ABSTRACT
The Drosophila bang-sensitive mutant tko25t, manifesting a global deficiency in oxidative phosphorylation due to a mitochondrial protein synthesis defect, exhibits a pronounced delay in larval development. We previously identified a number of metabolic abnormalities in tko25t larvae, including elevated pyruvate and lactate, and found the larval gut to be a crucial tissue for the regulation of larval growth in the mutant. Here we established that expression of wild-type tko in any of several other tissues of tko25t also partially alleviates developmental delay. The effects appeared to be additive, whilst knockdown of tko in a variety of specific tissues phenocopied tko25t, producing developmental delay and bang-sensitivity. These findings imply the existence of a systemic signal regulating growth in response to mitochondrial dysfunction. Drugs and RNAi-targeted on pyruvate metabolism interacted with tko25t in ways that implicated pyruvate or one of its metabolic derivatives in playing a central role in generating such a signal. RNA-seq revealed that dietary pyruvate-induced changes in transcript representation were mostly non-coherent with those produced by tko25t or high-sugar, consistent with the idea that growth regulation operates primarily at the translational and/or metabolic level.
Introduction
Mitochondrial dysfunction is a common underlying cause or manifestation of human disease [Citation1–Citation3]. Whilst mammalian models such as the mouse have provided insights into the underlying processes, the use of Drosophila to understand mitochondrial pathophysiology has been relatively neglected, despite its versatility and the availability of a wide variety of easily applied genetic tools.
Deficient mitochondrial protein synthesis is frequently associated with mitochondrial diseases [Citation4], and Drosophila provides a valuable model to study the physiological effects of limitations on mitochondrial translation in the context of animal development. The Drosophila tko gene, encoding mitoribosomal protein S12, a core component of the mitoribosomal decoding centre, has been a particular object of study in this regard. The canonical mutant tko25t displays a range of phenotypic features that resemble mitochondrial disease in humans, including developmental delay, impaired sound-responsiveness, bang-sensitivity (paralytic seizures induced by mechanical shock) and antibiotic sensitivity [Citation5]. Other tko25t phenotypes are unique to Drosophila, such as male courtship defect [Citation5]. These phenotypic features reflect an underlying deficiency of mitoribosomes [Citation5] and consequent global deficiency of the enzymatic functions of oxidative phosphorylation (OXPHOS) that depend upon mitochondrial translation products, manifesting in both adults [Citation5] and larvae [Citation6]. All of these phenotypes are reversed by ubiquitous expression of a transgenic copy of the wild-type tko gene, using the UAS/GAL4 system [Citation6]. Since developmental delay occurs during the larval stages [Citation5], this prompts the question as to which of the larval tissues mediates the crucial signalling that regulates growth in response to limitations on mitochondrial protein synthesis, and by what mechanism.
In a follow-up study [Citation7], we obtained some relevant clues as to the underlying mechanism(s) whereby the growth rate of tko25t larvae is adjusted, so as to take account of the decreased capacity for processing nutritional resources caused by mitochondrial dysfunction. In particular, we observed that components of the apparatus of cytosolic protein synthesis and secretion were down-regulated in tko25t larvae, both at the transcript level and via a key regulatory step of cytosolic protein synthesis, the ribosomal protein S6 kinase (S6K). tko25t larvae were also found to be unaffected by low levels of the cytosolic protein synthesis inhibitor cycloheximide, which retarded the development of wild-type larvae, also implicating the cytoribosome as a crucial target in larval growth regulation. Many other genes that were downregulated in tko25t at the RNA level encoded secreted proteins of the gut and cuticle, suggesting that growth rate in tko25t could be adjusted to compensate for stress in the protein secretory system caused by disruption of redox homeostasis.
In the same study [Citation7] we determined that the strength of the tko25t phenotype depends upon the culture conditions, specifically the sugar content of the growth medium. When tko25t flies were cultured on high-sugar medium, their growth was further impaired compared with those grown on low-sugar medium, and many of the observed changes in gene expression were more pronounced. The effect of high sugar was accompanied by increases in the level of pyruvate and lactate in the larvae, whilst supplementation of the medium with pyruvate or lactate exacerbated developmental delay. tko25t larvae also manifested low levels of ATP and a greatly decreased NADPH/NADP ratio, both of which were enhanced by high-sugar medium [Citation7].
A key result from the previous study was the observation that expression of wild-type tko specifically in portions of the larval gut, a major secretory tissue, partially alleviated the developmental delay of tko25t [Citation7]. Since the driver used in this experiment did not express at a high level in all regions of the gut, we reasoned that other gut-specific drivers used in combination might provide a more complete rescue of the phenotype. To embark on such a study, we initially implemented what we assumed would be negative controls, directing wild-type tko expression in other regions of the larva with high specificity. However, this produced the unexpected result that partial rescue of developmental delay was conferred by expression in each of the tissues tested (muscle, fat-body, neurons, as well as gut), with some evidence of additive effects. Conversely, tko knockdown in each specific tissue produced a partial developmental delay, accompanied by bang sensitivity, the canonical adult phenotype of tko25t.
These new findings indicate the existence of a systemic and possibly metabolic signal integrating growth across the entire larva, in response to limitations on mitochondrial translational or OXPHOS capacity. Given the previous findings implicating pyruvate and/or lactate as key regulatory metabolites, we studied the effects of drugs and RNAi targeted on pyruvate and its metabolic transactions. The findings are consistent with pyruvate metabolism playing a central role in generating the signal that links growth and mitochondrial function in Drosophila larvae.
Materials and methods
Drosophila strains and culture
Drosophila strains were procured from stock centres, supplied by colleagues or maintained long term in our laboratory. A full list of GAL4 drivers, RNAi lines and other strains used in the study are provided in , and , respectively. Markers carried on standard balancers were used to distinguish experimental from control progeny. Except where stated, flies were cultured on standard high-sugar medium (HS), as detailed in [Citation7]. A variant, ‘zero-sugar’ medium (ZS), containing standard dietary supplements but no added sugars [Citation7], was used where indicated. Note that HS and ZS media are not isocaloric: in an earlier study [Citation7] the extent of developmental delay in tko25t flies was shown to depend only on the sugar content of the medium, not its calorific value. Sodium pyruvate, dichloroacetate or UK5099 (Sigma-Aldrich), were added to these media from aqueous stock solutions after the medium had been cooled to below 65°C, giving the final concentrations indicated in the figures.
Table 1. GAL4 drivers used in the study.
Table 2. RNAi lines used in the study.
Table 3. Other Drosophila strains used in the study.
Developmental and bang-sensitivity assays
Mean developmental time to eclosion and bang-sensitivity were measured as previously [Citation5,Citation7], in temperature-controlled incubators, with temperature verified daily throughout the experiment. In all crosses where developmental time to eclosion was measured, at least 3 (usually 4) replicate vials were studied, and the entire experiment was repeated to validate the findings.
RNA analysis
Quantitative reverse-transcription PCR (qRTPCR) to confirm the effectiveness of RNAi was conducted as previously [Citation7,Citation37], using RpL32 as an internal standard and verified primer pairs (all shown 5´ to 3´) as follows: for Pdk – GGATTCGGAACAGATGCAAT and CGCGATAGAACTTTGAGCTTG, for Mpc1 – GCCGACACACAAAAGAGTCC and GCTGGACCTTGTAGGCAAAT, for Men – ACTCGATCCTACGACGCTGT and TGAGGAAGGACTCTGCGAAT. RNA sequencing and data analysis were carried out as previously [Citation7], using RNA from Oregon R or tko25t L3-stage larvae cultured on different media. Note that initial statistical filtering by Cuffdiff (chipster.csc.fi) excludes genes in any pairwise comparison where the differences fail significance testing, regardless of their magnitude. For further analysis (see Results), arbitrary thresholds were then applied to restrict the analysis to genes showing substantial differences in expression, as measured by either of two parameters: >8 fold change or >100 units of FPKM (mass fraction). Raw sequence data have been deposited at ArrayExpress (www.ebi.ac.uk/ArrayExpress/).
Metabolite analysis
Batches of 20 larvae were homogenized in 100 µl of 6M guanidine hydrochloride on ice. The homogenate was incubated at 95°C for 5 min and centrifuged at 12000gmax for 5 min at 4°C. The supernatants were stored at −80°C, and later diluted 1:10 and with PBS (pH 7.4) for analysis. Pyruvate and lactate were measured with commercially available fluorescence-based determination kits (Abcam) according to manufacturer’s instructions. 10 µl of sample was combined with 50 µl of either lactate or pyruvate reaction mix, incubated at room temperature for 30 min after which fluorescence was measured (excitation at 535 nm, emission at 590 nm) using a plate reader. Lactate and pyruvate standards were used to generate standard curves and concentrations were normalized to soluble protein as measured using the Bradford method.
Statistics
For pairwise comparisons between groups, the two-tailed (unpaired) Student’s t test (Microsoft Excel) was applied. For multiple comparisons, we used one-way ANOVA with Tukey post hoc HSD test online (astatsa.com). For comparisons where multiple factors were being assessed, two-way ANOVA (GraphPad Prism or online tool at vassartstats.net/anova2u.html, as indicated) was used, together with Dunnett’s or Tukey’s post hoc multiple comparisons tests as indicated, where interactions were detected, or where more than two levels were compared.
Results
Wild-type tko expression in diverse tissues alleviates developmental delay in tko25t
Using the line UAS-tko+(8) [Citation6], in which a wild-type tko transgene is expressed under the control of GAL4, we tested the tissue-specificity of developmental delay in the tko25t background, by combining it with different GAL4 drivers directing distinct tissue patterns of expression. In addition to almost complete rescue using da-GAL4 and the partial rescue with gut-GAL4 documented previously, we found that drivers specific for the fat body (Lsp2-GAL4), muscle (G14 and Mef2-GAL4) and CNS (neurons, elav-GAL4) all gave a partial rescue (), although this was most pronounced or significant in each case at different characteristic temperatures (Table S1).
Figure 1. tko25t developmental delay is partially alleviated by tko+ expression directed by different drivers. Time to eclosion (means ± SD, n ≥ 3 replicate vials for each cross), of flies of the indicated genotypes, using UAS-tko+(8) with the indicated drivers. Controls were FM7 balancer flies with (a, b and d – right-hand panel) driver but no transgene, (c), driver or transgene (these classes could not be distinguished due to the nature of the cross, since the G14 driver is not viable as a homozygote), and (d – left-hand panel) neither transgene nor driver, as dictated by the chromosomal location of the drivers. Because the elav-GAL4 driver is located on the X chromosome, one of the two reciprocal crosses used in (d) generates only informative females and not males. Horizontal lines denoted by asterisks (*, **, ***) indicate significant differences in pairwise comparisons of flies of a given sex and tko genotype, with and without actively driven tko+ (Student’s t test, p < 0.05, 0.01, 0.001, respectively. The specificity of each driver was confirmed by parallel crosses in which it was used to direct the synthesis of nuclear- or membrane-localized GFP (see Fig. S1). Note that we avoided the use of the TM3 balancer because we established that it conferred a developmental delay in conjunction with tko25t, whereas the chromosome 2 balancer CyO did not (Fig. S2). The partial rescue of developmental delay was also observed at other temperatures with some drivers (see Table S1) and using the alternate transgene UAS-tko+(1) – see . Note that most GAL4 drivers exhibit the classic pattern of temperature dependence [Citation38], i.e. increased activity at higher temperature. However, for the strongest drivers, this may also lead to deleterious effects of over-expression at high temperature, such that a lower temperature produces optimal effects.
![Figure 1. tko25t developmental delay is partially alleviated by tko+ expression directed by different drivers. Time to eclosion (means ± SD, n ≥ 3 replicate vials for each cross), of flies of the indicated genotypes, using UAS-tko+(8) with the indicated drivers. Controls were FM7 balancer flies with (a, b and d – right-hand panel) driver but no transgene, (c), driver or transgene (these classes could not be distinguished due to the nature of the cross, since the G14 driver is not viable as a homozygote), and (d – left-hand panel) neither transgene nor driver, as dictated by the chromosomal location of the drivers. Because the elav-GAL4 driver is located on the X chromosome, one of the two reciprocal crosses used in (d) generates only informative females and not males. Horizontal lines denoted by asterisks (*, **, ***) indicate significant differences in pairwise comparisons of flies of a given sex and tko genotype, with and without actively driven tko+ (Student’s t test, p < 0.05, 0.01, 0.001, respectively. The specificity of each driver was confirmed by parallel crosses in which it was used to direct the synthesis of nuclear- or membrane-localized GFP (see Fig. S1). Note that we avoided the use of the TM3 balancer because we established that it conferred a developmental delay in conjunction with tko25t, whereas the chromosome 2 balancer CyO did not (Fig. S2). The partial rescue of developmental delay was also observed at other temperatures with some drivers (see Table S1) and using the alternate transgene UAS-tko+(1) – see Figure 2. Note that most GAL4 drivers exhibit the classic pattern of temperature dependence [Citation38], i.e. increased activity at higher temperature. However, for the strongest drivers, this may also lead to deleterious effects of over-expression at high temperature, such that a lower temperature produces optimal effects.](/cms/asset/6efc666f-8f41-40ca-9071-d58262808c02/kfly_a_1662266_f0001_c.jpg)
Figure 2. Alleviation of tko25t developmental delay in a second UAS-tko+ line. Time to eclosion at 25°C (means ± SD, n ≥ 3 replicate vials for each cross), of flies of the indicated genotypes, using various drivers plus the UAS-tko+(1) transgene, shown previously to confer a modest rescue of developmental delay without any driver [Citation6]. Controls were FM7 balancer flies with transgene but without driver, as shown. Horizontal lines denoted by asterisks (*, **, ***) indicated significant differences in pairwise comparisons of flies of a given sex and tko genotype, with and without actively driven tko+(Student’s t test, p < 0.05, 0.01, 0.001, respectively. The specificity of each driver was confirmed by parallel crosses in which it was used to direct the synthesis of nuclear- or membrane-localized GFP (see Figure S1). Note that we avoided the use of the TM3 balancer because we established that it conferred a developmental delay in conjunction with tko25t, whereas the chromosome 2 balancer CyO did not (Fig. S2).
![Figure 2. Alleviation of tko25t developmental delay in a second UAS-tko+ line. Time to eclosion at 25°C (means ± SD, n ≥ 3 replicate vials for each cross), of flies of the indicated genotypes, using various drivers plus the UAS-tko+(1) transgene, shown previously to confer a modest rescue of developmental delay without any driver [Citation6]. Controls were FM7 balancer flies with transgene but without driver, as shown. Horizontal lines denoted by asterisks (*, **, ***) indicated significant differences in pairwise comparisons of flies of a given sex and tko genotype, with and without actively driven tko+(Student’s t test, p < 0.05, 0.01, 0.001, respectively. The specificity of each driver was confirmed by parallel crosses in which it was used to direct the synthesis of nuclear- or membrane-localized GFP (see Figure S1). Note that we avoided the use of the TM3 balancer because we established that it conferred a developmental delay in conjunction with tko25t, whereas the chromosome 2 balancer CyO did not (Fig. S2).](/cms/asset/32985f41-320e-4413-a56e-db994aa3bc6f/kfly_a_1662266_f0002_c.jpg)
We confirmed the specificity of patterns of expression of these drivers using GAL4-dependent constructs for GFP (Fig. S1). The alleviation of the phenotype was due to a positive effect of the transgene/driver combination, and not due to a negative effect of the CyO balancer chromosome in the tko25t background (Fig. S2). Note, however, that this was not true of the TM3Sb balancer (Fig. S2), the use of which was therefore avoided in all experiments described.
The partial rescue produced by several drivers appeared to be additive, based on two lines of evidence. First, we attempted to combine pairs of drivers and the UAS-tko+(8) transgene. This experiment was technically challenging for several reasons: the problematic nature of the TM3Sb balancer precluded its use; some transgenic combinations had poor viability, especially as homozygotes, and the drivers did not all perform optimally at the same temperature. However, the combination of the fat-body and muscle drivers Lsp2-GAL4 and G14 appeared to give an additive enhancement at 22°C (Fig. S3), although this should be interpreted cautiously, due to the imperfect experimental design. The second piece of evidence for a combinatorial effect was that a second transgenic line, UAS-tko+(1), which already showed a one-day alleviation of developmental delay compared with tko25t flies bearing no transgene [Citation6], showed a further alleviation of developmental delay when combined with different GAL4 drivers (). These findings suggest the operation of a systemic signal that integrates the degree of mitochondrial dysfunction across tissues, calibrating growth to the ability of the organism to process nutritional resources.
RNAi-mediated tko knockdown in diverse tissues phenocopies tko25t
To further test this hypothesis, we used RNA-mediated knockdown of tko to profile the tissues in which the resulting mitochondrial translational deficit leads to a tko25t-like phenotype. Ubiquitous tko knockdown using the da-GAL4 driver and the Bloomington TRiP line 38251 targeted on tko resulted in a phenotype resembling an exaggerated version of tko25t. At 25°C tko knockdown was lethal, whilst at 22°C, it was lethal to males and semilethal to females, which eclosed with a long delay (5–9 d) and were too weak to permit a meaningful test of their bang-sensitivity. At 18°C females eclosed with a 7 to 8-d delay ()) and were highly bang-sensitive ()), whilst the few males that eclosed were even more delayed ()) and extremely weak. Knockdown using tissue-specific drivers produced a milder version of the same phenotype, whether knockdown was targeted specifically to neurons (using elav-GAL4, ), )) or muscle (Mef2-GAL4 at 18°C, , ). Even more limited knockdown targeted on the early embryo, and portions of the midgut (Kr-GAL4, ), Fig. S1) also produced a significant, though very modest developmental delay, but without bang-sensitivity ()). Mef2-GAL4-driven tko knockdown at higher temperatures (22, 25°C) again gave semilethality and severe weakness, which was more severe in males. elav-GAL4-driven knockdown also gave sex- and temperature-dependent bang-sensitivity: at 25°C flies were extremely weak, whilst developmental delay was seen at all temperatures but was generally significant only in males ()). Driving tko knockdown with the glial driver nrv2-GAL4 gave no bang sensitivity ()).
Figure 3. RNAi knockdown of tko by different drivers results in developmental delay. Times to eclosion (means ± SD, n ≥ 3 replicate vials for each cross) for flies of the indicated sex and genotype, using the various drivers at the temperatures shown. Horizontal lines denoted by asterisks (*, **, ***) indicate significant differences in pairwise comparisons between knockdown and control flies of a given sex, using a given driver (Student’s t test, p < 0.05, 0.01, 0.001, respectively). Note that males were in general more severely affected and in some cases (e.g. da-GAL4) too few males eclosed to permit a statistically meaningful analysis. Note that most GAL4 drivers exhibit the classic pattern of temperature dependence [Citation38], i.e. increased activity at higher temperature. However, for the strongest drivers this may also lead to highly deleterious effects at high temperature, such that, at a lower temperature, results are more informative.
![Figure 3. RNAi knockdown of tko by different drivers results in developmental delay. Times to eclosion (means ± SD, n ≥ 3 replicate vials for each cross) for flies of the indicated sex and genotype, using the various drivers at the temperatures shown. Horizontal lines denoted by asterisks (*, **, ***) indicate significant differences in pairwise comparisons between knockdown and control flies of a given sex, using a given driver (Student’s t test, p < 0.05, 0.01, 0.001, respectively). Note that males were in general more severely affected and in some cases (e.g. da-GAL4) too few males eclosed to permit a statistically meaningful analysis. Note that most GAL4 drivers exhibit the classic pattern of temperature dependence [Citation38], i.e. increased activity at higher temperature. However, for the strongest drivers this may also lead to highly deleterious effects at high temperature, such that, at a lower temperature, results are more informative.](/cms/asset/64e20078-e9ee-4581-a176-066848f34899/kfly_a_1662266_f0003_c.jpg)
Figure 4. RNAi knockdown of tko by different drivers results in bang-sensitivity. Box-plots of recovery times from mechanical shock (bold black or red lines – medians, respectively, for controls and tko knockdown flies, with filled boxes representing first to third quartiles) of flies of the indicated genotype, sex and culture temperature. Note the different scales required to plot these data. White bars for control flies were in most cases not plottable, since median and both quartiles were at or very close to zero.
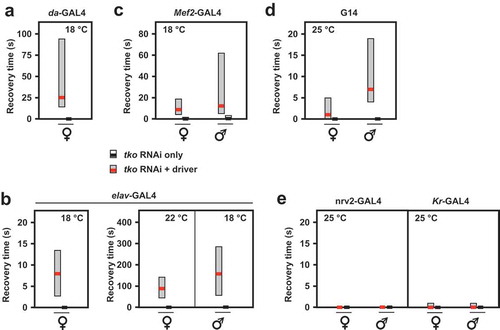
Drugs that affect pyruvate metabolism impact larval growth
In principle, a systemic signal regulating growth according to mitochondrial function could be endocrine or metabolic in nature. Candidate metabolites for such a role that were previously shown to be markedly abnormal in tko25t larvae, include pyruvate and lactate, which were approximately threefold elevated, and NADPH and ATP, which were highly depleted [Citation7]. Since ATP and NADPH may be considered too labile to perform an intercellular role, we focused our attention on pyruvate and lactate, which are interconvertible through lactate dehydrogenase, and which were previously found to exacerbate and phenocopy the developmental delay of tko25t, when added to the medium ([Citation7], )). We investigated the developmental effect of two drugs known to affect pyruvate metabolism, dichloroacetate (DCA), an inhibitor of pyruvate dehydrogenase kinase (Pdk) and UK5099, an inhibitor of the mitochondrial pyruvate carrier, which could be predicted to have opposite effects on mitochondrial pyruvate utilization. We tested different concentrations of pyruvate and DCA for their effects on the development of wild-type and tko25t females in ZS medium ()), and performed a more extensive study at single, effective concentrations on different media and both sexes, including heterozygous tko25t females ()). Like pyruvate, DCA produced an additional, dose-dependent developmental delay in both tko25t as well as in wild-type flies (, , S5)).
Figure 5. Drugs affecting pyruvate metabolism modify the tko25t phenotype. Means ± SD of times to eclosion of flies of the indicated genotypes and sex, at 25°C, on different media: HS – standard high-sugar medium, ZS – zero sugar medium, with addition of the indicated drugs that were present throughout the experiment: pyr – pyruvate, 25 mg/ml in (a) and concentrations as indicated in (b), DCA – dichloroacetate, 12.5 mg/ml in (a) and concentrations as indicated in (b), UK5099 – 25 μg/ml. For statistical analysis of data from (a) by two-way ANOVA, see Figure S5. In summary, this revealed a significant effect of both genotype and drug addition (p < 0.001) for both diets and sexes, as well as an interaction between genotype and drug addition for both sexes on HS diet (p < 0.01) and for females only, on ZS diet (p < 0.001). Tukey post hoc HSD test (p < 0.05) was used, where appropriate, to determine the source of variation (see Figure S5 and comments in main text). Note that some of the data from (a) was previously published in [Citation7], but without this statistical analysis, but is included here for full comparison. For a summary diagram of the reactions targeted by these drugs and by RNAi (see .
![Figure 5. Drugs affecting pyruvate metabolism modify the tko25t phenotype. Means ± SD of times to eclosion of flies of the indicated genotypes and sex, at 25°C, on different media: HS – standard high-sugar medium, ZS – zero sugar medium, with addition of the indicated drugs that were present throughout the experiment: pyr – pyruvate, 25 mg/ml in (a) and concentrations as indicated in (b), DCA – dichloroacetate, 12.5 mg/ml in (a) and concentrations as indicated in (b), UK5099 – 25 μg/ml. For statistical analysis of data from (a) by two-way ANOVA, see Figure S5. In summary, this revealed a significant effect of both genotype and drug addition (p < 0.001) for both diets and sexes, as well as an interaction between genotype and drug addition for both sexes on HS diet (p < 0.01) and for females only, on ZS diet (p < 0.001). Tukey post hoc HSD test (p < 0.05) was used, where appropriate, to determine the source of variation (see Figure S5 and comments in main text). Note that some of the data from (a) was previously published in [Citation7], but without this statistical analysis, but is included here for full comparison. For a summary diagram of the reactions targeted by these drugs and by RNAi (see Figure 10).](/cms/asset/380d964f-2fb1-43b4-beb6-122203fbebb8/kfly_a_1662266_f0005_c.jpg)
UK5099 (25 μg/ml) also exacerbated the developmental delay of tko25t, but had no significant effect on the eclosion timing of wild-type flies (, S5)).
Genetic manipulations that affect pyruvate metabolism impact larval growth and survival
Next, we analyzed the effects of knocking down the genes coding for the key proteins of pyruvate metabolism targeted by these drugs. The mitochondrial pyruvate carrier is a heterodimer of the ubiquitous subunit Mpc1 (CG14290) and a differentially expressed second subunit, Mpc2, encoded in Drosophila by a small gene family (CG9396, CG9399 and CG32832, the latter being testis specific). Pdk is encoded by a single-copy gene (Pdk, CG8808). Using the available RNAi lines for Mpc1 and Pdk from the Harvard Medical School TRiP library, we first confirmed that knockdown for each of the two genes using the ubiquitously acting daGAL4 driver gave viable flies, and used qRTPCR to verify that knockdown was effective at the RNA level ()). We then evaluated the effects of knockdown on wild-type and tko25t flies gown in standard high-sugar medium, or on medium supplemented with 25 mg/ml pyruvate ()). Mpc1 or Pdk knockdown produced no effect on eclosion timing in wild-type flies cultured on standard medium. However, when pyruvate was added to the medium, Mpc1 knockdown exacerbated the developmental delay produced in wild-type flies, but not that of tko25t (), panel i), whilst Pdk knockdown had no effect on eclosion timing of wild-type flies on either medium, but mildly alleviated the developmental delay of tko25t (), panel ii) on pyruvate-supplemented medium (see Fig. S6 for summary of the relevant statistical analyses by two-way ANOVA).
Figure 6. RNAi-mediated knockdown of Mpc1 or Pdk modifies the tko25t phenotype. (A) Mpc1 or Pdk RNA levels measured by qRTPCR and (B) time to eclosion of flies of the indicated genotypes (i.e. with relevant RNAi construct or balancer as shown), at 25°C, means ± SD, n ≥ 3 vials from each cross on the indicated medium: HS – high-sugar medium, with or without the addition of 25 mg/ml pyruvate. Horizontal dotted lines represent the mean eclosion times of Oregon R females and tko25t females and males on HS medium, to facilitate comparisons. Statistical analysis by two-way ANOVA (Fig. S6) revealed a significant effect of genotype under all conditions, but both Mpc1 and Pdk knockdown produced significant effects and showed significant interaction with genotype only in HS medium supplemented with pyruvate; Mpc1 knockdown retarding the development only of wild-type and Pdk knockdown retarding only that of tko25t flies of both sexes. Note that, in panel ii of (B), the CyO balancer flies from panel i, generated in the same experiment, are presented as the best indicative control. The TM3 balancer chromosome, whether marked with Sb or Ser, confers a developmental delay of >1 d that precludes its use as a control in such experiments, other than to allow identification of the non-balancer RNAi-bearing flies. Apart from this, all comparisons between control and knockdown flies of a given sex and genotype were from the same experiment, although Oregon R and tko25t flies were analyzed from separate crosses, as were flies cultured on different media. For a summary diagram of the reactions targeted by RNAi and by various drugs see .
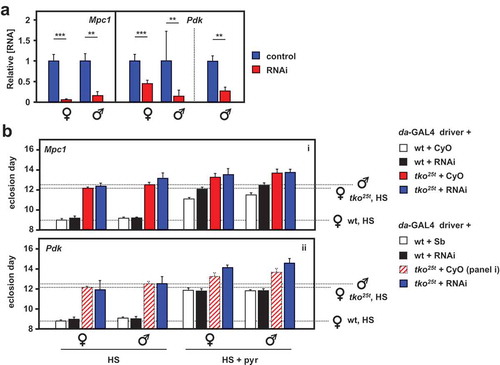
Knockdown of two other genes of pyruvate metabolism, coding, respectively, for the cytosolic and mitochondrial isoforms of malic enzyme, produced more dramatic results in combination with tko25t. In our previous study, we observed that knockdown of Men, the gene encoding the cytosolic isoenzyme, produced no significant effects on tko25t () of [Citation7]). In the present study we were able to make use of more potent and specific TRiP lines for Men, as well as one further dsRNA line from the VDRC collection, all of which gave a strong knockdown of the gene at the RNA level under the control of da-GAL4 ()), but had no significant effect on the development of otherwise wild-type flies ()). However, in combination with tko25t they were all developmentally lethal or semilethal at both 29°C and 25°C ()). Both of the Men-b knockdown lines produced a developmental delay in wild-type flies, and were again synthetically lethal with tko25t (Fig. S4), but this result should be interpreted cautiously, since we were not able to demonstrate convincing and consistent knockdown of Men-b at the RNA level by qRTPCR, using several different primer sets. Knockdown of lactate dehydrogenase (Ldh) was lethal to both wild-type and tko25t larvae, making comparable experiments uninformative.
Figure 7. RNAi-mediated knockdown of Men (malic enzyme is synthetically (semi)lethal with tko25t. (a) Men RNA levels measured by qRTPCR and (b) time to eclosion of flies of the indicated genotypes (i.e. with relevant Men RNAi construct or balancer as shown), means ± SD, n ≥ 3 vials from each cross. Eclosion timing is shown for the temperature at which flies were also cultured for qRTPCR (25°C or 29°C as shown). Eclosion timing at the other temperature was qualitatively similar, as shown in Fig. S4A. Horizontal bars denoted by asterisks (**) indicate significant differences, in pairwise comparisons of RNAi and balancer control flies of each given genotype and sex analyzed (Student’s t test, p < 0.001). (c) Proportion (%) of eclosing RNAi male progeny that also carried tko25t, as opposed to the FM7 balancer, from crosses of the general type: tko25t/FM7; daGAL4 x FM7/Y; RNAi. Means ± SD, n ≥ 3 vials from each cross. Asterisks (***) above the bars denotes significant deviation from expected frequency of 50% (chi-squared test with Yates’ continuity correction, p < 0.001). See also Fig. S4. For a summary diagram of the reactions targeted by RNAi and by various drugs see .
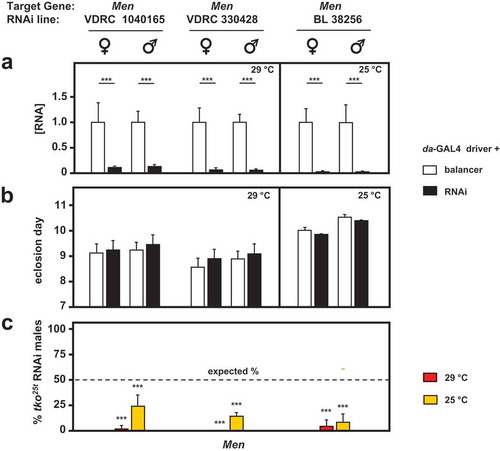
Despite their similar effects on eclosion timing (), the addition of pyruvate and DCA to the low-sugar culture medium had opposite effects on the tissue levels of pyruvate and lactate in L3 larvae (), although the changes were only significant for pyruvate levels (Tables S3). Pyruvate addition increased both lactate and pyruvate to levels comparable with those seen in high-sugar medium, whilst DCA lowered them, in accord with the expectation that it would augment pyruvate metabolism by removing inhibition from (i.e. activating) PDH. The different effects of pyruvate and DCA imply that growth rate cannot be determined directly or solely by pyruvate concentration averaged across the tissues. Instead, a metabolite of pyruvate, or pyruvate in a specific intracellular or tissue-compartment, is likely to be instrumental.
Figure 8. DCA modifies pyruvate and lactate levels oppositely from the effect of pyruvate addition. (a) Pyruvate and (b) lactate levels (means ± SD, n ≥ 3, generally four batches for each group) in L3 larvae of the indicated genotypes (OR = Oregon R wild-type). HS – high-sugar medium, ZS – zero-sugar medium, DCA – with addition of 12.5 mg/ml DCA, pyr, lac – with addition of 25 mg/ml pyruvate or lactate, respectively. Data are normalized to the values for wild-type on ZS medium. For statistical analysis, see Tables S3. For a summary diagram of the reactions targeted by drugs and RNAi see .
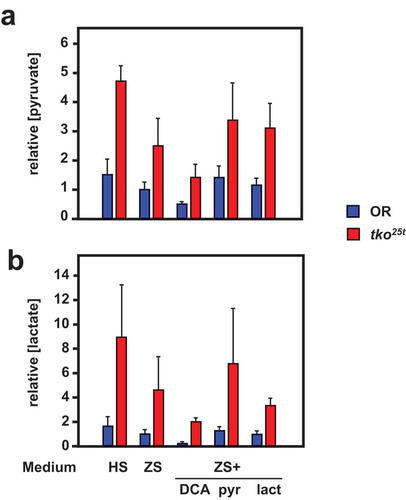
Gene expression changes induced by pyruvate are non-coherent with those induced by tko25t
Growth of tko25t in high sugar was previously observed to downregulate some components of the protein synthetic and secretory machinery, as well as a number of key genes involved in developmental progression. These effects were seen also seen in zero-sugar medium but were less pronounced. We used RNA-seq to test whether pyruvate addition produced the same changes in gene expression, applying similar criteria as in the previous study [Citation7]. Using each of two parameters (i) absolute magnitude of changes measured by mass fraction (FPKM), and (ii) fold-change, we created lists of the genes showing the most pronounced alterations in expression due to pyruvate supplementation (Table S4). We considered both protein-coding and non-coding RNAs, as well as increases and decreases. From these ‘base-comparison’ lists of pyruvate-regulated genes (198 in the mass-fraction list and 248 in the fold-change list, applying arbitrary thresholds of >100 FPKM units or >eightfold change, Tables S4) we asked how many are regulated similarly in each of six additional comparisons (). In each output we considered the genes from the base-comparison list in five categories: those regulated in the same direction, either (i) above or (ii) below the arbitrary thresholds, those regulated in the opposite direction, again (iii) above or (iv) below threshold, and (v) those missing from the compared list, due to the initial statistical filtering. This analysis enabled us to draw the following conclusions, illustrated in . First, most of the genes up- or down-regulated by pyruvate in wild-type larvae were regulated similarly by pyruvate in tko25t (comparison 1 in ). Second, only a minor subset of genes (≤15%) were regulated in the same direction by high-sugar (comparisons 2 and 3). Many genes were oppositely regulated by the presence of tko25t (comparisons 4 and 5), and many pyruvate-regulated genes in wild-type showed either no effect or a change in the opposite direction in tko25t larvae (comparison 6). The two approaches (mass-fraction and fold-change) gave similar outcomes. The functionally identified genes regulated similarly by pyruvate and by sugar were mostly linked to development (cuticular, muscle and a few gut proteins: Tables S5, sheet 1). Many of them, or others in the same functional categories, were oppositely altered in tko25t (Tables S5, sheet 2). Many of the remaining genes regulated by dietary pyruvate, that were not affected by sugar or by tko25t, code for components of the gene expression machinery or for OXPHOS and other mitochondrial functions (Table S4).
Figure 9. Gene expression changes induced by pyruvate are mostly different from those induced by high-sugar or tko25t. Plotted comparisons are based on analysis by (a) FPKM (mass-fraction) and (b) fold-change, with arbitrary threshold of 100 FPKM units or eightfold change, respectively. The plot ignores the actual direction of change and the nature of the regulated transcripts (protein-coding or non-coding RNA) but instead shows whether the direction of change is the same or different between the base-comparison (genes regulated by pyruvate addition to zero-sugar medium) and the comparisons as enumerated. In each case, the changes to the remaining genes from the base-comparison set did not pass statistical filtering in the other comparisons.
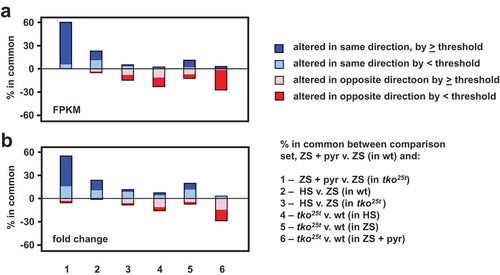
Discussion
Developmental delay in tko25t depends on a systemic signal
Three lines of evidence presented in this paper support the idea that a systemic signal links mitochondrial function to growth rate in Drosophila development. First, the developmental delay of tko25t mutant larvae could be partially corrected () by expression of the wild-type allele via any of several different tissue-specific drivers, showing little or no overlap in the specificity of their expression (Fig. S1). Second, as far as could be demonstrated, these effects were additive (, S4). Third, RNAi-mediated knockdown of tko using similarly specific drivers regenerated the mutant phenotype of tko25t, including both developmental delay as well as (at least in some cases) bang-sensitivity. These observations imply that diminished mitochondrial function in a specific tissue is somehow integrated across the entire larva, presumably by the sharing of metabolites and their processing. Furthermore, this is not an all-or-none phenomenon, where growth is switched between two alternate programs whenever mitochondrial function crosses some threshold: the amount of developmental delay is variable, implying that the overall metabolic capacity of the larva is somehow being measured physiologically, and growth rate adjusted accordingly. These findings could be further strengthened by the use of additional drivers, especially in combination, although the problematic nature of the TM3 balancer means that we would need first to identify those carried on chromosome 3 that were viable as homozygotes (also with no maternal effects) and which had no unreported off-target expression patterns.
As indicated above, we hypothesized that such a signal could be either endocrine or metabolic in nature, or a combination of both. Endocrine signals of metabolic – specifically mitochondrial stress are well established in other organisms, such as FGF21 or GDF15 in mammals [Citation39]. Although both of these growth-factor superfamilies have representatives in insects, neither has a clear orthologue in Drosophila. The closest match to human GDF15, Glass bottom boat, is much more similar to other members of the BMP/TGFβ superfamily, whilst none of the three Drosophila FGFs (Pyramus, Thisbe and Branchless) is a convincing match to human FGF21, and each of them plays a well-studied role in specific developmental programs (see [Citation40] for review).
Two other well-known endocrine systems in Drosophila do provide a more direct connection between growth regulation and mitochondrial metabolism, namely the insulin-like peptides and the steroid hormone ecdysone. The insulin/IGF signalling system in the fly [Citation41,Citation42] is responsive to metabolic and nutritional signals to co-ordinate growth and behaviour, whilst ecdysteroids, partly synthesized in mitochondria [Citation43,Citation44], regulate progression between the different developmental stages, especially the onset of metamorphosis [Citation45]. It will be worthwhile to study whether either interacts with tko25t.
Tissue-specificity of the bang-sensitive phenotype
Bang-sensitivity is reported as a feature of mutants in two other genes for core functions of mitochondria, namely sesB (adenine nucleotide translocase) [Citation46] and kdn (citrate synthase) [Citation47]. These mutants, as well as tko25t, show ATP depletion [Citation47–Citation49]. Global tko knockdown was here shown to phenocopy both the developmental delay () and bang-sensitivity () of the original tko25t mutant, supporting the view that tko25t is a simple hypomorph. However, the two phenotypes are clearly separable, based on the fact that the transgenic line UAS-tko+(8) shows no rescue of developmental delay in the absence of a GAL4 driver, but does exhibit effective rescue of bang-sensitivity [Citation6], due, presumably, to an insertional effect [Citation50]. The use of tissue-specific drivers to knock down tko sheds further light on this, since exclusively neuronal or muscle drivers both gave a bang-sensitive phenotype (–)), whereas a glial driver or one active in embryogenesis and in parts of the larval midgut (Fig. S1A) did not (,)). These findings imply that adequate mitochondrial OXPHOS capacity in muscle and in neurons, but not in other tissues, is required to avoid the prolonged paralytic seizures characteristic of tko25t. The severity of the bang-sensitive phenotype produced by knockdown of tko using different GAL4 drivers is comparable with, or in some cases stronger, than that produced by tko25t. However, since the relative strength of the various tissue-specific drivers is not easily measured, the significance of this cannot be assessed.
Lactate and pyruvate metabolism is linked to growth regulation in tko25t
Candidates for a metabolic signal of mitochondrial dysfunction were already suggested by the previous observation of elevated pyruvate and lactate levels in tko25t larvae [Citation7], and the fact that their addition to the culture medium phenocopied the effects of high-sugar on tko25t. Serum lactate is already considered a key biomarker for diagnosing OXPHOS disorders in humans [Citation39], whilst pyruvate has been identified as a crucial regulator of stemness and growth in mammalian cells [Citation51]. In flies, the enzyme that interconverts lactate and pyruvate, lactate dehydrogenase (Ldh, or ImpL3) has previously been implicated as a target of growth signalling by the estrogen-related receptor [Citation52]. This offered an attractive starting point for the present study.
Drugs and genetic manipulations that affect pyruvate metabolism (summarized in ) impacted the development of tko25t (and, in some cases, wild-type flies), broadly supporting the view that pyruvate accumulation is a marker for a metabolic process that limits growth. The growth-inhibiting effects of pyruvate and of DCA were also dose-dependent. However, measurements of lactate and pyruvate levels in flies grown on different media () implied that neither was a direct predictor of developmental phenotype, which may instead depend on a downstream product of pyruvate metabolism. Thus, pyruvate or lactate supplementation resulted in only a modest increase in the steady-state level of pyruvate, whereas DCA, which substantially decreased pyruvate and lactate levels (), produced paradoxical effects on developmental timing, accelerating the growth of tko25t on zero-sugar medium, but retarding that of wild-type flies, whilst in high-sugar medium it compounded rather than alleviated tko25t developmental delay ()). Pdk knockdown exacerbated the developmental delay of tko25t (), ii), but only under conditions of pyruvate overload. Although DCA is a potent inhibitor of Pdk [Citation53], it may also have other targets [Citation54–Citation56] and is accumulated in cells via the plasma-membrane monocarboxylate transporters, MCTs [Citation57]. Moreover, in cancer cells, it has been reported to inhibit the pentose phosphate pathway [Citation58] and thereby decrease the level of NADPH, which is already depleted in tko25t, especially when grown on high-sugar medium [Citation7]. Such off-target effects may account for the different outcomes of DCA treatment and Pdk knockdown.
Figure 10. Summary diagram of steps in the pyruvate metabolic network targeted by drugs and RNAi. Metabolites are shown in black text, proteins in red, and drugs in white on black. Mpc1, Mpc2, Pdk, Men, Men-b and Ldh are shown by the systematic names from flybase. PDC – pyruvate dehydrogenase complex. For clarity, enzymes not targeted in the present experiments (such as pyruvate kinase and pyruvate carboxylase) are omitted.
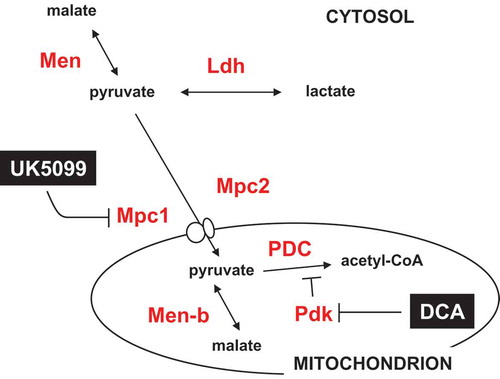
UK5099, predicted to limit mitochondrial pyruvate utilization by inhibiting the mitochondrial pyruvate carrier, produced a clear exacerbation of the tko25t developmental phenotype ()), whist Mpc1 knockdown produced different effects, slowing the growth of wild-type flies under pyruvate overload, but exhibiting only minor effects on tko25t development that were essentially epistatic to the effect of added pyruvate (), i). Although originally identified as an inhibitor of the mitochondrial pyruvate carrier [Citation59], UK5099 was subsequently shown to block MCTs as well [Citation60–Citation63]. If it blocks lactate efflux in vivo, its net effect may be to increase cytosolic lactate and/or pyruvate independently of its effects on mitochondria, potentially accounting for the different outcome of Mpc1 knockdown. Other, more specific inhibitors, such as PS10 for Pdk [Citation64], or GW604714X and GW450863X for the mitochondrial pyruvate carrier [Citation65], may prove useful in disentangling these effects, although their efficacy and specificity would need to be verified for Drosophila.
The findings with Men are also consistent with pyruvate being a critical metabolite, although the effect on tko25t was lethality rather than extended development. One possibility is that a high level of pyruvate facilitates NADPH depletion via the NADPH-dependent conversion of pyruvate to malate catalyzed by Men. If, as hypothesized earlier, NADPH depletion is a critical element of growth signalling in tko25t [Citation7], a decreased capacity of pyruvate to malate conversion could undermine this signalling, with catastrophic consequences for a larva with severe limitations on TCA-cycle and biosynthetic flux, and ATP depletion.
Overall, whilst we can infer that manipulations affecting pyruvate and lactate metabolism affect growth-regulation in tko25t, possible off-target or secondary effects of the drugs and knockdowns preclude a definitive mechanistic conclusion at this time. Unravelling the many possibilities will require the development of methods for assaying fluxes of the key metabolites at the subcellular level in vivo, considering separately the mitochondrial and cytosolic pools of pyruvate, malate, NADPH, ATP and their derivatives.
Pyruvate modulates growth rate translationally and/or post-translationally
Growth of tko25t in high-sugar medium was previously observed to affect gene expression in two ways: first, via a post-translational mechanism affecting S6K, second, via changes in transcript representation [Citation7]. In the present study, we found that only a minor fraction of the genes regulated at the RNA level by pyruvate were regulated in the same way by high sugar, whilst those responding to tko25t were mostly altered in the opposite direction. This was unexpected, given the fact that tko25t responded similarly to pyruvate and high sugar, and suggests that global growth regulation in response to mitochondrial dysfunction and metabolic disturbance occurs mainly at the (post-)translational level. Regulation at the protein level is also suggested by the fact that the list of similarly or oppositely regulated genes at the RNA level includes very few that are connected either to metabolism or to transcription. It remains possible that relevant changes remain buried in the list of transcriptional targets because they change only by rather modest amounts that are below the thresholds set here but are nevertheless critical to the regulation of growth. More plausibly, a global regulator like the cyclin-dependent kinases or AMPK, which would not have to compete with cell-specific transcriptional programs, can respond to a systemic signal in broadly similar ways in all cells.
In conclusion, this study provides evidence that mitochondrial dysfunction triggers a systemic response that curtails the rate of growth of Drosophila larvae, and confirms a key role for pyruvate or its metabolic products in eliciting this signal.
Supplemental Material
Download Zip (4.1 MB)Acknowledgments
We thank Shweta Manjiry, who conducted some preliminary experiments relevant to this paper, Eveliina Teeri and Essi Eräsalo for technical assistance, and Troy Faithfull for help with the manuscript.
Disclosure statement
No potential conflict of interest was reported by the authors.During the review process of the manuscript, corresponding author HTJ was appointed as Editor-in-Chief of the journal. However, he remaineduninvolved in, and blinded to, the review and editorial process.
Supplementary material
Supplemental data for this article can be accessed here.
Additional information
Funding
References
- Thompson K, Collier JJ, Glasgow RIC, et al. Recent advances in understanding the molecular genetic basis of mitochondrial disease. J Inherit Metab Dis. 2019. [Epub before print]. DOI:10.1002/jimd.12104.
- Kanungo S, Morton J, Neelakantan M, et al. Mitochondrial disorders. Ann Transl Med. 2018;6:475.
- Murphy MP, Hartley RC. Mitochondria as a therapeutic target for common pathologies. Nat Rev Drug Discov. 2018;17:865–886.
- Boczonadi V, Horvath R. Mitochondria: impaired mitochondrial translation in human disease. Int J Biochem Cell Biol. 2014;48:77–84.
- Toivonen JM, O’Dell KM, Petit N, et al. Technical knockout, a Drosophila model of mitochondrial deafness. Genetics. 2001;159:241–254.
- Toivonen JM, Manjiry S, Touraille S, et al. Gene dosage and selective expression modify phenotype in a Drosophila model of human mitochondrial disease. Mitochondrion. 2003;3:83–96.
- Kemppainen E, George J, Garipler G, et al. Mitochondrial dysfunction plus high-sugar diet provokes a metabolic crisis that inhibits growth. PLoS One. 2016;11:e0145836.
- Wodarz A, Hinz U, Engelbert M, et al. Expression of crumbs confers apical character on plasma membrane domains of ectodermal epithelia of Drosophila. Cell. 1995;82:67–76.
- Yin S, Qun Q, Zhou B. Functional studies of Drosophila zinc transporters reveal the mechanism for zinc excretion in Malpighian tubules. BMC Biol. 2017;15:12.
- Osterwalder T, Yoon KS, White BH, et al. A conditional tissue-specific transgene expression system using inducible GAL4. Proc National Acad Sci USA. 2001;98:12596–12601.
- Berger C, Renner S, Lüer K, et al. The commonly used marker ELAV is transiently expressed in neuroblasts and glial cells in the Drosophila embryonic CNS. Dev Dyn. 2007;236:3562–3568.
- Sun B, Xu P, Wang W, et al. In vivo modification of Na+,K+-ATPase activity in Drosophila. Comp Biochem Physiol B Biochem Mol Biol. 2001;130:521–536.
- Oland LA, Biebelhausen JP, Tolbert LP. The glial investment of the adult and developing antennal lobe of Drosophila. J Comp Neurol. 2008;509:526–550.
- Górska-Andrzejak J, Salvaterra PM, Meinertzhagen IA, et al. Cyclical expression of Na+/K+-ATPase in the visual system of Drosophila melanogaster. J Insect Physiol. 2009;55:459–468.
- Edwards TN, Meinertzhagen IA. The functional organisation of glia in the adult brain of Drosophila and other insects. Prog Neurobiol. 2010;90:471–490.
- Stork T, Bernardos R, Freeman MR. Analysis of glial cell development and function in Drosophila. Cold Spring Harb Protoc. 2012;2012:1–17.
- Wang J-W, Beck ES, McCabe BD. A modular toolset for recombination transgenesis and neurogenetic analysis of Drosophila. PLoS One. 2012;7:e42102.
- Aberle H, Haghighi AP, Fetter RD, et al. wishful thinking encodes a BMP type II receptor that regulates synaptic growth in Drosophila. Neuron. 2002;33:545–558.
- Kemppainen KK, Rinne J, Sriram A, et al. Expression of alternative oxidase in Drosophila ameliorates diverse phenotypes due to cytochrome oxidase deficiency. Hum Mol Genet. 2014;23:2078–2093.
- Ranganayakulu G, Elliott DA, Harvey RP, et al. Divergent roles for NK-2 class homeobox genes in cardiogenesis in flies and mice. Development. 1998;125:3037–3048.
- Casso D, Ramírez-Weber FA, Kornberg TB. GFP-tagged balancer chromosomes for Drosophila melanogaster. Mech Dev. 1999;88:229–232.
- Castelli-Gair JE, Greig S, Micklem G, et al. Dissecting the temporal requirements for homeotic gene function. Development. 1994;120:1983–1995.
- Reiling JH, Hafen E. The hypoxia-induced paralogs Scylla and Charybdis inhibit growth by down-regulating S6K activity upstream of TSC in Drosophila. Genes Dev. 2004;18:2879–2892.
- Takeuchi T, Suzuki M, Fujikake N, et al. Intercellular chaperone transmission via exosomes contributes to maintenance of protein homeostasis at the organismal level. Proc National Acad Sci USA. 2015;112:E2497–E2506.
- Perkins LA, Holderbaum L, Tao R, et al. The transgenic RNAi project at harvard medical school: resources and validation. Genetics. 2015;201:843–852.
- Yan D, Neumüller RA, Buckner M, et al. A regulatory network of Drosophila germline stem cell self-renewal. Dev Cell. 2014;28:459–473.
- Pletcher RC, Hardman SL, Intagliata SF, et al. A genetic screen using the Drosophila melanogaster TRiP RNAi collection to identify metabolic enzymes required for eye development. G3. 2019:9:2061–2070.
- Ni JQ, Zhou R, Czech B, et al. A genome-scale shRNA resource for transgenic RNAi in Drosophila. Nat Methods. 2011;8:405–407.
- Dietzl G, Chen D, Schnorrer F, et al. A genome-wide transgenic RNAi library for conditional gene inactivation in Drosophila. Nature. 2007;448:151–156.
- Judd BH, Shen MW, Kaufman TC. The anatomy and function of a segment of the X chromosome of Drosophila melanogaster. Genetics. 1972;71:139–156.
- Royden CS, Pirrotta V, Jan LY. The tko locus, site of a behavioral mutation in Drosophila melanogaster, codes for a protein homologous to prokaryotic ribosomal protein S12. Cell. 1987;51:165–173.
- Shah ZH, O’Dell KMC, Miller SCM, et al. Metazoan nuclear genes for mitoribosomal protein S12. Gene. 1997;204:55–62.
- Merriam JR. FM7: a ‘new’ first chromosome balancer. Drosophila Inf Serv. 1969;44:101.
- Lindsley DL, Zimm GG. The genome of Drosophila melanogaster. NY: Academic Press; 1992.
- Barolo S, Carver LA, Posakony JW. GFP and beta-galactosidase transformation vectors for promoter/enhancer analysis in Drosophila. BioTechniques. 2000;29:726–732.
- Lee T, Luo L. Mosaic analysis with a repressible neurotechnique cell marker for studies of gene function in neuronal morphogenesis. Neuron. 1999;22:451–461.
- Fernandez-Ayala DJ, Sanz A, Vartiainen S, et al. Expression of the Ciona intestinalis alternative oxidase (AOX) in Drosophila complements defects in mitochondrial oxidative phosphorylation. Cell Metab. 2009;9:449–460.
- Brand AH, Perrimon N. Targeted gene expression as a means of altering cell fates and generating dominant phenotypes. Development. 1993;118:401–415.
- Finsterer J, Zarrouk-Mahjoub S. Biomarkers for detecting mitochondrial disorders. J Clin Med. 2018;7:16.
- Muha V, Müller HA. Functions and mechanisms of fibroblast growth factor (FGF) signalling in Drosophila melanogaster. Int J Mol Sci. 2013;14:5920–5937.
- Nässel DR, Liu Y, Luo J. Insulin/IGF signaling and its regulation in Drosophila. Gen Comp Endocrinol. 2015;221:255–266.
- Grönke S, Clarke DF, Broughton S, et al. Molecular evolution and functional characterization of Drosophila insulin-like peptides. PLoS Genet. 2010;6:e1000857.
- Gilbert LI. Halloween genes encode P450 enzymes that mediate steroid hormone biosynthesis in Drosophila melanogaster. Mol Cell Endocrinol. 2004;215:1–10.
- Niwa R, Niwa YS. Enzymes for ecdysteroid biosynthesis: their biological functions in insects and beyond. Biosci Biotechnol Biochem. 2014;78:1283–1292.
- Yamanaka N, Rewitz KF, O’Connor MB. Ecdysone control of developmental transitions: lessons from Drosophila research. Annu Rev Entomol. 2012;58:497–516.
- Zhang YQ, Roote J, Brogna S, et al. stress sensitive B encodes an adenine nucleotide translocase in Drosophila melanogaster. Genetics. 1999;153:891–903.
- Fergestad T, Bostwick B. Ganetzky B metabolic disruption in Drosophila bang-sensitive seizure mutants. Genetics. 2006;173:1357–1364.
- Chen S, Oliveira MT, Sanz A, et al. A cytoplasmic suppressor of a nuclear mutation affecting mitochondrial functions in Drosophila. Genetics. 2012;192:483–493.
- Vartiainen S, Chen S, George J, et al. Phenotypic rescue of a Drosophila model of mitochondrial ANT1 disease. Dis Models Mech. 2014;7:635–648.
- Jacobs HT, Fernández-Ayala DJ, Manjiry S, et al. Mitochondrial disease in flies. Biochim Biophys Acta. 2004;1659:190–196.
- Schell JC, Wisidagama DR, Bensard C, et al. Control of intestinal stem cell function and proliferation by mitochondrial pyruvate metabolism. Nat Cell Biol. 2017;19:1027–1036.
- Tennessen JM, Baker KD, Lam G, et al. The Drosophila estrogen-related receptor directs a metabolic switch that supports developmental growth. Cell Metab. 2011;13:139–148.
- Whitehouse S, Cooper RH, Randle PJ. Mechanism of activation of pyruvate dehydrogenase by dichloroacetate and other halogenated carboxylic acids. Biochem J. 1974;141:761–774.
- Gong F, Peng X, Sang Y, et al. Dichloroacetate induces protective autophagy in LoVo cells: involvement of cathepsin D/thioredoxin-like protein 1 and Akt-mTOR-mediated signaling. Cell Death Dis. 2013;4:e913.
- Woo SH, Seo SK, Park Y, et al. Dichloroacetate potentiates tamoxifen-induced cell death in breast cancer cells via downregulation of the epidermal growth factor receptor. Oncotarget. 2016;7:59809–59819.
- Yang Y, Sun Y, Chen J, et al. AKT-independent activation of p38 MAP kinase promotes vascular calcification. Redox Biol. 2018;16:97–103.
- Carpenter L, Halestrap AP. The kinetics, substrate and inhibitor specificity of the lactate transporter of Ehrlich-Lettre tumour cells studied with the intracellular pH indicator BCECF. Biochem J. 1994;304:751–760.
- De Preter G, Neveu MA, Danhier P, et al. Inhibition of the pentose phosphate pathway by dichloroacetate unravels a missing link between aerobic glycolysis and cancer cell proliferation. Oncotarget. 2015;7:2910–2920.
- Halestrap AP. The mitochondrial pyruvate carrier: kinetics and specificity for substrates and inhibitors. Biochem J. 1975;148:85–96.
- Wiemer EA, Michels PA, Opperdoes FR. The inhibition of pyruvate transport across the plasma membrane of the bloodstream form of Trypanosoma brucei and its metabolic implications. Biochem J. 1995;312:479–484.
- Halestrap AP, Price NT. The proton-linked monocarboxylate transporter (MCT) family: structure, function and regulation. Biochem J. 1999;343:281–299.
- Yang J, Ruchti E, Petit JM, et al. Lactate promotes plasticity gene expression by potentiating NMDA signaling in neurons. Proc National Acad Sci USA. 2014;111:12228–12233.
- Baufeld A, Vanselow J. Lactate promotes specific differentiation in bovine granulosa cells depending on lactate uptake thus mimicking an early post-LH stage. Reprod Biol Endocrinol. 2018;16:15.
- Wu CY, Satapati S, Gui W, et al. A novel inhibitor of pyruvate dehydrogenase kinase stimulates myocardial carbohydrate oxidation in diet-induced obesity. J Biol Chem. 2018;293:9604–9613.
- Hildyard JC, Ammälä C, Dukes ID, et al. Identification and characterisation of a new class of highly specific and potent inhibitors of the mitochondrial pyruvate carrier. Biochim Biophys Acta. 2005;1707:221–230.