Abstract
Ultrafiltration membranes were prepared using cellulose acetate (CA) as a polymer, LiCl and CaCl2 as porogens and methyl-(S)-lactate as a solvent. CA, methyl lactate and the porogens used in this work are obtained from renewable resources; they are biodegradable, non-toxic and non-volatile organic compounds. Flat sheet ultrafiltration membranes were prepared by the phase inversion technique. A molecular weight cut-off between 15 and 35 kDa (polyethylene glycol) and pure water permeability between 13 and 177 litres h− 1m− 2 bar− 1 were obtained. These parameters are in the ideal range for water treatment industry. Improvement of pollutant degree and ecotoxicity of the process was evaluated by ‘green’ metrics by the P (pollutants, persistent and bioaccumulative) and E (ecotoxicity) parameters. Both of these variables were recorded as zero using our method. This study represents a step ahead towards the production of ultrafiltration polymeric membranes by a ‘greener’ process than current methods.
1. Introduction
It is widely acknowledged that there is a growing need for more environmentally acceptable processes in the chemical industry. This trend towards what has become known as ‘green chemistry’ or ‘sustainable engineering’ needs research on technologies that use preferably renewable raw materials, produce less waste and avoid the use of toxic and hazardous reagents and solvents (Anastas and Warner Citation1998).
In this context, biosolvents have been considered as one type of product meant to reduce pollution and environmental footprints. They are now widely used in cosmetics, pharmaceuticals and food industries. Some of them, such as fatty acid alkyl esters, are even used as biolubricants, a rapidly expanding field. Ethyl lactate, for example, has been proposed as a substitution solvent in magnetic tapes industry in the past (Nikles et al. Citation2001).
Membrane technology that is considered as a waste- and energy-efficient process could better control their environmental footprint simply by using such ‘green’ solvents. One of the most commonly used materials to prepare membranes is cellulose acetate (CA). This polymer is obtained by introducing the acetyl radical of acetic acid into cellulose (cotton or wood), which is renewable in nature (Siracusa et al. Citation2008).
Initially, CA membranes were developed for desalination of water by reverse osmosis, but essentially identical membranes have been used in pervaporation to dehydrate alcohol and are currently used in gas permeation to separate carbon dioxide from natural gas (Loeb and Sourirajan Citation1963, Baker Citation2004). Ultrafiltration CA membranes are currently used in water treatment, where the accepted molecular weight cut-off (MWCO) must be between 15 and 25 kDa and flux should be as high as possible, but at least 150 litres h− 1m− 2. Currently, membranes obtained from this polymer are inexpensive and easy to produce. Additionally, it has been shown that CA is biodegradable (depending on its degree of acetylation), being hydrolysed into ethyl alcohol and lactic acid, common constituents of food (Ishigaki et al. Citation2004).
CA membranes are currently fabricated by wet phase inversion. In this technique, a polymer and a porogen are solubilised in a suitable solvent (dope solution). This dope solution is cast on a glass plate and the film obtained is then immersed into a coagulation bath (a non-solvent of the polymer). Solvent and non-solvent exchange occurs, leading to a phase inversion. A flat sheet polymeric membrane is then obtained. The same method is also applied to produce hollow fibres by extrusion of the dope solution. Depending on the polymer, solvents currently used in dope solutions are N-methyl-2-pyrrolidinone (NMP), N,N-dimethylformamide (DMF), dimethyl sulphoxide (DMSO), acetone, cyclohexane, chloroform, etc. Some of these solvents are considered volatile organic compounds (VOCs) and some of them are harmful to environment or to human health (Table ).
Table 1 Environmental and human health risks of commonly used solvents. Ethyl and methyl lactates, as well as FAME, are presented for comparison purposes.
Another inconvenience of the use of current solvents is that they are non-renewable resources (Browning Citation1987, Sigma–Aldrich Citation1988, Browning Citation1990, Browning Citation1992, Lewis and van Nostrand Citation1996, ILO Citation1998, Gerin Citation2002). The case of the NMP is special as this solvent is not yet considered as dangerous, but its toxicological classification is expected to change as ‘toxic and dangerous to reproduction’ (INRS Citation2007). Inexpensive natural products with the added advantage of being readily biodegradable are currently being used as environmentally attractive solvents. Table shows the properties of some of these solvents.
As the solvents used in the coagulation bath must not solubilise the polymer, water is frequently employed because it is cheap, easy to use and it does not solubilise most of the polymers. A mixture of a solvent and a non-solvent (of polymer) is sometimes used to control phase inversion.
During the membrane manufacturing process, the solvent contained in the dope solution is lost in the water used as the coagulation bath. The mixture of the solvent from the dope solution and the non-solvent from the coagulation bath is sent directly to the wastewater treatment plant or to a recycling unit. Treatment of these effluents is not easy due to their toxicity, volatility and environmental implications of the solvents used. This is the key point in the management of effluents from membrane production, as these solvents must be treated before disposal. When a treatment is possible, it is generally expensive.
The environmental impact of membrane production processes can hence be improved. An important component of green chemistry is the search for new solvents to replace VOCs and other toxic, mutagenic or carcinogenic solvents. Ideally, solvent substitution should reduce toxicity and volatility and should be obtained from renewable resources as stated in principle 4 of green chemistry: safer chemicals (Anastas and Warner Citation1998, Anastas Citation2002, Chang Citation2002, Warner et al. Citation2004). In addition, reverse osmosis and ultrafiltration membranes for the production of drinking water have a 10% per year expansion. According to a study published by Global Markets Direct Citation2010, the membrane treatment market for industrial water and wastewater is expected to grow at a compound annual growth rate (CAGR) of 13% from $2.3 billion in 2008 through 2015 to reach $5.5 billion. Globally, membrane treatment is likely to witness 15% CAGR growth during 2008–2015.
Ultrafiltration has a broad variety of applications ranging from the processing of biological macromolecules to wastewater treatment. Food and biotechnological applications account for nearly 40% of the current total usage of ultrafiltration membranes. Processing of biological macromolecules, such as proteins and nucleic acids, has assumed a significant importance in the bioprocess industry, where the impact of downstream processing on the overall process economics is now better appreciated (Rizvi Citation2009). The use of ultrafiltration in the biotechnology industry is growing even faster than the sector itself. Purchasing of ultrafiltration membranes and modules grows at the rate of $300 million and crossflow equipment at a rate of $700 million per year only in biotechnology and pharmaceutical industries (McIlvaine Citation2005). The US market for membrane modules used in liquid and gas separations is expected to be worth $2.3 billion in 2008. This will increase to $3.3 billion in 2013, for a CAGR of 7.8%.
Preparation of ultrafiltration membranes showing characteristics similar to those employed in water production processes by using a greener route than the traditional one presents a challenge. The aim of this work was to prepare CA membranes by substituting the solvents used traditionally such as NMP by greener solvents.
2. Experimental
2.1 Determination of suitable solvents and porogens
The first step was to find a substitute solvent for CA. This solvent must be water miscible in all proportions and solubilise the porogens and the polymer, giving a suitable solution to form a membrane. For instance, fatty acid methyl esters (FAME) could not be tested due to their poor solubility in water. Hansen solubility parameters were used as a first approach (Hansen Citation2000) to create a short list of potential candidates. The criterion to choose the candidate was the distance between the solvents and CA points on the Hansen 3D space (d), calculated by Equation (Equation1). These distances are shown in Table .
The short distance between methyl lactate and CA suggested that it could be a suitable solvent.
As the pore size distribution of a membrane plays a key role on its performance, the porogen is an important component of the preparation process. Two porogens currently used in the phase inversion membranes production are polyvinylpyrrolidone (PVP) and PEG of different molecular weights. Toxicities of PVP and PEG are still not completely known (Sigma–Aldrich Citation1998). Nevertheless, neither of them is produced from renewable resources because they are petrochemicals.
Salts can be used as porogens and we have selected LiCl and CaCl2 for our experiments.
2.2 Membrane preparation
Methyl-(S)-lactate was kindly provided by Purac, Barcelona, Spain (97% purity). CA-398-3 was obtained from Eastman, Poitiers, France. LiCl and CaCl2 (99% purity) were purchased from Sigma-Aldrich, Lyon, France. All products were used without further purification. Membranes were obtained by wet phase inversion. Concentration of the polymer in Loeb–Sourirajan type porous membranes is normally found between 13 and 17%. Nevertheless, concentrations as high as 23% have already been studied (Mulder Citation1997, Baker Citation2004). Solutions of CA in methyl lactate were prepared at concentrations of 16 and 20% w/w in the following way: CA was solubilised in methyl lactate at 80°C, and LiCl was then added at 6% w/w and mixed thoroughly overnight at 80°C. When observing the literature, porogens such as polyethylene glycol are not added in a concentration higher than 10% to the cast solution as the viscosity makes it impossible to be cast. After adding the porogen, solutions were allowed to reach room temperature prior to casting. The solutions were cast on a glass plate using a Gardner knife with a gap of 120 μm, and the glass plate was immediately immersed in water at 25°C as the coagulation bath. In order to observe the effect of a more readily available salt, the best of the membranes obtained with LiCl was prepared with CaCl2 this time. This membrane was prepared using CaCl2 instead of LiCl at 6% and a concentration in CA of 20%. Dope solution was prepared in the same way as with LiCl.
2.3 Membrane characterisation
The obtained membranes were characterised using a scanning electronic microscope (SEM) (JEOL JSM 6700F). Cross sections of the membranes were obtained by breaking the membranes after they had been immersed successively in methanol and liquid nitrogen for a few seconds. Pure water permeability, solution flux and MWCO were measured using an Amicon cell (Millipore, Model 8050; Molsheim, France) on the equipment sketched in Figure . Solutions of PEG of various molecular weights (Table ) at 1 mg/ml were used for MWCO tests according to the method developed by Causserand et al. (Citation2004). This method minimises the effects of concentration polarisation by successive filtrations at various transmembrane pressures (or various permeation fluxes) of the same PEG solution in order to obtain, by extrapolation, the retention at zero pressure (or zero flux). This method allows the determination of the intrinsic retention of a PEG tracer, which is independent of the operating conditions. Concentrations of PEG in permeate and retentate were determined by total organic carbon (TOC) using a TOC standard model equipment TOC-VCSN (Combustion catalytic oxidation/NDIR method, standalone, standard model) from Shimadzu. As a measure of resistance of these membranes to normally exerted stress, tests of breaking pressure were effectuated by using an Amicon cell in the same equipment sketched in Figure . These measurements enabled us to compare the performance of our membranes with those that are commercially available. In all cases, no membrane compaction before breaking pressure was observed in our experiments.
Figure 1 Lab-scale equipment for mass transfer characterisation (permeability, PEG retention and MWCO determination).
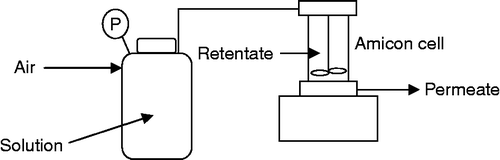
Table 2 Molecular mass and hydrodynamic radius of the PEG used on the MWCO determination.
3. Results and discussion
Hansen parameters allowed us to find that alkyl lactates could replace solvents such as NMP, chloroform, acetone, etc. As shown in Table , in the Hansen 3D space, the distance between methyl lactate and CA is small compared to the distance between NMP and CA. This suggested that methyl lactate could be a good solvent for the polymer. The distance between CA and ethyl lactate was the smallest, but solubility tests revealed that methyl lactate was the best solvent for membrane preparation as the viscosity of the final solution allowed us to prepare the membranes by the Gardner knife technique. When ethyl lactate was used, the viscosity did not allow this step. Lowering the quantity of CA in the dope solution to obtain a good viscosity leads to less-resistant membranes. Nevertheless, a future investigation of the possible use of ethyl lactate may be worthwhile.
When substituting solvents, capacity of the substituting solvent to be used in large scale, as well as its cost and availability, becomes crucial. Methyl lactate has been largely considered as a green solvent and its production at large scale has been strongly encouraged. The Argonne National Laboratory in its programme for the production of methyl lactate to be used as an intermediate for the production of a host of other chemicals, polymers and specialty derivatives has shown that a manufacturing cost of methyl lactate as 29.1 ¢/lb is achievable (Datta et al. Citation1995). This allows the thinking that market and prices of green solvents such as alkyl lactates will become interesting in the near future. Temperature for preparing the dope solution has not been optimised, and has been chosen to minimise the time to prepare it in the laboratory, nevertheless, as CA is soluble in methyl lactate at room temperature (Mishra Citation2000), dope solutions could be prepared at 25°C.
The characteristics of the membranes obtained are summarised in Table . The pure water permeabilities obtained were between 13 and 177 litres h− 1m− 2 bar− 1. The breaking pressures were from 3 to more than 5 bars. In all cases, no compaction was observed before membrane rupture. The breaking pressure of the membrane prepared with CaCl2 was the highest of all of the membranes prepared (i.e. 5 bars). As the CA concentration increased from 16 to 20% with LiCl as the porogen, pure water permeability decreased from 177 to 23 litres h− 1m− 2 bar− 1.
Table 3 Membranes MWCO, pure water permeability and breaking pressure.
Intrinsic retention vs. molecular weight of PEG for three membranes is shown in Figure . The hydrodynamic radii (r hyd) of the PEG molecules were calculated as a function of the molecular weight using Figure (Meireles et al. Citation1995, Causserand et al. Citation2004):
The MWCO of the membranes obtained by the method described varied between 15 kDa (5.7 nm in hydrodynamic radius) and 35 kDa (9.2 nm) using PEG as a tracer. The cut-off of the membrane is also given using the hydrodynamic radius for comparison with other tracers such as dextrans (Meireles et al. Citation1995, Causserand et al. Citation2004). The characteristics of the three membranes were in the range of the ultrafiltration as defined by the European Society of Membrane Science and Technology, with MWCO between 10 and 500 kDa (Koops Citation1995). The membrane fabricated using CaCl2 as the porogen showed the lower MWCO with 15 kDa. The membranes prepared had a rejection comparable to or better than those found in the literature when CA membranes are prepared in the presence of NMP as a solvent and PEG or PVP as porogens, where rejections of 20–69 kDa are currently found (Lafrenière et al. Citation1987, Raguime et al. Citation2007).
In the case of membranes prepared with LiCl, the MWCO changed with the concentration of the polymer of the dope solution: a MWCO of 35 kDa was observed in a membrane prepared from a solution with 16% of the polymer, while a membrane prepared with 20% of CA shown a MWCO of 20 kDa. This behaviour has already been reported, for example, by Lafrenière et al. (Citation1987) who prepared polysulphone ultrafiltration membranes using NMP as a solvent and PVP as a porogen. The authors also observed a direct dependence of PEG retention on the polymer concentration with MWCO varying from 7 to 3 kDa when the polymer concentration increased from 15 to 20%.
Microphotographs obtained by SEM are shown in Figures commercial membrane Sterlitech CA UF CQ (CA, ultrafiltration, GE Osmonics) in CA is shown in Figure for comparison purposes. A high number of macrovoids were observed on membranes produced using LiCl (see Figures and ), together with a sponge-like structure (Figures (b) and (b)). The membrane obtained with 16% CA and 6% LiCl (Figure ) shows a porous structure (Figure (b)). This structure is characteristic of a membrane with high flux and MWCO (Strathmann et al. Citation1975). These membranes are currently used in low-pressure ultrafiltration applications. A structure like the one shown in Figure (b), where finger-like pores are isolated on a sponge-like structure, reveals that during phase inversion, the pore initiation was difficult, but once the pore was formed it could have penetrated across the entire membrane. This structure is between a structure with finger-like pores and a sponge-like one. The presence of macrovoids in membranes is often undesirable. Structures containing macrovoids tend to be fragile or defective, as the macrovoids can be connected to surface pores giving a defective membrane (Zeman and Zydney Citation1996, Remigy and Meireles Citation2006, Remigy Citation2009).
Figure 3 SEM image of the skin (a) and of the cross section (b) of the membrane obtained with 16% w/w CA, 6% LiCl and methyl lactate as a solvent.
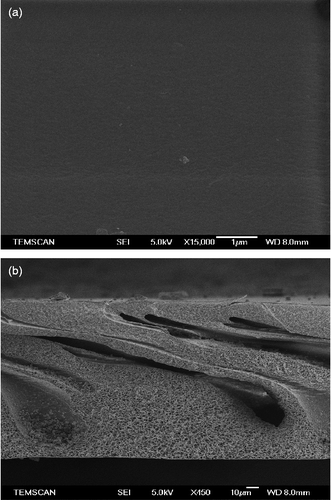
Figure 4 SEM image of the skin (a) and of the cross section (b) of the membrane obtained with 20% w/w CA, 6% LiCl and methyl lactate as a solvent.
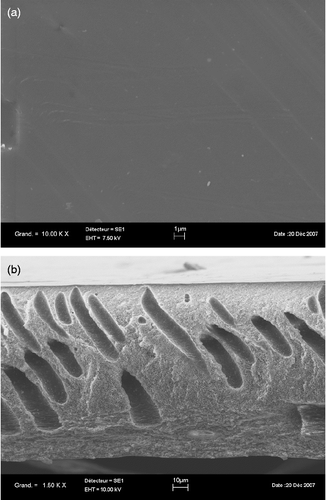
Figure 5 SEM image of the skin (a) and of the cross section (b) of the membrane obtained with 20% w/w CA, 6% CaCl2 and methyl lactate as a solvent.
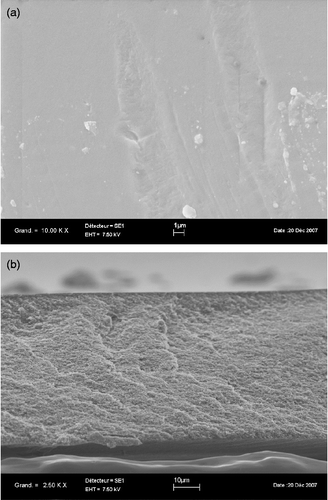
Figure 6 SEM image of the skin (a) and of the cross section (b) of commercial membrane Sterlitech CA UF CQ.
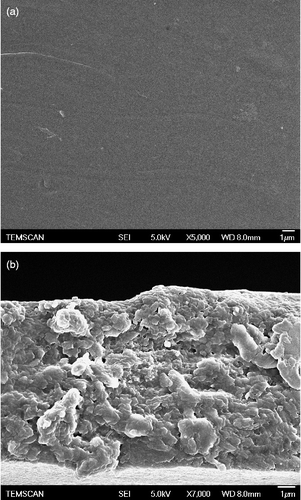
The width and the length of the macrovoids seemed to be dependent on the CA concentration in the dope solution, as shown in Figures (b) and (b). The shape of the macrovoids shown in Figure (low polymer concentration) seems to be compressed during membrane formation. These macrovoids lead to a more fragile structure as demonstrated by the low breaking pressures observed.
For membranes obtained with a solution of 20% of CA and CaCl2 as a porogen (Figure ), no macrovoids were present (Figure (b)), whereas a spongy structure was observed with pore diameters in the micrometre range. It is interesting to observe that the same structure can be found in the commercial sample of the ultrafiltration CA membrane (Figure ). In our case, this membrane was the most resistant to pressure of all the membranes studied. This is undoubtedly due to the homogeneous sponge-like structure and the lack of macrovoids. Performance of this membrane is comparable to the commercial ultrafiltration membrane (Table ) with a MWCO in the 15–20 kDa range and a pure water permeability in the 10–15 litres h− 1m− 2 bar− 1 range. Although these results are encouraging, we believe our membranes could be further improved to obtain better pure water fluxes.
The transition between a finger-like pore structure and a sponge-like structure in our membranes agrees with results cited previously by Smolders et al. (Citation1992), where an increase in the CA concentration decreases the apparition of macrovoids. In our case, an increase from 16 to 20% decreased the pore size (membrane with 6% LiCl) or completely prohibited it (membrane with 6% CaCl2). Nevertheless, in the membranes with 20% CA but different porogens (6% of LiCl or CaCl2), the difference between the presence or absence of pores can be explained by a phenomenon observed before by Li et al. (Citation2004). These authors have observed the dependence of the transition between sponge-like and finger-like pore structures on the thickness of the membrane. In our case, even if all the membranes were cast with the same Gardner knife, different thicknesses were obtained. These differences strongly influenced the structure: the thinnest membrane presented a sponge-like structure (20% CA and 6% CaCl2, 58.3 μm); at the highest thickness, we observed a finger-like pore structure (16% CA, 6% LiCl, 140 μm). The membrane with the thickness in between presented a transition between the two structures (20% CA, 6% LiCl, 104 μm).
3.1 Quantification of the ‘green’ character of the membrane obtained
In order to quantify the improvement due to the use of methyl lactate instead of NMP as the solvent in membrane production, we applied the ‘green’ metrics as proposed by Curzons et al. (Citation2001). We chose to calculate the variable P in the category pollutants, persistent and bioaccumulative and the variable E for ecotoxicity, taking into account that membrane preparation is a process without reaction.
In the category pollutants, persistent and bioaccumulative, the variable P is calculated as
If we consider a classical dope solution with NMP as a solvent and concentrations close to our dope solutions: 16% of CA, 6% of porogen and 78% of NMP, P = 4.875 kg/kg. If the concentration of CA is 20%, 6% of porogen and 74% of solvent, then P = 3.7 kg/kg. Given that legislation for NMP will change to consider it as ‘toxic and dangerous to reproduction’, all the NMPs were considered for the P calculation. LiCl was considered as the porogen, but if PVP is used as currently done, then P will increase.
In the case of methyl lactates used as solvents, they are not persistent or bioaccumulative, so, in all cases, P = 0 kg/kg.
In the category ecotoxicity, E is estimated as
For NMP, E = 20 kg for 78% in solvent and 18.9 kg for 74% of NMP concentration, if we take the LD50 in oral ingestion for rats (Table ). All the NMPs were used on calculation of E. In the case of methyl lactate, E = 0 kg.
According to these ‘green’ metrics, the substitution of solvents such as NMP by ‘green’ solvents such as methyl lactate improves membrane production by decreasing the use of persistent, bioaccumulative and ecotoxic solvents, going towards a greener membrane production than the actual process. Additionally, the characteristics of the membrane produced using alkyl lactates are comparable to its commercially available equivalent in terms of resistance to pressure, permeability and MWCO. The influence of major parameters, such as the concentration in polymer or porogen is also similar to the one observed with more classical routes.
4. Conclusions
CA membranes were obtained using a biodegradable and non-VOC solvent. Methyl lactate allowed the preparation of ultrafiltration membranes using LiCl and CaCl2 as porogens.
The utilisation of CaCl2 as the porogen resulted in a ‘sponge-like’ structure. This structure is very appropriate for the preparation of defect-free, pressure-resistant membranes at low rejection rates. Membranes prepared with LiCl contained macrovoids and could present some defects at the membrane surface. All the prepared membranes could have been classed as ultrafiltration membranes with a MWCO lower than 500 kDa. A possible utilisation of these membranes is the ultrafiltration of water in transmembrane pressure domains up to 5 bars. Although the breaking pressure measurements allowed us a comparison with commercially available membranes, a deeper study of mechanical properties as a function of composition is needed.
The preparation process described in this study is in agreement with principle 7 of green chemistry: renewable feedstocks. As presented in Table , methyl lactate does not present any known risk. The use of these solvents meets principle 5 of green chemistry: use of alternative solvents. The handling of these products is less dangerous than commonly used solvents, agreeing with principle 12: accident prevention.
As shown by the ‘green’ metrics, the substitution of currently used solvents by methyl lactate would improve the process by decreasing the use of persistent and bioaccumulative materials and ecotoxicity. Our method to produce membranes not only reduces pollution and environmental footprints but also allows the preparation of membranes with the criteria needed to be used in water treatment. This first step in this direction suggests that it is possible to rethink the membrane production process, so as to move towards a greener process.
Acknowledgement
The authors gratefully thank PURAC for kindly providing the alkyl lactate samples.
Additional information
Notes on contributors
Y. Medina-Gonzalez
1Notes
1. Current address: Department of Chemical and Biochemical Engineering, Faculty of Engineering, University of Western Ontario, London, Ontario, Canada, N6A5B9
References
- Anastas , P.T. 2002 . “ Clean solvents: alternative media for chemical reactions and processing ” . In ACS symposium series 819 , 1 – 9 . Washington DC : American Chemical Society .
- Anastas , P.T. and Warner , J.C. 1998 . Green chemistry: theory and practice , New York : Oxford University Press .
- Baker , R.W. 2004 . Membrane technology and application , New York : McGraw-Hill .
- Browning , E. 1987 . Toxicity and metabolism of industrial solvents. Volume I: hydrocarbons , Edited by: Snyder , R. New York : Elsevier .
- Browning , E. 1990 . Toxicity and metabolism of industrial solvents. Volume II: nitrogen and phosphorus solvents , Edited by: Snyder , R. New York : Elsevier .
- Browning , E. 1992 . Toxicity and metabolism of industrial solvents. Volume III: alcohols and esters , Edited by: Snyder , R. New York : Elsevier .
- Causserand , C. 2004 . Improvement of a method for the characterization of ultrafiltration membranes by measurements of tracers retention . Journal of Membrane Science , 238 : 177 – 190 .
- Chang , J. 2002 . Vertec biosolvents on verge of breaking out with new replacement applications . Chemical Marketing Report , 267 : 4
- Curzons , A.D. 2001 . So you think your process is green, how do you know?: using principles of sustainability to determine what is green: a corporate perspective . Green Chemistry , 3 : 1 – 6 .
- Datta , R. 1995 . Technological and economic potential of poly(lactic acid) and lactic acid derivatives . FEMS Microbiology Reviews , 16 : 221 – 231 .
- Gerin , M. 2002 . “ Solvants industriels ” . In Santé, sécurité, substitution, collection médecine du travail , Edited by: Masson , A. Paris : Masson .
- Global Markets Direct, 2010. Global membrane market for water and wastewater treatment forecasts and analysis to 2015. Global Markets Direct Rapport. Available from: http://www.globalmarketsdirect.com/[Accessed 11 May 2010]
- Hansen , C.M. 2000 . Hansen solubility parameters, a user's handbook , Boca Raton, FL : CRC Press LLC .
- ILO, 1998. Encyclopaedia of Occupational Health and Safety, International Labour Office. Available from: http://www.ilocis.org/en/default.html [Accessed 11 May 2010]
- INRS, 2007. Etiquetage des Substances et Préparations chiMiques Dangereuses. Available from: http://www.inrs.fr/htm/etiquetage_substances_preparations_chimiques.html [Accessed 11 May 2010]
- Ishigaki , T. 2004 . The biodegradability of biodegradable plastics in aerobic and anaerobic waste landfill model reactors . Chemosphere , 54 : 225 – 233 .
- Koops , G.H. 1995 . Nomenclature and symbols in membrane science and technology , Toulouse : The European Society of Membrane Science and Technology .
- Lafrenière , L.Y. 1987 . Effect of polyvinylpyrrolidone on the performances of polyethersulfone ultrafiltration membranes . Industrial and Engineering Chemistry Research , 26 : 2385 – 2389 .
- Lewis , R.J. and van Nostrand , R. 1996 . Sax's dangerous properties of industrial materials , New York : John Wiley and Sons .
- Li , D. 2004 . Thickness dependence of macrovoid evolution in wet phase-inversion asymmetric membranes . Industrial and Engineering Chemistry Research , 43 : 1553 – 1556 .
- Loeb , S. and Sourirajan , S. 1963 . Sea water demineralization by means of an osmotic membrane . Advances in Chemistry Series , 38 : 117 – 132 .
- McIlvaine . 2005 . Sales of membrane systems set to grow . Membrane Technology , 9 : 4
- Meireles , M. 1995 . An appropriate molecular size parameter for porous membranes calibration . Journal of Membrane Science , 103 : 105 – 115 .
- Mishra , S.P. 2000 . A textbook of fibre, science and technology , 184 – 186 . New Delhi : New Age International Limited .
- Mulder , M. 1997 . Basic principles of membrane technology , Dordrecht : Kluwer Academic Publishers .
- Nikles , S.M. 2001 . Ethyl lactate: a green solvent for magnetic tape coating . Green Chemistry , 3 : 109 – 113 .
- Raguime , J.A. 2007 . Performance characterization of cellulose acetate and poly(vinylpyrrolidone) blend membranes . Journal of Applied Polymer Science , 104 : 3042 – 3049 .
- Remigy , J.C. 2009 . “ X-ray tomography application to 3D characterization of membranes on monitoring and visualizing membrane-based processes ” . In Monitoring and visualizing membrane based process , Edited by: Güell , C. , Ferrando , M. and López , F. Weinheim : Wiley-VCH Verlag GmbH & Co. KGaA .
- Remigy , J.C. and Meireles , M. 2006 . Assessment of pore geometry and 3D architecture of filtration membranes by synchrotron radiation computed microtomography . Desalination , 199 : 501 – 503 .
- Rizvi , S.S.H. 2009 . “ Membrane applications in biotechnology, food processing, life sciences, and energy conversion: introduction ” . In Handbook of membrane separations: chemical, pharmaceutical, food, and biotechnological applications , Edited by: Pabby , A.K. , Rizvi , S.S.H. and Sastre , A.M. Boca Raton, FL : CRC Press, Taylor & Francis Group .
- Sigma‐Aldrich, 1998. The Sigma-Aldrich Library of Chemical Safety Data Vols. 1 and 2. Available from: http://www.sigmaaldrich.com/safety-center.html [Accessed 11 May 2010]
- Siracusa , V. 2008 . Biodegradable polymers for food packaging: a review . Trends in Food Science and Technology , 19 : 634 – 643 .
- Smolders , C.A. 1992 . Microstructures in phase-inversion membranes. Part 1. Formation of macrovoids . Journal of Membrane Science , 73 : 259 – 275 .
- Strathmann , H. 1975 . The formation mechanism of asymmetric membranes . Desalination , 16 : 179 – 203 .
- Warner , J.C. , Cannon , A.S. and Dye , K.M. 2004 . Green chemistry . Environmental Impact Assessment Review , 24 : 775 – 799 .
- Zeman , L.J. and Zydney , A.L. 1996 . Microfiltration and ultrafiltration, principles and applications , 115 New York : Marcel Dekker, Inc. .