ABSTRACT
In many European countries, the environmental properties of waste-derived aggregates are mostly assessed based on laboratory leaching tests such as the standardised percolation tests CEN/TS/14405, ISO/TS 21268-3, or DIN 19528. These tests are conducted under specified conditions, which are similar yet somewhat different from realistic field conditions. Therefore, this study aimed to investigate the leaching properties of ADR (Advanced Dry Recovery) recovered MSWI BA in the field in order to understand more about its environmental impacts in actual civil engineering structures. Two field scale studies (a lysimeter and a larger interim storage field study) were constructed and the leachate quality was investigated. These results were then complemented with the results of previously conducted laboratory leaching tests using a liquid to solid ratio (L kg−1) comparison. The results demonstrated that the leaching behaviours of many potentially harmful substances, such as chloride, copper and antimony, was similar despite the study scale. In addition, this study illustrated the importance of investigating the leaching properties of waste-derived aggregates on a larger scale, even though the uncertainties in such studies may not be easily controlled.
1. Introduction
At the end of 2015, the European Commission (EC) launched its renewed Circular Economy Package (EC Citation2015), promoting Europe as a recycling society, and special emphasis is given to the recycling and utilisation of waste materials. In civil engineering, the utilisation of waste-derived aggregates is especially interesting as large amounts of materials are required in the different types of structures (Prezzi et al. Citation2011). In addition, less greenhouse gas emissions are produced, and the carbon footprint decreases when waste aggregates are used instead of virgin raw materials (Vandecasteele Citation2015).
Despite the possible economic value of waste-derived aggregates, the environmental properties should, however, be assessed in detail in order to ensure a safe and sustainable use of these materials. In many countries, the environmental properties of waste-derived aggregates are mostly assessed on the basis of laboratory leaching tests such as the standardised percolation tests CEN/TS/14405, ISO/TS 21268-3, or DIN 19528 (DIN Citation2009; ISO Citation2007; SFS Citation2004). Such tests are nowadays conducted under specified conditions, which are similar but yet for practical reasons somewhat different from realistic field conditions (Åberg, Kumpiene, and Ecke Citation2006; Flyhammar and Bendz Citation2006; Lidelöw and Lagerkvist Citation2007; Schreurs, van der Sloot, and Hendriks Citation2000; de Windt et al. Citation2011). For example, hydrology and climatic factors, temperature differences, pH and redox conditions can strongly affect the leaching behaviours of different substances in the field.
Since the beginning of the 2000s, several studies have focused on assessing the leaching behaviour of, for example, municipal solid waste incineration bottom ash (MSWI BA) in larger-scale field studies (e.g. Åberg, Kumpiene, and Ecke Citation2006; Bruder-Hubscher et al. Citation2001; Dabo et al. Citation2009; Flyhammar and Bendz Citation2006; François and Pierson Citation2009; Hjelmar, Holm, and Crillesen Citation2007; Izquierdo et al. Citation2008; Lidelöw and Lagerkvist Citation2007; Schreurs, van der Sloot, and Hendriks Citation2000; de Windt et al. Citation2011). The investigation of MSWI BA has been of great interest to many researchers, the industry and authorities, since approximately 18 million tonnes of BA is produced across Europe annually (CEWEP Citation2016). The technical properties of this material are comparable to those of natural aggregates (Chandler et al. Citation1997; Izquierdo et al. Citation2001; Sormunen and Rantsi Citation2015), which makes its utilisation in civil engineering appealing.
The preceding field scale studies on the leaching properties of MSWI BA have been conducted using conventionally treated MSWI BA, whereas this study focused on investigating the leaching properties of MSWI BA recovered with a novel Dutch dry treatment technology called ADR (Advanced Dry Recovery). The aim was to study materials’ leaching properties in the field in order to understand more about its environmental impacts on actual civil engineering structures. This particular material was chosen because its leaching properties have not yet been studied, especially with larger field scale studies. The principle behind ADR technology is somewhat different from those of conventional dry treatment processes that are listed, for example in the reference document on the best available techniques for waste incineration (WI BREF) (EC Citation2006). The ADR technology has a ballistic separator for the removal of fine fractions, which improves the recovery of nonferrous metals from the BA (de Vries and Rem Citation2013). In turn, the environmental compatibility of mineral fractions should be improved as well. The use of ADR technology has rapidly increased in different places around the world, and at the moment, it treats up to five million tonnes of MSWI BA in Europe, Asia and North America (Inashco B.V., personal communication).
In this study, the leaching of ADR-recovered BA was studied with two types of field scale studies: a lysimeter and a larger interim storage field study. Among other hydrological parameters, each study investigated the leachate quality. These results were then complemented with the results of previously conducted laboratory leaching tests (Sormunen and Rantsi Citation2015; Sormunen et al. Citation2016). The comparison between field and laboratory tests was made as a function of Liquid to Solid ratio (L S−1, L kg−1) calculated for each test scenario as already applied for conventionally treated BA (e.g. Hjelmar, Holm, and Crillesen Citation2007). Such a comparison between laboratory and field scale results is important in order to assess materials’ environmental impacts on a larger scale. In addition, the scalability and reliability of laboratory leaching tests can be estimated.
In general, the study also aimed to illustrate the importance of investigating the leaching properties of waste-derived aggregates on a larger scale, even though the data can be limited or the uncertainties in such studies may not be that easily controlled. In addition, the comparability between field scale results and the results from a controlled laboratory environment often require simplifications that can cause additional uncertainty in such comparison studies.
2. Material and methods
2.1. Study structure and materials
illustrates the research structure. The MSWI BA used in the study was originally generated in a waste incineration plant located in the western part of Finland. The grate-fired plant (1 000 °C) incinerates approximately 180 000 t of mainly source-separated household waste, which yields approximately 30 000 t of BA in a year.
Figure 1. The study structure (ADR: Advanced Dry Recovery; MSWI BA: Municipal solid waste incineration bottom ash; NF: Non-ferrous; F: Ferrous; I–IV: referred to in the text).
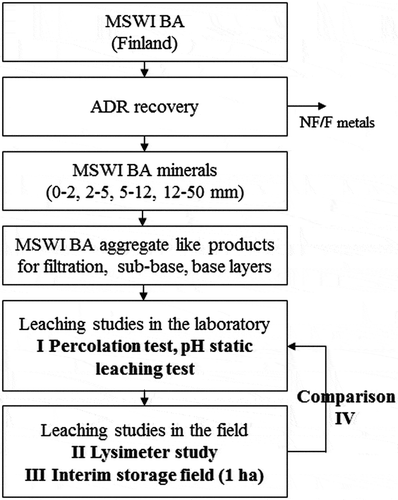
In the summer of 2013, the annual amount of MSWI BA was first treated with the ADR dry treatment method to improve the removal of non-ferrous (NF) and ferrous (F) metals from the minerals. A more detailed description of the treatment process is given in Sormunen et al. (Citation2016). The ADR process generates approximately 75–85 weight-% (w-%) of four different size fractions of MSWI BA minerals (0–2, 2–5, 5–12 and 12–50 mm). Samples of these mineral fractions were taken separately for laboratory analysis during the treatment process, as described in Sormunen and Rantsi (Citation2015).
The grain size distributions of individual BA mineral fractions illustrated in Sormunen and Rantsi (Citation2015) were not in all cases suitable for unbound structural layers of roads and field structures as defined by the Finnish quality criteria for infrastructure construction by Rakennustietosäätiö RTS (Citation2010). Therefore, aggregate-like products were designed for the unbound structural layers (filtration, sub-base and base layers) of the interim storage field and the lysimeters. A more detailed description of this BA product design is given in Sormunen et al. (Citation2016). summarises the proportions of each mineral fraction in the treated BA aggregate-like products of different unbound structural layers (Sormunen et al. Citation2016).
Table 1. The proportions of different MSWI BA mineral fractions (%) in the base, sub-base and filtration layers (Sormunen et al. Citation2016).
The samples collected during the treatment process in 2013 were combined in the laboratory according to the proportions given in for each BA structural layer product. The laboratory leaching tests (I) conducted on these combined BA samples are briefly summarised in Section 2.2, but a more detailed description on the test arrangements is given in Sormunen and Rantsi (Citation2015) and Sormunen et al. (Citation2016). The structures and sampling procedure of the lysimeter (II) and the interim storage field (III) studies are given in Sections 2.3 and 2.4, respectively. The methodology for comparing the laboratory and field scale results (IV) is explained in Section 2.5.
2.2. Laboratory studies (I)
The standardised percolation test CEN/TS 14405 adopted by the Finnish Standard Association (SFS 2004) was used to characterise the leaching properties of potentially harmful substances from the composed samples of the three different BA aggregate-like products used in the unbound structural layers (i.e. filtration, sub-base, base) of the interim storage field (Sormunen and Rantsi Citation2015; Sormunen et al. Citation2016). The laboratory leaching tests were conducted up to LS 10 (L kg−1), but only the results up to LS 1.5 (L kg−1) were used in this study for comparison since the maximum LS (L kg−1) obtained in the field was not more than 1.6 during the tests (in the lysimeter study). The number of samples used in this study for each material in the laboratory leaching tests was 5 (total n = 15).
The standardised pH-static leaching test CEN/TS 14997 (SFS Citation2007) was used to evaluate the pH dependency of the leaching of different substances from the MSWI BA mineral fractions. The pH-static leaching tests were performed for each initial BA mineral fraction (i.e. 0–2, 2–5, 5–12 and 12–50 mm) samples separately, and not with the composed BA mixture samples as in the percolation tests. This situation was not ideal, but was necessary due to practical reasons (i.e. the pH-static leaching tests were conducted before the final design of aggregate-like products from the BA minerals was completed).
Finally, the leaching test filtrates were analysed for different potentially harmful substances (metals only) with the following equipment:
Cold Vapour Atomic Fluorescence Spectroscopy (CVAFS): mercury.
Inductively Coupled Plasma Mass Spectrometer (ICP-MS): arsenic, barium, cadmium, cobalt, chromium, copper, molybdenum, nickel, lead, antimony, selenium, vanadium and zinc.
Only metals were chosen since in most cases they have been critical in utilising MSWI BA, and their leaching can be very much pH-dependent [e.g. Cornelis, Van Gerven, and Vandecasteele Citation2012; Hjelmar, Holm, and Crillesen Citation2007; Izquierdo et al. Citation2001). The measurement uncertainty of the leachate analyses varied from ±10–30%, depending on the substance and the sample. The pH, the electrical conductivity (EC) and the dissolved organic carbon (DOC) of each filtrate were also analysed. Regarding the total measurement uncertainty of the two laboratory leaching tests used in this study, it should be mentioned that the repeatability of the tests is always dependent on the material and substances of interest. The general repeatability of such tests is estimated to be approximately ±30% (Geurts et al. Citation2016).
2.3. Lysimeter study (II)
Three identical lysimeters (n = 3) were constructed with the MSWI BA aggregate-like products in Oulu, which is located by the Gulf of Bothnia, approximately 600 km north off Helsinki. The lysimeter construction took place in the beginning of August 2014.
An example of lysimeter structure is illustrated in . The diameter of each lysimeter was 800 mm and the height was 860 mm. The plastic lysimeters (PEH = Polyethylene, high density) were first excavated into the ground. Then a layer of washed natural gravel (#6–16 mm) and a filter fabric was placed on the bottom of each lysimeter. This was done to prevent MSWI BA particles from flushing into the water containers, and also to facilitate water drainage (). The MSWI BA aggregate-like products were mixed in the laboratory according to the proportions given in , and they were compacted layer by layer into each lysimeter. The total amount of MSWI BA minerals in each lysimeter was 0.294 tonnes (bulk density 0.735 t/m3).
The water containers of each lysimeter were emptied at least every week, and the amount of leachate (litres) was measured and recorded. A subsample of the leachate was taken from each sampling container at every sampling action and stored in a freezer (−20 °C) for further analysis. The subsamples of each lysimeter were composed every four months into combined leachate samples, which were analysed similarly to the laboratory test filtrates (see Section 2.2). At the time of writing, the lysimeter study is an ongoing project, but this paper only reports the results from a sampling period between the 1st of August, 2014, and the 31st of August, 2016 (i.e. 25 months). The number of analysed samples during this sampling period was 6 for each lysimeter (total n = 18).
Finally, for water balance calculations from the lysimeter site, linearly extrapolated precipitation data were used. This data were provided by the FMI [Finnish Meteorological Institute].
2.4. Interim storage field (III)
The ADR-treated MSWI BA aggregate-like products () were used in the unbound structural layers (i.e. filtration, sub-base and base) of the interim storage field, which was constructed inside a waste treatment centre in the summer of 2014. The waste treatment centre was located in Ilmajoki in the western part of Finland.
A principal cross-section of the field structure is illustrated in . The size of the field is approximately 9 900 m2. The subsoil underneath the field varied from solid rock to stiff moraine. Crushed concrete/brick/glass was used as an embankment fill. An additional base layer (300 mm) from natural rock aggregate was placed on top of the MSWI BA layers to fulfil the bearing capacity requirements given for the field (Sormunen et al. Citation2016).
Figure 3. A cross-section of the structural layers of the interim storage field, in which ADR-recovered MSWI BA aggregates were used (scale enlarged for clarity).
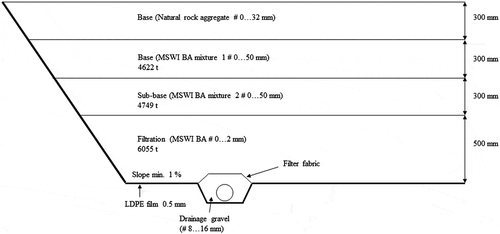
An LDPE (low-density polyethylene) film (0.5 mm) was placed below the structural layers () to steer the leachate water into the subsurface drainage. An LDPE film was also placed on the field edges to minimise water flow from the edges into the structure. Fine crushed aggregate (#0–2 mm) was used as backfill material between the embankment and the LDPE film to prevent the formation of holes in the film. The groundwater level varies seasonally, but it always remains at a minimum of 0.8 m beneath the LDPE film.
The leachate samples were taken from two sampling wells, which collected the subsurface leachate from the whole field. The sampling wells were located along the edge of the western part of the field. The leachate sampling period started on the 18th of September, 2014, after the structural layers illustrated in were constructed and finished on the 6th of May, 2015, when a bitumen course (110 mm) was constructed on top of the field. The leachate sampling period was therefore 7.5 months altogether. In total, 14 water samples were taken from both wells during the whole sampling period (i.e. total n = 28). Samples were taken more often in the beginning of the sampling period, and after that, they were taken based on the cumulative amount of leachate at approximately every 300 m3. The leachate pH and temperature were measured with a calibrated handheld device (Oakton Instruments, Waterproof Double Junction pHTestr® 30) in the field during each sampling.
Directly after sampling, the individual water samples (altogether n = 28) were stored in a cool place (4 °C). Prior to analysis, the samples were filtrated with a 0.45 μm sieve and analysed in the laboratory, as described in Section 2.2. For some substances, such as DOC, only 12 samples were analysed due to practical reasons. For the sake of clarity, it is worth noting that sampling in the interim storage field differs from that of laboratory and lysimeter studies since they are point measurements, whereas the analysed samples from the lysimeter and the laboratory studies are combined samples collected within a certain sampling period. This was because in the interim storage field, it was not practically possible to collect all the leachate that drained during the sampling period.
In addition, it was not possible to instal continuous water metres that would measure the amount of leachate. This was because low drainage and the narrow structure of drainage pipes would not allow the installation of such water metres inside the pipes. Therefore, the amount of leachate was measured once every weekday with a bucket (5 L). These measurements were then used to estimate the daily amount of leachate in each well, along with the cumulative amount of leachate during the whole sampling period within the whole field area. The linearly extrapolated precipitation data obtained from the FMI were used for the water balance calculations of the interim storage field site.
2.5. Comparison of laboratory and field scale studies (IV)
According to Hjelmar, Holm, and Crillesen (Citation2007), the L S−1 (L kg−1) scale can be converted to a time-scale for a given physical scenario under known conditions, when at a given time, the amount of water that has been in contact with a certain amount of material is known. The L S−1 (L kg−1) approach was chosen for the current study since it allowed for rough comparison of the data from the laboratory leaching tests between the large-scale lysimeter and field scale studies. Other methods, such as geochemical modelling, were not considered suitable for this research due to the limited amount of data collected during the field tests.
The interim storage field and the lysimeters were built with three different types of structural layers in which the proportions and therefore the leaching properties of the MSWI BA mixtures varied. For this reason, a weighted average was calculated for the laboratory leaching test results based on the amount (total dry weight) of different BA mixtures used in the interim storage field. The total dry weights (t) of each MSWI BA material used in the interim storage field are given in . For each scale studied, the L S−1 (L kg−1) was then calculated for each sampling point (interim storage field) or sampling period (lysimeter and laboratory test) and finally plotted with the concentrations (mg L−1 or μg L−1) of different substances analysed in the study.
3. Results and discussion
3.1. Water balance of field test sites
presents the leachate balance results for both field test sites: the lysimeter site in Oulu and the interim storage field site in Ilmajoki. As seen in , the total accumulated precipitation during the sampling periods was 1540 mm for the lysimeter and 350 mm for the interim storage field site. Based on the collected leachate amounts and areas exposed to rain, an average of 64 and 60% of precipitation during the sampling periods (lysimeter: 25 months and interim storage field: 7.5 months) was collected as leachate in the lysimeter and the interim storage field sites, respectively.
Table 2. Leachate balance for the two field test sites.
When interpreting the leachate balance results, it is necessary to emphasise that the measurements for leachate were obtained with manual measurements that were taken once every weekday in the interim storage field site. Therefore, it is possible that the actual amount of leachate in the interim storage field during the sampling period may have been somewhat different from what was obtained with this rough measuring technique. This may have also had influence on the L S−1 (L kg−1) calculations presented in the following chapter.
3.2. Leachate quality compared between three study scales
3.2.1. L S−1 (L kg−1) and the pH
summarises the L S−1 ratios (L kg−1) and concentrations of the analysed substances at the beginning and at the end of the laboratory and both field tests. At the end of the sampling period, the achieved L S−1 ratios (L kg−1) were 1.6 and 0.13 for the lysimeter and interim storage field site, respectively (). In the laboratory percolation test, an L S−1 (L kg−1) of up to 1.5 was taken for comparison even though the test was continued up to an L S−1 (L kg−1) of 10. This was because such an L S−1 (L kg−1) was too high to be compared with the L S−1 (L kg−1) obtained in the field studies within the given sampling period. As mentioned in chapter 2.3, the lysimeter study is still ongoing, and more data will be collected in order to make comparisons between laboratory and lysimeter studies with higher L S−1 (L kg−1) in the future.
Table 3. Concentrations of analysed substances in leachates obtained from the laboratory, lysimeter and interim storage field studies at the beginning and at the end of each test.
The leachate pH varied from 9.9 to 12.0 in the laboratory, whereas in the field studies, the leachate pH varied from 8.0–11.5 and 7.1–10.0 in the interim storage field and lysimeter studies, respectively (). Similar differences in the pH values between field and laboratory experiments have been observed in the field studies of conventionally treated MSWI BA by other researchers as well (e.g. Dabo et al. Citation2009; Hjelmar, Holm, and Crillesen Citation2007; Izquierdo et al. Citation2008 and de Windt et al. Citation2011). According to Dabo et al. (Citation2009), pH evolution in MSWI BA field studies can be dependent on the hydrodynamics of rainwater infiltration or carbonation processes, but to a lesser extent on seasonal variation of temperature as well. The preferential flow pathways may also cause lower pH values in the field conditions (Hjelmar, Holm, and Crillesen Citation2007). Due to the limited number of samples, the results obtained in this study did not allow for the verification of possible causes for lower pH values in the field. It is, however, suspected that one of the main reasons is the different level of carbonation for MSWI BA between field and laboratory test results, which was also noticed by, for example, Arickx, Van Gerven, and Vandecasteele (Citation2006) and Todorovic and Ecke (Citation2006). Laboratory column tests are performed in water-saturated conditions, whereas in field and lysimeter studies, the material is also exposed to atmospheric air. Therefore, carbonation reactions in these set-ups may essentially be faster.
3.2.2. Concentrations of potentially harmful substances
The concentrations of potentially harmful substances analysed from the leachate samples mostly decreased during the sampling period in both field test sites as summarised in . The only exception was the concentration of vanadium (V), which increased at the beginning in the interim storage field site, but not in the lysimeter or the laboratory study (). In most cases, the concentrations of substances were higher in the interim storage field than in the lysimeter and laboratory tests (). This is most likely due to differences in sampling between the interim storage field and the lysimeter and laboratory studies (i.e. point measurements vs. combined samples within a certain sampling period, as explained in Section 2.4). In subsequent paragraphs, results of the most critical substances of ADR-recovered MSWI BA in respect to their utilisation potential in Finland (Sormunen and Rantsi Citation2015 and Sormunen et al. Citation2016) are compared with the laboratory test results and are discussed in more detail.
3.2.2.1. Chloride and sulphate
(a)–(g) illustrates the concentrations of salts (chloride [Cl−] and sulphate []) and certain metals (antimony [Sb], copper [Cu], chromium [Cr], molybdenum [Mo] and vanadium [V]) in relation to the L S−1 (L kg−1) calculated for both field test sites and the percolation tests in the laboratory.
Figure 5. Average concentrations of (a) chloride (Cl−, mg L−1), (b) sulphate (, mg L−1), (c) chromium (Cr, μg L−1), (d) copper (Cu, μg L−1), (e) molybdenum (Mo, μg L−1), (f) antimony (Sb, μg L−1) and (g) vanadium (V, μg L−1) as a function of Liquid to Solid ratio (L S−1 (L kg−1)) in the three test scales (Interim storage field (n = 2), lysimeter (n = 3), and laboratory).
Note: Error bar shows the standard deviation.
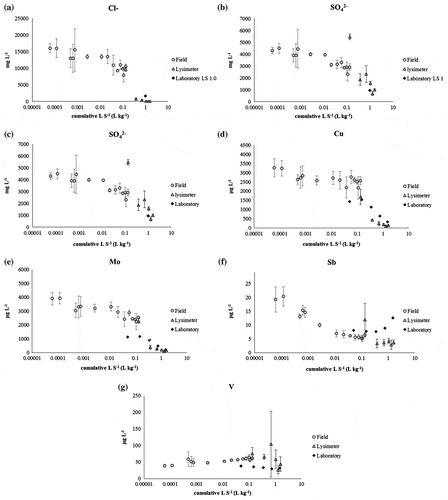
A reasonable agreement was observed for the leaching behaviour of Cl− and in the lysimeter and laboratory experiments ((a) and (b)). This is in accordance with the findings of Hjelmar, Holm, and Crillesen (Citation2007), who investigated the leaching of conventionally treated BA in a field scale. For the interim storage field, it was not possible to fully compare the obtained results with the laboratory results since the obtained L S−1 (L kg−1) at the end of the sampling period was less in the field (0.13) than it was in the laboratory (1.0). Nevertheless, the trends for Cl− and
concentrations are decreasing in the interim storage field ((a) and (b)). Considering the soluble nature of these substances, it is very likely that their concentrations would have decreased further if the water percolation had continued in a similar manner and no bitumen course had been constructed on top. When considering the potential field application scenarios of MSWI BA, the utilisation of this waste-derived material in unbound structural layers would in any case require a bitumen cover on top of the structures. This is not only due to possible environmental concerns, but also to the technical properties of this material, as it is known that MSWI BA particles are prone to crushing, and an additional base layer of natural aggregate or a thicker bitumen layer is required to ensure the durability of such road or field structures (Arm Citation2003; Bendz et al. Citation2006; Sormunen and Rantsi Citation2015) This would therefore also decrease the leaching of soluble salts as the materials’ contact with water is decreased.
3.2.2.2. Metals (Mo, Sb, Cr, Cu and V)
Leaching behaviour observed for molybdenum, antimony, chromium and copper in the laboratory and field scale studies were similar regardless of the differences in concentration levels of different substances between these different study scales ((c)–(f)). Conversely, the leaching behaviour of vanadium in the interim storage field was somewhat different from that of lysimeter and laboratory studies: it increased in the interim storage field at the beginning, whereas it decreased in the other two studies ((g)). These findings cannot be compared with literature data since many of the previous MSWI BA field studies did not include vanadium in their analyses (e.g. Bruder-Hubscher et al. Citation2001; Dabo et al. Citation2009; Hjelmar, Holm, and Crillesen Citation2007; Lidelöw and Lagerkvist Citation2007 and de Windt et al. Citation2011). Vanadium, however, is considered to be the second most abundant trace oxyanion in MSWI BA after antimony (Chandler et al. Citation1997), and only recently it has come into focus among researchers. For example, in a MSWI BA field scale study in Italy, Izquierdo et al. Citation2008 reported that the leaching of vanadium followed a leaching trend similar to highly soluble salts in the field: the most soluble fraction of vanadium was depleted in the initial leaching stages. It is possible, therefore, that the leaching of vanadium would have decreased in the interim storage field when the L S−1 (L kg−1) had increased, and thus would have started to follow the leaching behaviour of vanadium that was obtained in the lysimeter and laboratory studies. It is suggested that the mobility of vanadium under field conditions may be enhanced at the beginning, but the leached concentrations are at a low level and still below the stringent limit values such as those in Germany (e.g. for road base layer < 150 μg L−1) (BMUB Citation2015).
DOC has also been shown to affect the leaching of, for example, copper from MSWI BA (e.g. Arickx, Van Gerven, and Vandecasteele Citation2006 and Hyks, Astrup, and Christensen Citation2009). In this study, only a moderate relationship between copper concentration (μg L−1) and DOC (mg L−1) was observed ((a)). Conversely, the relationship between DOC (mg L−1) and molybdenum (μg L−1) was more prominent: the molybdenum concentration clearly increased with increasing DOC ((b)). To the authors’ best knowledge, such a relationship between molybdenum concentration and DOC has not yet been reported in the literature for MSWI BA.
Figure 6. Concentrations of (a) copper (Cu, μg L−1) and (b) molybdenum (Mo, μg L−1) plotted against the dissolved organic carbon (DOC, mg L−1) measured in the interim storage field.
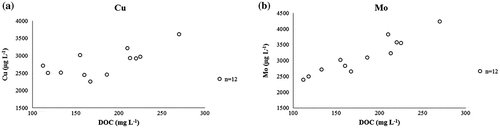
According to van der Sloot, Kosson, and Hjelmar (Citation2001), molybdenum leaches as molybdate () and its mobile fraction is nearly leached out within L S = 2 L kg−1. This was observed for molybdenum in this study and was illustrated in (e). Molybdenum also shows pH dependency characteristics: at pH 8 and 10, the leaching of molybdenum increases (Dijkstra, van der Sloot, and Comans Citation2006). Similar observations were made for molybdenum leaching in the current field study as well (). According to Dijkstra, van der Sloot, and Comans (Citation2006), a possible reason for this increased leaching is that at those pH levels, the adsorption of anions (
) seems to be absent. Overall, it seems that the leaching of molybdenum from MSWI BA is the cause of many factors. The results obtained in this study indicated that one of these reasons can be the complexation with DOC. This should, however, be confirmed with larger data sets in future studies.
Figure 7. Concentration of molybdenum (Mo, μg L−1) plotted against the measured pH in the interim storage field.
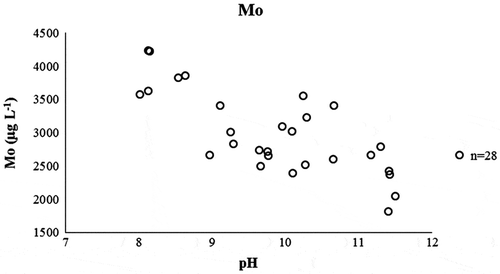
A more detailed observation of the leaching behaviour of antimony in the field revealed that the behaviour of antimony leaching was strongly dependent on the development of leachate pH during the test period. illustrates the concentration of antimony (Sb, μg L−1) as a function of the measured daily amount of leachate (m3 d−1) and shows the leachate pH as a function of cumulative L S (L kg −1) measured in the field. From these figures, it can be clearly seen that the concentration of antimony decreased when the amount of leachate increased (). This decrease in leachate amount also caused an increase in pH in the field experiment ().
Figure 8. Concentration of antimony (Sb, μg L−1) plotted against the daily amount of leachate (m3 d−1) measured in the interim storage field.
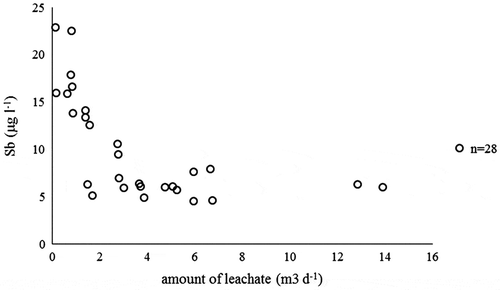
Figure 9. Leachate pH plotted against the cumulative LS−1 (L kg−1) measured in the interim storage field.
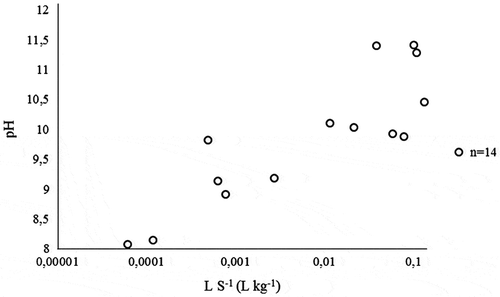
When observing this relationship of pH and antimony in more detail, the concentration of antimony was the highest in the interim storage field when the pH value was 8 ((a)). The same phenomenon was noticed with the pH-static leaching tests that were performed for the individual MSWI BA mineral fractions (0–2, 2–5, 5–12 and 12–50 mm) in the laboratory, as illustrated with the calculated weighted average of the four mineral fractions in (b). The same pH-dependent behaviour was observed for antimony in a recent article by Keulen et al. (Citation2016). Antimony is present in MSWI BA as oxyanion in the oxidation states +3 and +5. Thus, the decline of the Sb concentrations in the field experiment ((f)) can be explained with the increase in pH from approximately 8 to above 10 (). Conversely, the leaching of antimony is not only controlled by the pH but also by the formation of sparingly soluble antimonites (Cornelis, Van Gerven, and Vandecasteele Citation2006, 2012). A relationship between calcium and antimony concentration was first reported by Johnson et al. (Citation1999). This was explained by the formation of calcium antimonates like Ca(Sb(OH)6)2 with low solubility (Dijkstra, van der Sloot, and Comans Citation2006; Johnson et al. Citation1999, 2005). Thus, lower calcium concentrations after the first flush led to the increase in observed antimony concentrations, as observed in the laboratory and the lysimeter experiments.
Figure 10. The concentration of antimony (Sb, μg L−1) plotted against the leachate pH measured in the interim storage field (a), and the calculated weighted average of antimony concentration (mg L−1) at different pH values (b) according to the pH-static leaching test conducted in the laboratory (LS−1 10 L kg−1) for each separate mineral fraction.
Note: Error bar depicts the estimated measurement uncertainty of the laboratory analysis.
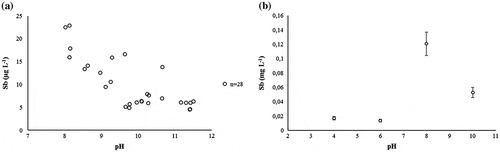
3.3. Uncertainty
As pointed out in the introduction, the leaching properties of waste-derived aggregates can vary depending on the study scale and the respective hydrodynamic conditions in which they are investigated. Hence, the comparison of test results obtained from different study scales is of the utmost importance in order to fully understand the leaching behaviour of potentially harmful substances in the reuse scenarios of waste-derived aggregates such as MSWI BA. However, in many cases, the comparison of different study scales is not that straightforward, especially if a limited number of data are available. This in turn causes different types of uncertainties that can hamper the result interpretation.
In this study, many uncertainties and trade-offs between the data and the chosen methodologies existed. For example, the comparison of different study scales and the leaching behaviour of potentially harmful substances were made based on L S−1 (L kg−1). Other methods, such as geochemical modelling, might have provided a more detailed analysis. However, this modelling could not be used in this study since the amount of data was not sufficient enough to perform such modelling. In fact, many large-scale studies often have to deal with limited amount of funding, which in turn limits the application of various methods that could be better, but that require more data that can actually be collected.
In addition, the set-up of study scales varied slightly, because the laboratory leaching tests were not conducted in a stratified structure, as was the case for both field scale studies. This was resolved by calculating the weighted average from the laboratory leaching test results based on the actual amount of materials used in the interim storage field. It is clear that the leaching of potentially harmful substances can vary depending on the grain size of different MSWI BA minerals. On the other hand, the field structure was a mixture of all different grain sizes of MSWI BA minerals, which in turn justifies the use of such a weighted average when the exact same structure was not used in all study scales.
Finally, the amount of leachate in the interim storage field study was obtained with a rough measurement technique that can cause some uncertainty in the L S−1 (L kg−1) calculations of the interim storage field. It is clear that this was not the most ideal set-up, but unfortunately, it was unavoidable, as the constant water metres could not be installed due to technical issues (see Section 2.4). In many large-scale field studies, such technical issues can be problematic. However, these problems should not prevent on the conducting and reporting of results of such large-scale studies, since at the end such studies provide valuable information about the leaching properties of waste-derived aggregates in more realistic conditions. For example, the results of this study showed that despite of uncertainties, the consistency in leaching behaviour of most substances in ADR-recovered MSWI BA was evident between the laboratory and field scale studies. However, it should be noted that even though ADR technology enhances the recovery of NF metals, the leaching of certain substances such as chloride and antimony still exists. In practice, this means that ADR technology can improve the environmental compatibility of MSWI BA minerals to some extent, but not entirely. Therefore, further technical improvements are required in case the leaching of these substances from MSWI BA needs to be decreased in the future.
3.4. National impact
In Finland, crushed concrete and ashes from coal, wood and pear burn facilities may have been utilised with a simple notification procedure under the Finnish Decree concerning the recovery of these materials in earth construction (Government Decree 591/2006, modifications 403/2009, and 1825/2009, Citation2006). For many other materials, such as recovered MSWI BA, a time-consuming environmental permit has been required. This has recently changed, since the previously mentioned national Decree was under renewal and a new Decree was set in to force on the 1st of January 2018. In this renewed Decree, several other waste materials have been added within the scope of application. One of these materials is recovered MSWI BA, which would not have been added in the Decree, unless data on materials’ leaching properties existed. However, the laboratory leaching test results, such as those presented by Sormunen and Rantsi (Citation2015) were not enough, since the authorities demanded field scale studies as well. As a result, this study was conducted, and the results presented in this paper provided important background data for decisionmakers when assessing the acceptance of recovered MSWI BA within this aforementioned Decree. Of course, it is clear that this study did not take a stand on the accepted risk levels for different potentially hazardous substances (i.e. the limit values). This is an issue that needs to be decided nationally as has been done, for example, in Germany (BMUB Citation2015) and in France (Sétra (Service d’études sur les transports, les routes et leurs aménagements) Citation2012).
4. Conclusions
This study aimed to investigate the leaching properties of ADR-recovered MSWI BA in the field in order to better understand its environmental impact in actual civil engineering structures. This particular material was chosen since its leaching properties have not yet been studied, especially with larger field scale studies. The obtained field scale test results were also complemented with previously conducted laboratory leaching test results. The comparison of leaching behaviour of potentially hazardous substances between the field and laboratory scale tests results was based on the L S−1 (L kg−1). Despite some uncertainties related to, for example, the measurements of leachate in the field and the slightly different set-up of laboratory and field scale studies, the following conclusions can be made from this research:
The leaching behaviours of potentially harmful substances, such as Cl−.
, Cr, Cu, Mo and Sb showed obvious consistency, both in the field studies and in the laboratory experiments with the recovered MSWI BA. The concentrations of most of these substances decreased both in the laboratory and in the field case studies over the course of the experiments.
The only exception was vanadium, the concentration of which increased in the field at the beginning, even though the conducted laboratory experiments showed decreased concentrations of vanadium when the L S−1 (L kg−1) increased. This suggested that the mobility of vanadium under field conditions may be enhanced at the beginning, but the leached concentrations are rather low and still below the stringent limit values, such as those in Germany (e.g. for road base layer <150 μg L−1).
Even though the ADR technology enhances the recovery of NF metals, the leaching of certain substances such as chloride and antimony still exists. In practice, this means that ADR technology can improve the environmental compatibility of MSWI BA minerals to some extent, but not entirely. Therefore, further technical improvements are required in case the leaching of these substances from MSWI BA needs to be decreased in the future.
Finally, the Finnish Government Decree concerning the recovery of certain wastes in earth construction (591/2006, modifications 403/2009 and 1825/2009, Citation2006) was under renewal, and a new Decree was set in to force on the 1st of January 2018. In this new Decree, the limit values have been recalculated and new waste materials have been added within the scope of application of this Decree. One of these new materials is recovered MSWI BA, which would not have been accepted in this Decree unless laboratory and field scale leaching data on this material existed. Therefore, the results of this study provided important background information for decisionmakers in order to nationally facilitate the use of this wastederived aggregate in civil engineering in the future.
Geolocation information
Ilmajoki and Oulu, Finland.
Disclosure statement
No potential conflict of interest was reported by the authors.
Funding
This work was supported by the Tekes [grant number 1105/2013/2013].
Notes on contributors
Laura Annika Sormunen is a recently graduated PhD at Tampere University of Technology. The title of her PhD is “Recovered municipal solid waste incineration bottom ash: Aggregate like products for civil engineering structures”. Currently, she is also working as an R&D Manager at a private company Suomen Erityisjäte Oy which treats, for example, municipal solid waste incineration bottom ashes in Finland.
Tommi Kaartinen (MSc (Tech)) is a senior scientist at VTT. He is mainly in research, development and consultation projects regarding waste treatment, valorisation and final disposal, environmental impacts, waste characterization and water treatment.
Riina Rantsi (PhD) is a business development manager at Suomen Erityisjäte Oy. She has initiated and been involved with many research projects within the company, including this one with the investigation of MSWI BAs.
Acknowledgements
The investigations were carried out within a joint research project. The authors would like to thank the steering group of the research programme for their valuable comments and suggestions throughout the investigations. The assistance of the Lakeuden Etappi staff in the field is also very much appreciated.
References
- Åberg, A., J. Kumpiene, and H. Ecke. 2006. “Evaluation and Prediction of Emissions from a Road Built with Bottom Ash from Municipal Solid Waste Incineration (MSWI).” Science of the Total Environment 355 (1–3): 1–12. doi:10.1016/j.scitotenv.2005.03.007.
- Arickx, S., T. Van Gerven, and C. Vandecasteele. 2006. “Accelerated Carbonation for Treatment of MSWI Bottom Ash.” Journal of Hazardous Materials 137 (1): 235–243. doi:10.1016/j.hazmat.2006.01.059.
- Arm, Maria. 2003. Mechanical Properties of Residues as Unbound Road Materials – Experimental Test on MSWI Bottom Ash, Crushed Concrete and Blast Furnace Slag. PhD diss., KTH Land and Water Resources Engineering, Stockholm.
- Bendz, D., M. Arm, P. Flyhammar, G. Westberg, K. Sjostrand, M. Lyth, and O. Wik. 2006. Projekt Vandora: En studie av langtisdegenskaper hos en vag anlagd med bottenaska fran avfallsborbranning [Project Vandora: A Study on the Long-term Properties of a Road Built with MSWI BA]. Stockholm: Varmefors Service AB.
- BMUB. 2015. Arbeitsentwurf der Mantelverordnung: Verordnung zur Festlegung von Anforderungen für das Einbringen oder das Einleiten von Stoffen in das Grundwasser, an den Einbau von Ersatzstoffen und für die Verwendung von Boden und bodenähnlichem Material [Working Draft of the Mantelverordnung: Ordinance Laying down Requirements for the Introduction into or Discharge of Substances into Groundwater, the Installation of Substitutes and the use of Soil and Soil-like Material]. July 23.
- Bruder-Hubscher, V., F. Lagarde, M. J. F. Leroy, C. Coughanowr, and F. Enguehard. 2001. “Utilisation of Bottom Ash in Road Construction: Evaluation of the Environmental Impact.” Waste Management & Research 19 (6): 545–556.10.1177/0734242X0101900611
- CEWEP (Confederation of European Waste-to-Energy Plants). 2016. “Bottom Ash Fact Sheet.” Accessed October 21, 2016. http://www.cewep.eu/news/m_1485
- Chandler, A., T. Eighmy, O. Hjelmar, D. Kosson, S. Sawell, J. Vehlow, J. H. van der Sloot, and J. Hartlén. 1997. Municipal Solid Waste Incinerator Residues, Studies in Environmental Science 67. Amsterdam: Elsevier Science B. V.
- Cornelis, G., T. Van Gerven, and C. Vandecasteele. 2006. “Antimony Leaching from Uncarbonated and Carbonated MSWI Bottom Ash.” Journal of Hazardous Materials 137 (3): 1284–1292. doi:10.1016/j.hazmat.2006.04.048.
- Cornelis, G., T. Van Gerven, and C. Vandecasteele. 2012. “Antimony Leaching from MSWI Bottom Ash: Modelling of the Effect of PH and Carbonation.” Waste Management 32 (2): 278–286. doi:10.1016/j.wasman.2011.09.018.
- Dabo, D., R. Badreddine, L. de Windt, and I. Drouadaine. 2009. “Ten-year Chemical Evolution of Leachate and Municipal Solid Waste Incineration Bottom Ash Used in a Test Road Site.” Journal of Hazardous Materials 172 (2–3): 904–913. doi:10.1016/j.hazmat.2009.07.083.
- Dijkstra, J. J., H. van der Sloot, and R. N. J. Comans. 2006. “The Leaching of Major and Trace Elements from MSWI Bottom Ash as a Function of PH and Time.” Applied Geochemistry 21 (2): 335–351. doi:10.1016/j.apgeochem.2005.11.003.
- DIN 19528. 2009. Leaching of Solid Materials – Percolation Method for the Joint Examination of the Leaching Behaviour of Organic and Inorganic Substances for Materials with a Particle Size Up to 32 mm – Basic Characterization using a Comprehensive Column Test and Compliance Test using a Quick Column Test. Berlin: German Standardisation Organisation.
- European Commission. 2006. “Integrated Pollution Prevention and Control, Reference Document on the Best Available Techniques for Waste Incineration.” Accessed October 25, 2016. http://eippcb.jrc.ec.europa.eu/reference/BREF/wi_bref_0806.pdf
- European Commission. 2015. “Closing the Loop: Commission Adopts Ambitious New Circular Economy Package to Boost Competitiveness, Create Jobs and Generate Sustainable Growth.” Press release database, December 2. http://europa.eu/rapid/press-release_IP-15-6203_en.htm.
- Flyhammar, P., and D. Bendz. 2006. “Leaching of Different Elements from Subbase Layers of Alternative Aggregates in Pavement Construction.” Journal of Hazardous Materials 137 (1): 603–611. doi:10.1016/j.hazmat.2006.02.072.
- François, D., and K. Pierson. 2009. “Environmental Assessment of Road Site Built with MSWI Residue.” Science of the Total Environment 407 (23): 5949–5960. doi:10.1016/j.scitotenv.2009.08.007.
- Geurts, R., J. Spooren, M. Quaghebeur, K. Broos, C. Kenis, and L. Debaene. 2016. “Round Robin Testing of a Percolation Column Leaching Procedure.” Waste Management 55: 31–37. doi:10.1016/j.wasman.2016.06.010.
- Government Decree 591/2006 (modifications 403/2009 & 1825/2009). 2006. Recovery of Certain Wastes in Earth Construction in Finland. Accessed October 26, 2016. https://www.finlex.fi/en/laki/kaannokset/2006/en20060591.pdf
- Hjelmar, O., J. Holm, and K. Crillesen. 2007. “Utilisation of MSWI Bottom Ash as Sub-base in Road Construction: First Results from a Large-Scale Test Site.” Journal of Hazardous Materials 139 (3): 471–480. doi:10.1016/j.hazmat.2006.02.059.
- Hyks, J., T. Astrup, and T. H. Christensen. 2009. “Influence of Operational Conditions, Waste Input and Ageing in Contaminant Leaching from Waste Incineration Bottom Ash: A Full Scale Study.” Chemosphere 76 (9): 1178–1184. doi:10.1016/j.chemosphere.2009.06.040.
- ISO/TS 21268-3. 2007. Soil Quality – Leaching Procedures for Subsequent Chemical and Ecotoxicological Testing of Soil and Soil Materials – Part 3: Up-Flow Percolation Test. Berlin: The International Organization for Standardization.
- Izquierdo M., E. Vazquez, X. Querol, M. Barra, Á. López, and F. Plana. 2001. “Use of Bottom Ash from Municipal Solid Waste Incineration as a Road Material.” Paper 37 presented at International Ash Utilization Symposium, Center for Applied Energy Research, University of Kentucky, Lexington.
- Izquierdo, M., X. Querol, A. Josa, A. E. Vazquez, and A. López-Soler. 2008. “Comparison between Laboratory and Field Leachability of MSWI Bottom Ash as a Road Material.” Science of the Total Environment 389 (1): 10–19. doi:10.1016/j.scitotenv.2007.08.020.
- Johnson, A. C., M. Kaepeli, S. Brandenberger, A. Ulrich, and W. Baumann. 1999. “Hydrological and Geochemical Factors Affecting Leachate Composition in Municipal Solid Waste Incinerator Bottom Ash. Part II. The Geochemistry of Leachate from Landfill Lostorf, Switzerland.” Journal of Contaminant Hydrology 40 (3): 239–259. doi:10.1016/S0169-7722(99)00052-2.
- Johnson, C. A., H. Moench, H. P. Wersin, P. P. Kugler, and C. Wenger. 2005. “Solubility of Antimony and Other Elements in Samples Taken from Shooting Ranges.” Journal of Environmental Quality 34 (1): 248–254.
- Keulen, A., A. van Zomeren, P. Harpe, W. Aarnink, W. H. A. Simons, and H. J. Brouwers. 2016. “High Performance of Treated and Washed MSWI Bottom Ash Granulates as Natural Aggregate Replacement within Earth-Moist Concrete.” Waste Management 49: 83–95. doi:10.1016/j.wasman.2016.01.010.
- Lidelöw, S., and A. Lagerkvist. 2007. “Evaluation of Leachate Emissions from Crushed Rock and Municipal Solid Waste Incineration Bottom Ash Used in Road Construction.” Waste Management 27 (10): 1356–1365. doi:10.1016/j.wasman.2006.07.021.
- Prezzi, M., P. Bandini, J. A. H. Carraro, and P. J. M. Monteiro. 2011. “Editorial: Use of Recyclable Materials in Sustainable Civil Engineering Applications.” Advances in Civil Engineering 2011: 1–2. doi:10.1155/2011/896016.
- RTS (Rakennustietosäätiö) 2010. InfraRYL: Infrarakentamisen yleiset laatuvaatimukset, Osa I Väylät ja alueet [General Quality Criteria for Infrastructure Construction, Part I Routes and Areas]. Helsinki: Rakennustieto Oy.
- Schreurs, J. P. G. M., H. van der Sloot, and C. Hendriks. 2000. “Verification of Laboratory-field Leaching Behaviour of Coal Fly Ash and MSWI Bottom Ash as a Road Base Material.” Waste Management 20 (2–3): 193–201. doi:10.1016/S0956-053X(99)00322-0.
- Sétra (Service d’études sur les transports, les routes et leurs aménagements) 2012. Methodological Guide. Acceptability of Alternative Materials in Road Construction, Environmental Assessment. Paris: Éditions Sétra.
- SFS. 2004. Characterization of Waste. Leaching Behaviour Tests. Up-flow percolation test (under specified conditions), CEN/TS 14405. Helsinki: Finnish Standards Association.
- SFS. 2007. Characterization of Waste. Leaching Behaviour Tests. Influence of pH on leaching with continuous pH control, CEN/TS 14997. Helsinki: Finnish Standards Association.
- van der Sloot, H., D. S. Kosson, O. Hjelmar. 2001. “Characteristics, Treatment and Utilization of Residues from Municipal Waste Incineration.” Waste Management 21 (8): 753–765. doi:10.1016/S0956-053X(01)00009-5.
- Sormunen, L. A., A. Kalliainen, A. P. Kolisoja, and P. R. Rantsi. 2016. “ Combining Mineral Fractions of Recovered MSWI Bottom Ash: Improvement for Utilization in Civil Engineering.” Waste Biomass and Valorization, 8 (5): 1467–1478. doi: 10.1007/s12649-016-9656-4.
- Sormunen, L. A., and R. Rantsi. 2015. “To Fractionate Municipal Solid Waste Incineration Bottom Ash: Key for Utilisation?” Waste Management & Research 33 (11): 995–1004. doi:10.1177/0734242X15600052.
- Todorovic, J., and H. Ecke. 2006. “Demobilisation of Critical Contaminants in Four Typical Waste-to-Energy Ashes by Carbonation.” Waste Management 26 (4): 430–441. doi:10.1016/j.wasman.2005.11.011.
- Vandecasteele, C. 2015. “Foreword in Book of Extended Abstracts of WASCON 2015 – Resource Efficiency in Construction Conference.” Santander, Spain, June 10–12.
- de Vries, W., and B. C. Rem. 2013. “ADR – A Classifier for Fine Moist Materials.” In Separating Pro-Environment Technologies for Waste Treatment, Soil and Sediments Remediation, edited by V. Gente and F. La Marca, 43–58. Sharjah: Bentham Science Publishers.
- de Windt, L., D. Dabo, S. Lidelöw, R. Badreddine, and A. Lagerkvist. 2011. “MSWI Bottom Ash Used as Basement at Two Pilot-Scale Roads: Comparison of Leachate Chemistry and Reactive Transport Modelling.” Waste Management 31 (2): 267–280. doi:10.1016/j.wasman.2010.06.002.