensity patterns also suggested chromatin reorganization during spreading and relaxation stages, with the formation of condensed chromatin domains in deformed nuclei, as reflected by changes in the fluorescence intensity (). Modifications of the nuclear shape that take place in hundreds of minutes during the spreading stage induce marked reorganization of chromatin distribution (), which are relaxed in a few dozen of seconds during cell detachment.
Figure 4. Evolution of the nuclear deformation during cellular spreading and relaxation processes. (A) Image sequence of the cellular (DIC) and the nuclear (DAPI) deformations during the cell spreading stage on an elongated micropattern (depicted in red). A schematic representation of the cell for each step is indicated for clarity. (B) DIC and DAPI images of a relaxed cell and its nucleus, respectively, after cell detachment with Accutase. (C) Color-coded fluorescent images (H2B-GFP labeling) of the nucleus of an endothelial cell spreading on a 3MPa micropatterned substrate. The scale bar represents 10 µm. (D) Color-coded fluorescent images (H2B-GFP labeling) of the nucleus of an endothelial cell relaxing from a 3MPa micropatterned substrate after detachment with Accutase. The scale bar represents 10 µm. (E) Typical evolution of cellular (in black) and nuclear (in blue) deformations during spreading and relaxation stages on a 3MPa micropatterned substrate. (F) Schematic representation of the reorganization of chromatin during the slow (∼min.) nuclear deformation associated to cell spreading and the fast (∼s) nuclear relaxation after cell detachment.
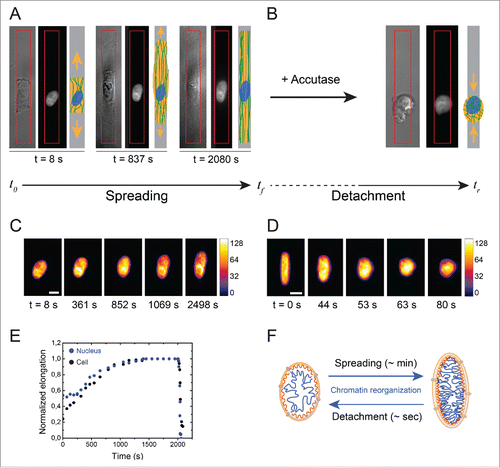
Nuclear relaxation dynamics is determined by the cell state
We quantified the dynamics of nuclear relaxation as a function of the matrix stiffness (E = 5 kPa, 9 kPa and 3 MPa) by performing time-lapse experiments of H2B-GFP labeled nuclei. As presented in , our results showed that nuclear relaxation dynamics was significantly affected by the stiffness of the substrate. Indeed, the mean characteristic relaxation time decreased from ∼20 s on 5 kPa matrices to ∼9 s on a 3MPa substrate, emphasizing that the nuclear relaxation is intimately related to the cell relaxation process. This was further supported by the nuclear relaxation time of Y-27632-treated cells which was 8 times slower than controls ( and Supplementary Movie S5), suggesting that the nuclear relaxation dynamics is modulated by substrate-induced changes in actomyosin tension.
Figure 5. Dynamics of nuclear relaxation. Evolution of the characteristic nuclear relaxation time as a function of (A) the matrix stiffness (E = 5 kPa n = 5, 9 kPa n = 6 and 3 MPa n = 7) and (B) a Y-27632 treatment that affects the actomyosin contractility (control cells: n = 7 and Y-27632 treated cells: n = 5). Data are expressed as mean ± SD, **p < 0.01 and n.s. non significant.
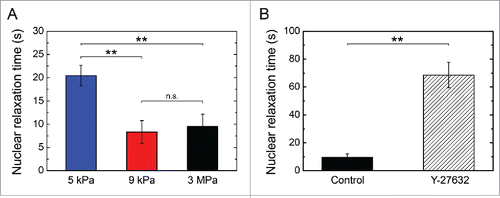
Our results demonstrate that cell detachment from adhesive protein micropatterns allows to probe the nuclear mechanics without applying external forces and without any alteration of the nucleo-cytoskeletal interactions, such as observed in micropipette aspiration experiments on isolated nuclei.Citation11
Lamin A impacts on nuclear relaxation dynamics
The balance of A-type and B-type lamins has been shown to affect the rheological properties of the nucleus and alteration of the composition of the nuclear lamina are encoutered in different diseases such as laminopathies.Citation26,27 Indeed, micropipette aspiration experiments have shown that nuclei with a low expression of lamin A were more compliant than those with a high expression of lamin A.Citation2,28 Cells lacking A-type lamins have a reduced nuclear stiffness and an increased nuclear fragility, leading to more cell death under mechanical strain.Citation29,30 In contrast, accumulation of farnesylated prelamin A, as encountered in restrictive dermopathy and Hutchinson–Gilford Progeria Syndrome (HGPS), may lead to a lower deformability of the nucleus under mechanical stress.Citation30,31
We investigated the role of the nuclear lamina in the nuclear shape relaxation dynamics by treating HUVECs spread on 3 MPa micropatterned substrates with the HIV protease inhibitor saquinavir, which inhibits ZMPSTE24, the protease that catalyzes conversion of farnesylated prelamin A to prelamin A.Citation32 As shown in , the nuclei of saquinavir-treated cells were statistically less deformed (NSI ∼ 0.86) than the nuclei of control cells (NSI ∼ 0.79). Our results are in agreement with the observation of Dahl and coworkers that the altered nuclear lamina in HGPS induces an increased resistance of the nucleus to mechanical stress.Citation27 As shown in , immunostaining of the actin network and lamin A/C shows that saquinavir-treated cells present an intact actin cytoskeleton. Saquinavir-treated cells present a broader distribution of their characteristic nuclear relaxation times, with 70% of nuclei relaxing slower than the mean relaxation time of control nuclei (). In contrary, saquinavir treatment did not influence the cell relaxation time. Together, our results indicate that accumulation of farnesylated prelamin A in saquinavir-treated cells leads to a modification of the viscoelastic properties of the nucleus, which leads to less deformed nuclear shapes and an increase of the nuclear relaxation dynamics. Our results are consistent with the hypothesis that the characteristic relaxation time of the nucleus (tN) reflects the viscoelastic properties of the nucleus,Citation34 that depend on the ratio of lamin A:B isoforms.Citation2,28 Modifications of the ratio between lamin-A which contributes as a highly viscous fluid (η) and lamin-B which acts as an elastic solid (G) lead therefore to changes of the nuclear relaxation time (tN∼η/G). This illustrates the performance of our approach and positions (de-)adhesion dynamics as a powerful technique to differentiate living cells based on the composition of their nuclear lamina.
Figure 6. Role of the nuclear lamina on nuclear relaxation dynamics. (A) Characterization of the nuclear shape of control (in black, n = 44) and saquinavir-treated cells (in gray, n = 41) plated on a 3 MPa micropatterned substrate. Mean ± SD and ***p ≤ 0 .001. (B) Immunostaining images of the actin cytoskeleton (in green), the nucleus (in blue) and the lamin A/C (in white) of rectangular micropatterned endothelial cells treated for 3 d with the protease inhibitor saquinavir after plating and then treated with fresh saquinavir for 24h before fixation and immunostaining. Scale bar is 10 µm. (C) Characteristic nuclear (n = 11, in red) and cellular (n = 11, in black) relaxation time of endothelial cells grown on rectangular micropatterns and treated for 4 d with the protease inhibitor saquinavir (20 µM). ** p ≤ 0 .01 and n.s. non significant.
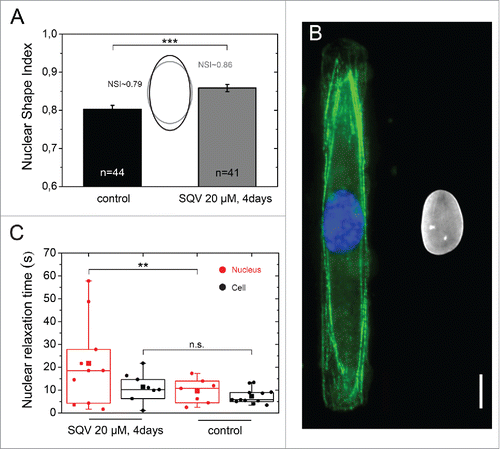
Conclusions
We have developed a simple and efficient assay to probe cellular and nuclear mechanical properties based on (de-)adhesion kinetics. By combining protein micropatterns with culture substrates of different stiffnesses, we standardized the shape and the spreading area of single endothelial cells, while modulating their internal pre-stress. The dynamics of detachment reflects the amount of pre-stress accumulated in the actomyosin network during the spreading stage. In addition, our technique allows characterizing the dynamics of nuclear deformations during cell spreading and detachment. Large deformations of the nucleus occur in hundreds of minutes during the spreading stage, whereas the nuclear shape and condensed chromatin can be relaxed in a few dozens of seconds after the cell detachment. Finally, our results indicate that chemically induced accumulation of farnesylated prelamin A causes a nuclear stiffening, which is reflected by less deformed nuclei and an increase of their relaxation dynamics. We envision that this simple and robust technique can be suitable for investigating the role of complex structures such as LINC complexes that link the actin cytoskeleton and the nuclear lamina and for the diagnosis of human diseases involving cytoskeletal and/or nuclear modifications.
Materials and methods
Rigidity-controlled substrate preparation
Culture substrates in polydimethylsiloxane (PDMS) silicone elastomer, (Sylgard 184 Silicone Elastomer Kit; Dow Corning, Midland, MI) of 3.0 ± 0.3 MPa Young's modulus were prepared from 1:10 cross-linker to monomer ratio. The uncured solution of PDMS was mixed and degassed in a conditioning mixer and then spin-coated onto a cleaned glass coverslip with a rotating speed gradually increasing from 500 to 5000 rpm, for a total duration of 2 min. Then PDMS substrates were cured for 4h at 60°C. Culture substrates in hydroxy-polyacrylamide (hydroxy-PAAm) hydrogels of 5.2 ± 0.2 kPa and 9.0 ± 0.3 kPa Young's moduli were prepared on circular glass coverslips of 25 mm in diameter. Glass coverslips were cleaned with a 0.1 M NaOH (Sigma, Saint-Louis, MO) solution during 5 min, rinsed abundantly (20 min under agitation) with deionized water, then treated during one hour with 3-(trimethoxysilyl)propyl acrylate (Sigma, Saint-Louis, MO) to promote strong adhesion between the PAAm gel and glass coverslips and finally gently dried under a nitrogen flow. In a 15 mL Eppendorf tube, 400 µL acrylamide, 40% w/w in HEPES (AAm, Sigma, Belgium), 50 or 250 µL N,N’-methylenebisacrylamide, 2% w/w in HEPES (BisAAm, Sigma, Saint-Louis, MO), and 1065 µL N-hydroxyethylacrylamide monomers (65 mg.mL-1 in HEPES, Sigma, Saint-Louis, MO) were mixed and the desired volume of a solution of 4-(2- hy droxyethyl)-1-piperazineethanesulfonic acid 50 mM (HEPES, Sigma, Saint-Louis, MO) was added to reach a final volume of 5 mL and Young moduli of 5 or 9, respectively. After degassing the mixture during 20 min under vacuum, the polymerization was started by adding 2.5 µL of N,N,N′,N′-tetramethylethylenediamine (TEMED, Sigma, Saint-Louis, MO) and 25 µL of ammonium persulfate solution (APS, 100 mg/ml w/w in deionized water, Sigma, Saint-Louis, MO). A volume of 50 µL of this mixture was deposited on a 25 mm diameter glass coverslip and then a 22 mm diameter glass coverslip was placed upon the solution. After 30 min, the polymerization was completed and the 22 mm diameter coverslip was gently removed under water to obtain ∼150 µm thick PAAm hydrogels. Finally, PAAm hydrogels were washed 3 times in sterile deionized water and stored at 4°C in sterile phosphate buffer saline (PBS).
Microcontact printing
Rectangular (1:10 aspect ratio) micropatterns of 1200 μm2 were drawn with the Clewin software (WieWeb Software, The Netherlands) and generated to a silicon master by deep reactive-ion etching (FH Vorarlberg University of Applied Sciences, Microtechnology, Dornbirn, Austria). The silicon surface was passivated under vacuum with vapors of fluorosilane (tridecafluoro-1,1,2,2-tetrahydrooctyl-1-trichlorosilane) for 30 min, and then molded with a thick layer of PDMS (Sylgard 184 Silicone Elastomer Kit; Dow Corning, Midland, MI). After 4h of curing at 60°C, the PDMS membrane was peeled off and stamps of 1 × 1 cm2 were cut manually. The structured surface of the PDMS stamps were made temporarily hydrophilic in an UV/O3 cleaner for 8 min and then inked for 1 h at room temperature with a 25 μg/ml fibronectin (FN) solution from human plasma. FN-coated stamps were dried under filtered nitrogen and gently deposited on a flat rigidity-controlled substrate for 1 hour (hydroxy-PAAm hydrogels) or 15 seconds (PDMS elastomers). Uncoated regions were then blocked by incubating hydroxy-PAAm hydrogels overnight in a 5 mg/ml BSA solution at 4°C and elastomers for 5 min in a 1% solution of Pluronic F-127.
Cell culture
Primary human umbilical vein endothelial cells, HUVECs (Cells Applications, San Diego, CA) were grown in complete endothelial cell growth medium (Cells Applications, San Diego, CA) supplemented with 1% Penicillin/Streptomycin and antimycotics and maintained at 37°C in a humidified atmosphere with 5% CO2. Cells between passages 2 and 8 were cultured on micropatterned PDMS coverslips at a concentration of about 15,000 cells/ml.
Live cell spreading
Cells were detached from a routine culture flask with a solution of Accutase, then suspended in fresh culture medium to a final concentration of 15 000 cells/ml and then transferred to a micropatterned rigidity-controlled substrate. The cellular spreading process on elongated micropatterns was recorded in DIC mode via time-lapse microscopy with a Roper QuantEM:512SC EMCCD camera controlled by NIS Elements Advanced Research 4.0 software (Nikon). Images were recorded every 2 sec. for at least 2 hours. Using an electron-multiplying CCD camera, low light conditions were used for living cell spreading experiments in order to minimize the risk of potential damage of light illumination on cells.
The dynamics of cell spreading on rectangular micropatterns was estimated by measuring the evolution of the projected cellular area, AC(t), as a function of the time. The normalized cellular spreading area, ϵs(t), was obtained by dividing the cellular area, AC(t), by the micropattern area (1200 µm2), AM, according to the equation:(1)
(1) Considering a mean cell diameter of 17.78 ± 2.62 µm as determined by FACS (Fig. S1), ϵs(t) varied therefore between 0,2 (t = t0) and 1, corresponding to the full spreading state when the cell entirely covers the micropattern area at t = tf.
The characteristic spreading time was determined by fitting ϵs(t) with a sigmoidal curve, as:(2)
(2) where A1 is the maximal cell spreading area at t = tf, A2 is the minimal cell spreading area at t = t0, tc is the center of the sigmoid and ts corresponds to the characteristic spreading time.
Live cell relaxation
Cells grown on rigidity-controlled micropatterned (1:10 aspect ratio) substrates for 24 hours were observed with a Nikon Eclipse Ti-E motorized inverted microscope equipped with ×10 Plan Apo, ×40 Plan Apo (NA 1.45, oil immersion), ×60 Plan Apo (NA 1.45, oil immersion) and ×100 Plan Apo (NA 1.45, oil immersion) objectives and with a temperature and CO2 level controller. The relaxation process of the elongated cells was induced by adding 1 ml of Accutase after a gentle wash of warm PBS (37°C) and recorded in DIC mode via time-lapse microscopy with a Roper QuantEM:512SC EMCCD camera (Photometrics, Tucson, AZ) controlled by NIS Elements Advanced Research 4.0 software (Nikon, Japan). Images were recorded every 500 ms up to complete cell detachment.
The dynamics of the cellular relaxation, ϵR(t), was determined by normalizing the temporal evolution of the cellular length, L(t) with the long axis of the micropattern, LM, as:(3)
(3) Considering that LM = 109.5 µm for a 1:10 micropattern of 1200 µm2 and an initial mean cell diameter of 17.78 ± 2.62 µm, ϵR(t) varied between 1 (full spreading state) and 0,16 (total cell relaxation).
The temporal evolution of the normalized cell deformation, ϵR(t), during the relaxation stage was fitted with a sigmoidal curve, according to:(4)
(4) where A1 is the maximal cellular deformation before the initiation of the detachment process, A2 is the minimal cell strain obtained after cell relaxation, tC is the center of the sigmoid and tR corresponds to the characteristic cell relaxation time.
Nuclear deformation
To observe nuclear DNA in live imaging, HUVECs were transduced with the BacMam 2.0 CellLight Histone 2B-GFP according to the manufacturer's protocol (Life Technologies, Molecular Probes). Routine culture flasks were incubated with approximately 20 BacMam particles/cell for at least 24 hours. Labeled cells were then detached and plated on rigidity-controlled micropatterned substrates to perform spreading and detachment experiments. The nuclear shape index (NSI) was defined as NSI = 4 ×area/(perimeter)2. The nuclear morphology was characterized with time-lapse fluorescence microscopy and the nuclear deformation, ϵN(t), during spreading and detachment experiments was fitted with a sigmoidal curve, according to:(5)
(5) where A1 is the maximal nuclear deformation, A2 is the minimal nuclear deformation, tc is the center of the sigmoid and tN corresponds to the characteristic relaxation time of the nucleus. The mean nuclear diameter was characterized by FACS as 12.42 ± 2.42 µm.
Pharmacological treatment
In order to assess the effect of the cytoskeletal organization on cellular and nuclear relaxation dynamics, endothelial cells were treated with ROCK-inhibitor Y-27632 (Sigma-Aldrich, 30 µM) or the actin polymerization inhibitor Latrunculin-A (Sigma-Aldrich, 0.5 µM) 1 hour prior detachment with Accutase. The HIV protease inhibitor Saquinavir (Sigma-Aldrich, 20µM) was used to selectively block the human ZMPSTE24 enzyme, leading to accumulation of farnesylated prelamin A. Endothelial cells were treated during 4 d with a 20 µM solution of saquinavir.
Cell fixation and immunostaining
Endothelial cells were fixed with a solution of 4% paraformaldehyde (Electron Microscopy Sciences, Hatfield, PA) for 10 min, permeabilized with 0.05% Triton X-100 (Sigma) in PBS (PAA Laboratories) for 15 min at 37°C and washed 3 times in PBS. Cells were incubated for 45 min at 37°C with 4,6-diamidino-2-phenylindole dihydrochloride 1/200 (DAPI, Molecular Probes, Life Technologies, Cergy Pontoise, France), Alexa Fluor 488 labeled Phalloidin 1/200 (Molecular Probes, Life Technologies), monoclonal mouse anti-vinculin antibody (1/200, HVIN-1 clone, Sigma-Aldrich), mouse anti-lamin A/C antibody (1/200, Sigma-Aldrich) or rabbit anti-myosin light chain antibody (1/300, Sigma-Aldrich) washed 3 times in PBS, and incubated for 45 min at 37°C with a goat anti-mouse antibody labeled with tetramethylrhodamine (Molecular Probes, Invitrogen) or with a goat anti-rabbit antibody labeled with tetramethylrhodamine (Sigma-Aldrich, T6778). Both secondary antibodies were used at a concentration of 1/200 for 45 min at 37°C. Slides were mounted in Slow Fade Gold Antifade (Molecular Probes, Invitrogen).
Measurement of cell stiffness by magnetic tweezers
BcMagTM Epoxy-Activated Magnetic beads (Bioclone Inc., San Diego, CA) of 4.5 μm in diameter were coated with human fibronectin (FN) by following the bead manufacturer's protocol for bead functionalization. Briefly, beads were first rinsed in phosphate buffered saline (PBS) and subsequently incubated on ice in 10 μg/mL human FN overnight under a gentle (0.5 Hz) agitation to disperse beads without precipitating FN still in solution. After rinsing in PBS, FN-coated beads were seeded onto cells plated on micropatterned rigidity-controlled substrates in culture medium for 20 minutes at a mean ratio of 4 beads per cell. A home-made magnetic tweezer mounted on an inverted Nikon Eclipse Ti microscope (Nikon, Japan) and connected to a LabVIEW software (National Instruments, Austin, Texas) was positioned at a 35-degree angle to the microprinted culture substrate by using an automated micromanipulator system (InjectMan NI2, Eppendorf, Hamburg, Germany).Citation6 The magnetic forces were calibrated using the Stokes' law by quantifying the beads displacements in a 99% glycerol solution as a function of the current intensity and the bead-tip distance. A constant pulling force, F, was then applied to paramagnetic beads of section S and radius R bounded to the cytoskeleton of the micropatterned endothelial cells. The cell elastic modulus, E, was estimated from the beads displacements, ∂, as Citation33(6)
(6) where S = 4πR2 is the bead surface and U is bead translation ∂ normalized by the bead radius R, as U = ∂/R.
Statistical analysis
Differences in means between groups were evaluated by 2-tailed Student's t-tests performed in Origin 8.5 (OriginLab, Northampton, MA). For multiple comparisons the differences were determined by using an analysis of variance (ANOVA) followed by Tukey post-hoc test. *p ≤ 0 .05, **p ≤ 0 .01 and ***p ≤ 0 .001. Unless otherwise stated, all data are presented as mean ± standard deviation (SD).
Disclosure of potential conflicts of interest
No potential conflicts of interest were disclosed.
Supplemental_Materials.zip
Download Zip (87.8 MB)Acknowledgments
The Mechanobiology and Soft Matter group belongs to the French research consortium GDR 3070 CellTiss. The authors would like to thank Frédéric Lhommé for FACS characterization and Sylvain Desprez for substrate stiffness measurements.
Funding
This work was supported by the Wallonia Region (DG06, First Spin Off « Opticell » n° 1510507) and the Fonds National de la Recherche Scientifique F.R.S.-FNRS under Grants « Nanomotility » FRFC n° 2.4622.11, « TIRF Microscopy » n° 1.5013.11F and « Curvedmotility » n° J.0099.16. M.R. is Research Fellow of the F.R.S.-FNRS. T.G., J.L, D.M., C.B. and L.A. doctoral fellowships are supported by the Foundation for Training in Industrial and Agricultural Research (FRIA).
References
- Stamenović D, Liang Z, Chen J, Wang N. Effect of the cytoskeletal prestress on the mechanical impedance of cultured airway smooth muscle cells. J. Appl Physiol 2002; 92:1443-50; PMID:11896008; http://dx.doi.org/10.1152/japplphysiol.00782.2001
- Swift J, Ivanovska IL, Buxboim A, Harada T, Dingal PCDP, Pinter J, Pajerowski JD, Spinler KR, Shin JW, Tewari M, et al. Nuclear lamin-A scales with tissue stiffness and enhances matrix-directed differentiation. Science 2013; 341:1240104-4; PMID:23990565; http://dx.doi.org/10.1126/science.1240104
- Osmanagic-Myers S, Dechat T, Foisner R. Lamins at the crossroads of mechanosignaling. Genes Dev 2015; 29:225-37; PMID:25644599; http://dx.doi.org/10.1101/gad.255968.114
- Guck J, Ananthakrishnan R, Mahmood H, Moon TJ, Cunningham CC, Käs J. The optical stretcher: a novel laser tool to micromanipulate cells. Biophys J 2001; 81:767-84; PMID:11463624; http://dx.doi.org/10.1016/S0006-3495(01)75740-2
- Kuznetsova TG, Starodubtseva MN, Yegorenkov NI, Chizhik SA, Zhdanov RI. Atomic force microscopy probing of cell elasticity. Micron 2007; 38:824-33; PMID:17709250; http://dx.doi.org/10.1016/j.micron.2007.06.011
- Grevesse T, Dabiri BE, Parker KK, Gabriele S. Opposite rheological properties of neuronal microcompartments predict axonal vulnerability in brain injury. Sci Rep 2015; 5:9475; PMID:25820512; http://dx.doi.org/10.1038/srep09475
- Desprat N, Richert A, Simeon J, Asnacios A. Creep function of a single living cell. Biophys J 2005; 88:2224-33; PMID:15596508; http://dx.doi.org/10.1529/biophysj.104.050278
- Gabriele S, Benoliel A-M, Bongrand P, Théodoly O. Microfluidic investigation reveals distinct roles for actin cytoskeleton and myosin II activity in capillary leukocyte trafficking. Biophys J 2009; 96:4308-18; PMID:19450501; http://dx.doi.org/10.1016/j.bpj.2009.02.037
- Lenormand G, Hénon S, Richert A, Simeon J, Gallet F. Direct measurement of the area expansion and shear moduli of the human red blood cell membrane skeleton. Biophys J 2001; 81:43-56; PMID:11423393; http://dx.doi.org/10.1016/S0006-3495(01)75678-0
- Yamada S, Wirtz D, Kuo SC. Mechanics of living cells measured by laser tracking microrheology. Biophys J 2000; 78:1736-47; PMID:10733956; http://dx.doi.org/10.1016/S0006-3495(00)76725-7
- Dahl KN, Engler AJ, Pajerowski JD, Discher DE. Power-law rheology of isolated nuclei with deformation mapping of nuclear substructures. Biophys J 2005; 89:2855-64; PMID:16055543; http://dx.doi.org/10.1529/biophysj.105.062554
- Neelam S, Chancellor TJ, Li Y, Nickerson JA, Roux KJ, Dickinson RB, Lele TP. Direct force probe reveals the mechanics of nuclear homeostasis in the mammalian cell. Proc Natl Acad Sci USA 2015; 112:5720-5; http://dx.doi.org/10.1073/pnas.1502111112
- Wildt B, Wirtz D, Searson PC. Triggering cell detachment from patterned electrode arrays by programmed subcellular release. Nat Protoc 2010; 5:1273-80; PMID:20595956; http://dx.doi.org/10.1038/nprot.2010.42
- Soumya SS, Sthanam LK, Padinhateeri R, Inamdar MM, Sen S. Probing Cellular Mechanoadaptation Using cell-substrate de-adhesion dynamics: experiments and model. PLoS ONE 2014; 9:e106915-10; PMID:25197799; http://dx.doi.org/10.1371/journal.pone.0106915
- Rape AD, Guo W-H, Wang Y-L. The regulation of traction force in relation to cell shape and focal adhesions. Biomaterials 2011; 32:2043-51; PMID:21163521; http://dx.doi.org/10.1016/j.biomaterials.2010.11.044
- Versaevel M, Grevesse T, Gabriele S. Spatial coordination between cell and nuclear shape within micropatterned endothelial cells. Nat Commun 2012; 3:671; PMID:22334074; http://dx.doi.org/10.1038/ncomms1668
- Makhija E, Jokhun DS, Shivashankar GV. Nuclear deformability and telomere dynamics are regulated by cell geometric constraints. Proc Natl Acad Sci USA 2016; 113:E32-E40; http://dx.doi.org/10.1073/pnas.1513189113
- Nisenholz N, Rajendran K, Dang Q, Chen H, Kemkemer R, Krishnan R, Zemel A. Active mechanics and dynamics of cell spreading on elastic substrates. Soft Matter 2014; 10:7234-17; PMID:25103537; http://dx.doi.org/10.1039/C4SM00780H
- Yarmola EG, Somasundaram T, Boring TA, Spector I, Bubb MR. Actin-latrunculin A structure and function: differential modulation of actin-binding protein function by latrunculin A. J Biol Chem 2000; 275:28120-7; PMID:10859320.
- Ishizaki T, Uehata M, Tamechika I, Keel J, Nonomura K, Maekawa M, Narumiya S. Pharmacological properties of Y-27632, a specific inhibitor of Rho-associated kinases. Mol Pharmacol 2000; 57:976-83; PMID:10779382.
- Ishizaki T, Naito M, Fujisawa K, Maekawa M, Watanabe N, Saito Y, Narumiya S. p160ROCK, a Rho-associated coiled-coil forming protein kinase, works downstream of Rho and induces focal adhesions. FEBS Lett 1997; 404:118-24; PMID:9119047; http://dx.doi.org/10.1016/S0014-5793(97)00107-5
- Kimura K, Ito M, Amano M, Chihara K, Fukata Y, Nakafuku M, Yamamori B, Feng J, Nakano T, Okawa K, et al. Regulation of myosin phosphatase by Rho and Rho-associated kinase (Rho-Kinase). Science 1996; 273:245-8; PMID:8662509; http://dx.doi.org/10.1126/science.273.5272.245
- Grevesse T, Versaevel M, Circelli G, Desprez S, Gabriele S. A simple route to functionalize polyacrylamide hydrogels for the independent tuning of mechanotransduction cues. Lab Chip 2013; 13:777-1; PMID:23334710; http://dx.doi.org/10.1039/c2lc41168g
- Solon J, Levental I, Sengupta K, Georges PC, Janmey PA. Fibroblast adaptation and stiffness matching to soft elastic substrates. Biophys J 2007; 93:4453-61; PMID:18045965; http://dx.doi.org/10.1529/biophysj.106.101386
- Tee S-Y, Fu J, Chen CS, Janmey PA. Cell shape and substrate rigidity both regulate cell stiffness. Biophys J 2011; 100:L25-7; PMID:21354386; http://dx.doi.org/10.1016/j.bpj.2010.12.3744
- Versaevel M, Riaz M, Grevesse T, Gabriele S. Cell confinement: putting the squeeze on the nucleus. Soft Matter 2013; 9:6665-12; http://dx.doi.org/10.1039/c3sm00147d
- Dahl KN, Scaffidi P, Islam MF, Yodh AG, Wilson KL, Misteli T. Distinct structural and mechanical properties of the nuclear lamina in Hutchinson–Gilford progeria syndrome. Proc Natl Acad Sci USA 2006; 103:10271-6; http://dx.doi.org/10.1073/pnas.0601058103
- Swift J, Discher DE. The nuclear lamina is mechano-responsive to ECM elasticity in mature tissue. J Cell Sci 2014; 127:3005-15; PMID:24963133; http://dx.doi.org/10.1242/jcs.149203
- Davidson PM, Lammerding J. Broken nuclei – lamins, nuclear mechanics, and disease. Trends in Cell Biol. 2014; 24:247-56; http://dx.doi.org/10.1016/j.tcb.2013.11.004
- De Vos WH, Houben F, Hoebe RA, Hennekam R, van Engelen B, Manders EMM, Ramaekers FCS, Broers JLV, Van Oostveldt P. Increased plasticity of the nuclear envelope and hypermobility of telomeres due to the loss of A–type lamins. BBA - General Subjects 2010; 1800:448-58; PMID:20079404; http://dx.doi.org/10.1016/j.bbagen.2010.01.002
- Verstraeten VLRM, Ji JY, Cummings KS, Lee RT, Lammerding J. Increased mechanosensitivity and nuclear stiffness in Hutchinson–Gilford progeria cells: effects of farnesyltransferase inhibitors. Aging Cell 2008; 7:383-93; PMID:18331619; http://dx.doi.org/10.1111/j.1474-9726.2008.00382.x
- Clarke SG. HIV protease inhibitors and nuclear lamin processing: Getting the right bells and whistles. Proc Natl Acad Sci USA 2007; 104:13857-8; http://dx.doi.org/10.1073/pnas.0706529104
- Kamgoué A, Ohayon J, Tracqui P. Estimation of cell young's modulus of adherent cells probed by optical and magnetic tweezers: influence of cell thickness and bead immersion. J Biomech Eng 2007; 129:523-8; http://dx.doi.org/10.1115/1.2746374
- Versaevel M, Braquenier JB, Riaz M, Grevesse T, Lantoine J, Gabriele S. Super-resolution microscopy reveals LINC complex recruitment at nuclear indentation sites. Sci Rep 2014; 4:7362; PMID:25482017; http://dx.doi.org/10.1038/srep07362