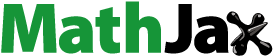
ABSTRACT
Here, a series of polyurethane porous hydrogels (PUF-s) loaded with different sodium carboxymethyl cellulose (CMC) were successfully prepared by one-step foaming method. The physio-chemical properties and morphologies were characterized. The effects of CMC content, adsorbent dosage, temperature, pH value and other factors on the adsorption of methylene blue (MB) dye in water by CMC-PUF-s were also investigated through static adsorption experiments. The results showed that CMC-PUF-10 had excellent adsorption performance for MB solution with removal rate of 81.47%, and the maximum adsorption capacity was 27.5 mg/g. In addition, the study of adsorption kinetics and adsorption isotherms showed that the adsorption of MB by CMC-PUF was more consistent with Langmuir isotherm adsorption model and pseudo second-order kinetic model. The adsorption thermodynamics study suggested that the adsorption process of MB by CMC-PUF-10 was spontaneous and exothermic at room temperature. The results of cyclic adsorption experiment demonstrated that the removal rate of MB reached above 70% after five cycles, indicating the foams with excellent recyclability. Finally, a low-cost, environmentally friendly and recyclable MB adsorbent was synthesized in this study. As polyurethane foam was synthesized by one-step foaming method, this adsorbent can be prepared on site in practical application and reduce the transportation cost.
Graphical Abstract
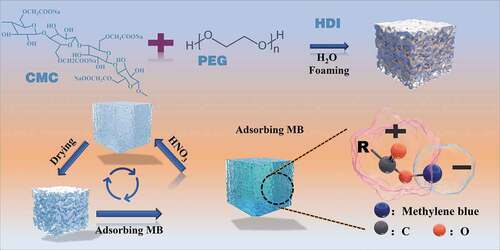
1. Introduction
With the rapid development of economy and industrial civilization, water contamination has become one of the most serious environmental issue that hazard people’s health and balance of nature [Citation1]. The accumulation of industrial production dyes in water is persistent in the natural environment and it is very difficult to eliminate these micropollutants [Citation2], which pose a great threat to the environment [Citation3]. Methylene blue is a typical cationic dye, which causes not only great harm to the environment but also harmful to the human body, such as nausea, shock, and potential cancer risk [Citation4]. This dye causes eye burns, breathing disorders, heart rate increases, shock, cyanosis, jaundice, quadriplegia, tissue necrosis, nausea, vomiting, mental confusion, painful micturition, and methemoglobinemia [Citation5,Citation6].
At present, the methods for removing these micropollutants are mainly divided into the following categories, including coagulation [Citation7,Citation8], chemical oxidation [Citation9], biodegradation [Citation10,Citation11], electrophoresis [Citation12], membrane filtration [Citation13] and adsorption [Citation14,Citation15]. Among these methods, adsorption method is considered as the simplest and most effective method to remove micropollutants due to its low cost, high efficiency, and simple operation [Citation15]. However, the disadvantages of low removal rate, easy to cause secondary pollution, or difficult to recover limited their applications and the conventional adsorbent is far from achieving the desired effect. Therefore, it is critical to develop new materials with high adsorption capacity, adapt to the environment, and could effectively remove micro pollutants from water.
The adsorption capacity of an adsorbent depends on the nature of the adsorbent (e.g. functional groups present, specific surface area, and pore size distribution), the nature of adsorbate (e.g. functional groups present, polarity, hydrophobicity, molecular weight and size, solubility, and pKa or pKb for weak acids or bases), and solution conditions (e.g. pH, temperature and adsorbate concentration, presence of competitive solutes, polarity of solvent) [Citation16]. In recent years, cellulose with its derivatives and composites character as natural bio-based materials have been widely used in the preparation of adsorbents due to their wide range of sources, environmental friendliness [Citation6,Citation17,Citation18]. Ali H. Jawad et al. introduced an alternative precursor for the production of activated carbon using dragon fruit (Hylocereus costaricensis) peel (DFP) [Citation19]. A.S. Abdulhameed used K2CO3 as a chemical activator to help pyrolysis, and converted grass chips (GW) into high-quality mesoporous activated carbon (GWAC) for effective removal of methylene blue from water [Citation20]. Sodium carboxymethyl cellulose (CMC) is an important cellulose derivative with a wide range of sources [Citation21]. Its rich carboxyl and hydroxyl groups in the molecule can form stable complexes with many metal ions; therefore, it could be used as a flocculant to treat wastewater containing heavy metal ions [Citation21,Citation22]. Furthermore, the carboxylic acid group in CMC could also generate strong electrostatic attraction with cationic dyes, and has the ability to adsorb cationic dyes [Citation23,Citation24]. Therefore, CMC can be used as an adsorbent to adsorb MB (cationic dyes). It can generate strong electrostatic attraction with MB by relying on its COO – to adsorb MB. Yashi and his colleagues prepared a biological adsorbent (CMC-PAA) consisting of PAA, PAM, and sodium carboxymethyl cellulose (CMC-PAMA) through simple thermal crosslinking. CMC with carboxymethyl and hydroxyl groups not only act as a substrate to react with other polymers but also provides active sites for adsorbing pollutants, CMC-PAA showed a significant adsorption level for MB (qm: 1611.4 mg/g) [Citation25]. Huie Liu and his colleagues prepared carboxymethyl cellulose/graphene composite aerogel beads (CMC/GAs) by the easy scaling-up method (i.e. wet spinning ambient pressure drying method). Under the experimental conditions explored, the maximum adsorption capacity of CMC/GAs-30 for MB is 222.72 mg/g [Citation26]. However, carboxymethyl cellulose is soluble in water with poor mechanical properties, it presents limited adsorption capacity for metal ions and dyes, and the adsorption rate is relatively slow [Citation27]. The separation process after adsorption is time-consuming, complicated, and incomplete, so the direct treatment of wastewater often cannot achieve satisfactory results [Citation28]. Polyurethane foam (PUF) is a type of polymer material with three-dimensional network crosslinking structure, which is obtained by polymerization of polyols and polyisocyanates. Due to its porous structure and high specific surface area, it is often used as adsorbent or carrier for wastewater treatment [Citation29,Citation30]. Using PUF as CMC carrier can not only make the adsorbent easy to separate from water and recover but also effectively improve the adsorption capacity of micro pollutants.
In this study, hexamethylene diisocyanate (HDI) and polyethylene glycol (PEG) were used as raw materials, and sodium carboxymethyl cellulose (CMC) in different proportions (based on the mass of PEG, 5%, 10%, and 15%, respectively) were added to them. A series of polyurethane porous hydrogel foams (CMC-PUF-s) loaded with CMC were prepared by gas foaming method (). The chemical structures CMC-PUF-s were characterized and observed by X-ray diffraction (XRD) and Fourier transform infrared (FTIR) spectrometer, the morphology and microstructure were characterized by scanning electron microscopy (SEM). Furthermore, the adsorption behavior of methylene blue of CMC-PUF-s in water was studied.
2. Experimental
2.1. Materials
Sodium carboxymethyl cellulose (CMC): relative molecular weight (Mn) 800 ~ 1200 g/mol, analytical purity, Aladdin; Polyethylene glycol (PEG) Mn ~ 4000 g/mol: analytical reagent, Shanghai Macklin Biochemical Co., Ltd.; Hexamethylene diisocyanate (HDI): >99.0%, Aladdin; tin 2-ethylhexanoate: C16H30O4Sn, >95.0%, Aladdin. Methylene blue (MB) and ethyl alcohol (≥99.7%) were obtained from Chengdu Chron Chemical Reagent Factory; Deionized water (DI), self-made in the laboratory.
2.2 Synthesis of the hydrogels
2.2.1 Preparation of polyurethane porous hydrogel (PUF)
Polyurethane foam was prepared by in situ polymerization and one-step foaming method. Specifically, 20 g of PEG solid was weighed into a 250 ml beaker, placed in an oven to maintain 100°C for 3 h to obtain molten PEG, and then the PEG was poured into the mold. 0.35 g of stannous octanoate, 3.5 g of HDI, and 0.5 g of deionized water were successively dropped into the mold, stirred vigorously at 80°C for 30 s, and the mixture was put into a 100°C oven for curing for 1 h, washed three times with deionized water, dried at 60°C for 8 h.
2.2.2 Preparation of CMC-PUF-s
CMC-PUF-s was prepared by one-step foaming method as PUF. First, five portions of 20 g PEG solid were weighed, put into a 250 ml beaker, and kept in a 100°C oven for 3 h. Then, the PEG was taken out and poured into the mold, and CMC powder of 5%, 10%, and 15% (based on the quality of PEG) was put into the mold, stirred evenly, and then 0.35 g stannous octanoate, 3.5 g HDI, and 0.5 g deionized water were dropped successively, and stirred vigorously at 80°C for 30 s. Then, it was put into an oven at 100°C for curing for 1 h, taken out, washed three times with deionized water, dried at 60°C for 8 h, and then taken out and recorded as CMC-PUF-5, CMC-PUF-10, and CMC-PUF-15.
2.3. Characterization
The microstructure morphology of the porous scaffolds was analyzed by using a field emission scanning electron microscope (FE-SEM, INSPECTF50, FEI, Holland) at an accelerating voltage of 10 kV, and the distribution of C, O, and N elements on the surface of the polyurethane hydrogel was investigated by energy-dispersive spectroscopy (EDS INSPECT F50). The samples were previously coated with gold in a sputtering device.
The water contact angle images (n = 3) were captured by a contact angle meter (SDC 200, Dongguan Yuding Precision Instrument Co., Ltd.).
The gel content (G) and swelling degree (S) of the polyurethane hydrogels were measured with ethyl acetate. The material was made into 10 × 10 × 10 mm block sample and weighed (W1). It was soaked at 37°C for 48 hours, and the solvent was changed every day. After soaking, the sample was taken out, the surface was absorbed with filter paper and weighed (W2). The gel content indicates the quantity of the insoluble fraction in polyurethane gel [Citation31]. Then, the samples were placed in an oven at 60°C for 24 hours until the weight remained constant and weighed (W3). At least five parallel samples were set for each material, and the gel content and swelling rate were calculated according to the following formula:
where W1 (g) is the weight of the sample before soaking, W2 (g) is the weight of the swollen sample, and W3 (g) is the constant weight of the sample after drying.
The concentration of MB solution was determined by UV–Vis spectrophotometric method. Firstly, the absorbance of MB solution was measured at a wavelength of 664 nm with the UV-Vis spectrophotometer (UV755B), and the corresponding solution concentration was calculated according to the calibrated standard curve.
The samples (10 102 mm) were prepared to analyze the functional groups of CMC-PUF-s by using a Fourier transform infrared spectrometer in the wavenumber range of 400–4000 cm−1 with 64 scans per spectrum at 4 cm−1 resolutions in total reflection mode (FTIR, TENSOR-27, Bruker, Germany).
The crystalline structure analysis was carried out using an X-ray diffractometer (XRD) (DX-2700, China Fangyuan Instrument Co., Ltd.) operated at 40 kV and 25 mA in a step size of 0.07° at a scanning speed of 0.31° s−1 with Cu-K radiation in the 2
range of 5°~70°.
2.4 Adsorption of methylene blue by CMC-PUF-s
2.4.1 Effect of CMC dosage on MB adsorption
The samples of PUF, CMC-PUF-5, CMC-PUF-10, and CMC-PUF-15 were cut into 0.1 g rectangular blocks, respectively. At 25°C, they were put into 50 ml of MB solution with a concentration of 10 mg/L and pH = 7, respectively, for static adsorption experiment. After 6 h, the adsorption equilibrium was reached, the concentration was measured, and the removal rate and adsorption capacity were calculated, so as to select the sample with better adsorption performance. This sample was used as the adsorbent of MB in all subsequent adsorption experiments. Formula (3) and formula (4) were used to calculate the dye removal rate and equilibrium adsorption capacity (qe, mg/g), respectively:
where C0 and Ce are the initial concentration and equilibrium concentration of MB, respectively, V (L) is the volume of solution, and m (g) is the mass of adsorbent.
2.4.2 Effect of different adsorbent dosage on MB adsorption
To explore the influence of different adsorbent dosage on MB adsorption, 0.1 g, 0.2 g, 0.3 g, 0.4 g, and 0.5 g CMC-PUF-10 samples were put into MB solution with concentration of 10 mg/L, volume of 50 ml, and pH of 7, and statically adsorbed for 6 h at 25°C to select the best adsorbent dosage.
2.4.3 Effect of time on MB adsorption
In order to study the effect of adsorption time on adsorption, 0.1 g CMC-PUF-10 sample was placed in 50 ml solution with pH = 7 of 10 mg/L and 30 mg/L, respectively, and was statically adsorbed at 25°C for a certain time. The solution concentration at different adsorption times was measured, and the removal rate and adsorption capacity were calculated to explore the optimal adsorption time.
2.4.4 Effect of different initial concentration of MB on adsorption
In order to explore the influence of different initial concentrations of MB on adsorption, 0.1 g CMC-PUF-10 samples were put into MB solutions with different concentrations (10–90 mg/L), respectively. The other conditions were the same, that is, pH = 7, volume of 50 ml, temperature of 25°C, static adsorption for 6 h to determine the solution concentration, so as to explore the best initial concentration of MB solution.
2.4.5 Effect of different pH on adsorption
In order to explore the influence of pH on MB adsorption, 0.1 g CMC-PUF-10 samples were put into MB solutions with different pH (3–11, pH adjusted with 0.1 M NaOH and 0.1 M HNO3 solution). Other conditions were the same, that is, the concentration was 10 mg/L, the volume was 50 ml, the temperature was 25°C, and the static adsorption was conducted for 6 h to determine the solution concentration, so as to explore the best pH conditions for adsorption.
2.4.6 Effect of different temperatures on MB adsorption
In order to explore the influence of temperature on MB adsorption, 0.1 g CMC-PUF-10 samples were put into MB solutions at different temperatures (25–60°C). Other conditions were the same, that is, the concentration was 10 mg/L, the volume was 50 ml, the pH was 7, and the solution concentration was measured after static adsorption for 6 h to explore the best pH conditions for adsorption.
2.4.7 Study on adsorption kinetics
In order to study the adsorption mechanism of MB by CMC-PUF, two adsorption kinetic models, pseudo-first-order (PFO) kinetic model and pseudo-second-order (PSO) kinetic model [Citation32], were used to evaluate the adsorption process. The fitting equation (Equationequation 5(5)
(5) and Equationequation 6)
(6)
(6) is as follows:
pseudo-first order [Citation33]:
pseudo-second order [Citation34]:
where qt (mg/g) is the adsorption capacity at a given time t (min), and qe (mg/g) is the equilibrium adsorption capacity. Here, k1(1/min) is the rate constant of the quasi-first-order adsorption kinetic model, and k2(g/(mg · min)) is the rate constant of the quasi-second-order adsorption kinetic model.
2.4.8 Study on adsorption isotherm
In order to further explore the interaction between adsorbent and adsorbate, Langmuir model and Freundlich model are used to fit the experimental data. The two adsorption isotherm model equations (Equationequations 7(7)
(7) and Equation8
(8)
(8) ) are as follows:
Langmuir [Citation35]:
Freundlich [Citation36]:
where Ce (mg/L) is the equilibrium concentration of MB; qm(mg/g) is the theoretical maximum equilibrium adsorption capacity of MB; KL (L/mg) is the balance factor; KF((mg/g)(L/mg)1/n) and n are Freundlich model constants [Citation37].
2.4.9 Study on adsorption thermodynamics
To better understand the feasibility and mechanism of adsorption process, thermodynamic studies were carried out. The thermodynamic parameters of MB adsorption were obtained from the adsorption experiments of CMC-PUF on MB at different temperatures (25°C, 40°C, 55°C, and 70°C). The relevant calculation formulas [Citation35,Citation38] (Equationequations 9(9)
(9) , Equation10
(10)
(10) and Equation11
(11)
(11) ) are as follows:
Gibbs free energy change of adsorption reaction ( Gθ):
Adsorption enthalpy change (Hθ) and Adsorption entropy change (
Sθ):
where R is the gas constant [J/(mol · K)]; T is the absolute temperature (K); Kd (dimensionless) is the standard thermodynamic equilibrium constant of adsorption based on molar concentrations; KM represents for KL or KF; Mw (g/mol) is the molar weight of adsorbate (MB ~319.6 g/mol); (mol/L) is the standard concentration of adsorbate; and
(dimensionless) is the activity coefficient of adsorbate.
2.4.10 Cycle performance test
In order to investigate the reusability of CMC-PUF, 0.1 g CMC-PUF was used at room temperature, the static adsorption concentration was 10 mg/L, and the volume was 50 ml of MB solution for 6 h. After adsorption equilibrium, the adsorbed CMC-PUF was washed with deionized water for three times, and soaked in 0.1 M HNO3 solution overnight to desorb MB. After desorption, it was washed with deionized water to make it neutral again, dried in a 45°C oven for 10 h, and then the next adsorption experiment was carried out. The adsorption-desorption process was repeated 5 times to evaluate the reusability of CMC-PUF.
3. Results and discussion
3.1 Physio-chemical properties of CMC-PUF foams
Hydrophilic polyurethane foams based on PEG with CMC were prepared, with high surface-to-volume ratio for better adsorption capability. Firstly, the chemical and crystalline structures were characterized to confirm the synthesis of the hydrogel foams. The XRD patterns of the synthesized polyurethane hydrogels and the adsorbed samples are shown in ). The peak at about 20° represents the characteristic bicrystal peak of polyurethane foam, which can be attributed to the existence of short-range ordered structure and the existence of amorphous soft and hard segments in PU [Citation39]. With the addition of CMC, the crystallinity behavior shows no significant differences, indicating well-crystalized structures for composite CMC-PUF hydrogel. ) and (c) shows the FTIR spectra of CMC and the synthesized samples. Most of the absorption peaks of CMC-PUF-s and PUF were basically the same, which demonstrated that the combination of CMC and PUF did not involve chemical reaction. In the FTIR spectrum of PU foam, the peaks at 3328 cm−1, 1648 cm−1, and 1550 cm−1 were attributed to N-H, CO-NH, and NH2 of carbamate, the peak at 2868 cm−1 was attributed to methyl, and the peak at 1095 cm−1 was attributed to C-O in polyether polyol [Citation40]. In the spectrum of CMC, 1621 cm−1 and 1425 cm−1 corresponded to the asymmetric tensile vibration and symmetric tensile vibration of coo- in sodium carboxymethyl cellulose, respectively [Citation41]. The corresponding absorption peaks appeared in the composite CMC-PUF-s, indicating successful synthesis of the porous hydrogels.
Figure 2. (a) XRD patterns of different polyurethane hydrogels, (b) FTIR spectra of polyurethane hydrogels with different CMC contents, (c) FTIR spectra of CMC and different polyurethane hydrogels, (d) gel content, (e) swelling rate, and (f) porosity of different samples.
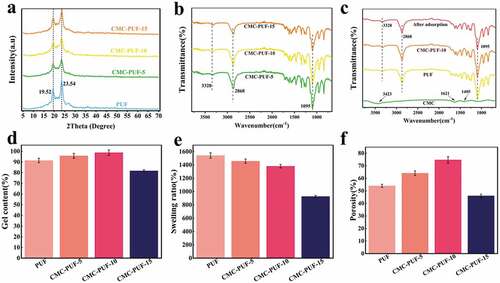
The gel content and swelling rate of different hydrogels are shown in ) and (e), respectively. All samples had high swelling rate and gel content. When the content of CMC increased, their swelling ratio decreased, while the gel content indicated the opposite trend. The swelling rate and gel content reflected the degree of cross-linking. More specifically, the higher the content of the gel, the lower the swelling rate, the higher the degree of cross-linking. This was due to the network structure of the composite hydrogels. With increasing CMC content, the crosslinking degree improved, resulting in a reduction of the pore size of the hydrogel, which decreased the swelling rate of the hydrogel. The low swelling rate of CMC-PUF-15 may be due to the less reactive groups between HDI and PEG by increasing CMC, resulting in a low degree of crosslinking.
3.2 Morphology and pore structure
) shows the porosity of the hydrogels with different CMC contents, while the SEM photos and pore size distribution of polyurethane foam with are shown in ) and (b), respectively. The results indicated that the pore structure of polyurethane foam was significantly influenced by the amount of CMC. PUF presents an interconnective three-dimensional porous structure, with low porosity of 53.99% and poor pore size distribution. After increasing the CMC content to 10%, the porosity increased to 74.67%. However, when the content of the CMC increased to 15%, the three-dimensional network structure of the sample became worse, and the porosity also decreased to 46.03%. This is due to the viscosity of the reaction process with excessive content of CMC, and the gas is not easily released out of the reaction system, which affected the foaming process of polyurethane foam. The contact angle test showed the hydrophilic changes of CMC-PUF-s, as shown in ). Except for CMC-PUF-15, the contact angle of the other samples was less than 90°, which was because of the increasing content of hydrophilic PEG. Combined with the SEM photos ()) and aperture distribution diagram ()), this may be because the hydrogen bond between CMC and PUF increased with the increase of CMC content, which made its three-dimensional network structure more uniform, resulting in the increase in its contact angle.
3. 3 EDS spectrum analysis
The EDS spectra of PUF and CMC-PUF-10 are shown in . Compared with PUF, the weight percentage of O element in CMC-PUF-10 was increased, and oxygen is the element with the largest mass ratio in carboxymethyl cellulose, which provided some evidence for the successful loading of CMC on PUF.
3.4 Adsorption comparison of MB by CMC-PUF-s
The removal rate of MB by polyurethane hydrogels with different CMC contents is shown in . The adsorption effect of pure PUF on MB solution was low with the removal rate of 12.5%. After adding CMC, the removal rates improved, respectively. After adding CMC, the removal rates significantly increased to 74.33% (5%CMC) and 81.47% (10%CMC), respectively. However, when CMC was increased to 15%, the adsorption effect on MB decreased. Thus, CMC-PUF-10 was selected as the adsorbent according to the result.
3.5 Effects of different factors on MB adsorption by CMC-PUF-10
3.5.1 Effect of different adsorbent dosage and time
Different adsorbent dosages were used for analysis of the influence of dosage content. As shown in ), when the dosage of adsorbent was 1 g/L, the removal rate of MB by adsorbent was less than 70%, while when the dosage was 2 g/L or above, the removal rate was more than 80%. This was because when the dosage of adsorbent was small, there were fewer reactive active sites, which affected the adsorption of MB. Considering the adsorption effect and economy, 2 g/L was selected as the dosage of adsorbent.
The change of removal rate with time is shown in ). The MB solution of 10 mg/L and 30 mg/L reached the adsorption equilibrium at 360 min, indicating that the initial concentration of MB solution had little effect on the time to reach the adsorption equilibrium.
3.5.2 Effect of initial concentration of MB on adsorption
The removal rate of MB by adsorbent decreased with the increase in initial concentration of MB. This result could be explained by the fact that with increasing solution concentration, the binding sites on the adsorbent surface were gradually occupied by dye molecules, and the adsorption gradually reached equilibrium. Therefore, the removal effect of the adsorbent on the dye molecules in the solution was reduced, resulting in the reduction of the removal rate [Citation42].
3.5.3 Effect of pH on MB adsorption
As could be seen from ), CMC-PUF-10 presented a high pH sensitivity for MB adsorption, while the adsorption rate was high in the range of pH 7–11. However, under acidic conditions, its adsorption capacity for MB decreased significantly. This phenomenon was mainly due to the competitive adsorption between a large amount of H+ and cationic dye MB in the solution under acidic conditions [Citation43]. When there was more H+ in the solution, the protonation phenomenon occurred, making -COO− protonation become -COOH [Citation44]. With the increase in pH, the charge density of MB solution decreased and the protonation phenomenon weakened, the abundant -COO− on the surface of CMC-PUF-10 resulted in a large number of reactive sites on the surface of the adsorbent, which increased the attraction between CMC-PUF-10 and MB, thus improved the adsorption effect [Citation45,Citation46].
3.5.4 Effect of temperature on MB adsorption
The influence of temperature results is shown in ), as a result, CMC-PUR-10 has a high-temperature sensitivity to MB adsorption, and its removal effect on MB decreased with the increase in temperature. Based on this result, 25°C was selected as the optimal condition for MB adsorption.
3.6 Study on adsorption kinetics
In order to further study, the adsorption mechanism of CMC-PUF-10 on MB, pseudo-first-order and pseudo-second-order models were used to fit the adsorption data. The experimental results are shown in and . By comparing the fitting parameters of the two models, the R2 of the pseudo-second-order kinetic model was 0.9675, significantly higher than the 0.7647 of the pseudo-first-order kinetic model, and the qe (4.06 mg/g) obtained from the pseudo-second-order kinetic model was closer to the experimental value (4.07 mg/g). In other words, the pseudo-second-order kinetic model was more suitable for describing the adsorption process of MB by CMC-PUF-10. This result is consistent with some literatures [Citation47–49]. These results suggested that the electrostatic attraction between -COO− and MB on the adsorbent played a very important role in the adsorption process [Citation50].
Table 1. Fitting constants of PFO and PSO equations for MB sorption.
3.7 Study on adsorption isotherm
Adsorption isotherm experiments are usually used to characterize the adsorption properties of adsorbents. In the equilibrium state, it is necessary to evaluate the distribution of adsorbate between adsorbent and liquid phases, which directly reflects the interaction between dye molecules and adsorbent. Two adsorption isotherm models, namely Langmuir model and Freundlich model, were used to fit the experimental data. The results are shown in and . The fitting curve was more in line with Langmuir model at four temperatures. At 25°C, R2 was 0.9905, which was significantly higher than Freundlich’s 0.8912. Furthermore, qm obtained from Langmuir model was 30.81 mg/g, which was also close to the experimental value. Similar results were reported for various sorbent-pollutant systems in literature [Citation51,Citation52]. These results demonstrated that the adsorption sites in CMC-PUF-10 were uniformly distributed, and the adsorption process was essentially monolayer adsorption [Citation53].
Table 2. Fitting parameters of Langmuir and Freundlich equations for CMC-PUF-10.
3.8 Thermodynamic study
The results of adsorption thermodynamics () suggested that the adsorption process of CMC-PUF-10 hydrogel for MB was spontaneous(<0) [Citation54]. With the increase in temperature, the spontaneity decreased. In the experiment (),
<0, indicating that the adsorption process of MB was an exothermic process [Citation55];
<0, indicated that the adsorption process was a process of entropy reduction. These results demonstrated that the adsorption of MB was more suitable at low temperature, which was consistent with the effect of temperature on the adsorption.
Table 3. Thermodynamic parameters for the adsorption of MB onto CMC-PUF-10.
3.9 Cycle performance evaluation
Further characterizations were done to explore the renewable performance of adsorbents. By comparing the SEM photos ()), XRD patterns ()), and FTIR patterns ()) of CMC-PUF-10 and CMC-PUF-10-MB, the surface morphology and pore structure presented no significant changes before and after adsorption, indicating an excellent structural and chemical stability. In order to test the reusable performance of the adsorbent, five adsorption desorption experiments were carried out, and the removal rate could reach 72.36% at the fifth cycle, indicating that CMC-PUF-10 can be reused as an adsorbent for MB. Moreover, unlike conventional adsorbents, which require complex operations such as centrifugation [Citation56], filtration [Citation57] or calcination [Citation58], the synthetic adsorbent only needs to be taken out of the solution, which reduced the use cost.
3.10 Comparison of maximum adsorption capacity with other adsorbent material
is showing several adsorbents for MB and their adsorption capacities. Ahmad B. Albadarin studied a new dye adsorbent, active lignin-chitosan extruded particles (AliCE), with a maximum adsorption capacity of 36.25 mg/g for methylene blue [Citation59]. HaoChen et al. activated palygorskite clay by heat treatment, and its maximum adsorption capacity for methylene blue is 78.11 mg/g [Citation60]. In contrast with some of the reported adsorbents, the adsorption capacity for CMC-PUF-10 is not superior compared to many adsorbents. However, these materials are either expensive, environmentally unfriendly, or require long-term chemical or heat treatment, which limits their applicability in practical applications.
Table 4. Comparison of the maximum adsorption capacity of the present study with that previously reported.
4. Conclusion
In this study, different doses of CMC were loaded into polyurethane foam (PUF) and manufactured series of composite foams (CMC-PUFs) by gas foaming method. Due to the open cell structure of PUF and a large number of carboxylate groups of CMC, CMC-PUF-10 can be used as a powerful adsorbent to remove MB as a cationic dye. SEM images showed that CMC-PUF-10 exhibited a great porous 3D structure, which could enhance the adsorption of MB molecules. SEM-EDS and FTIR analysis further proved the successful loading of CMC. The pore size analysis based on SEM images demonstrated that the porosity of CMC-PUF-10 was 74.67%, and the pore size was mainly distributed in the range of 0–300 μm. The adsorption experimental data showed that the removal rate of the composite hydrogel (CMC-PUF-10) was 81.47%, significantly higher than that of PUF (12.5%). In addition, the adsorption data fitted well with the Langmuir isotherm model, qm (mg/g) was 30.81 mg/g at pH = 7°C and 25°C. The adsorption behavior followed the PSO kinetic model, indicating that electrostatic attraction played an essential role in the adsorption process. At 25°C, < 0 and
< 0 indicated that the adsorption of MB by CMC-PUF-10 is a spontaneous and exothermic process. Furthermore, CMC-PUF-10 demonstrated significant pH responsiveness and reusability after five cycles of adsorption desorption with high removal rate of 72.78%. The obtained CMC-PUFs have potential applications in water treatment.
Acknowledgments
This project was financially supported by the Science and Technology Department of Sichuan Province (2021YFH0098).
Disclosure statement
No potential conflict of interest was reported by the author(s).
Additional information
Funding
References
- Bennett GF, Environmental chemistry of dyes and pigments: A. Reife and H.S. Freeman (Eds.), Wiley, New York, NY, 1995, $99.00, 329, J Hazard Mater 54(1) (1997) 124. Proceedings of the nineteenth Arctic and marine oil spill program (AMOP) technical seminar, Calgary, Alberta, Canada
- Qin Y, Wang L, Zhao C, et al. Ammonium-functionalized hollow polymer particles as a pH-responsive adsorbent for selective removal of acid dye. ACS Appl Mater Interfaces. 2016;8(26):16690–16698.
- Qamar M, Saquib M, Muneer M. Semiconductor-mediated photocatalytic degradation of anazo dye, chrysoidine Y in aqueous suspensions. Desalination. 2005;171(2):185–193.
- Tkaczyk A, Mitrowska K, Posyniak A. Synthetic organic dyes as contaminants of the aquatic environment and their implications for ecosystems: a review. SciTotal Environ. 2020;717:137222.
- Duman O, Tunç S, Polat TG, et al. Synthesis of magnetic oxidized multiwalled carbon nanotube-κ-carrageenan-Fe3O4 nanocomposite adsorbent and its application in cationic methylene blue dye adsorption. Carbohydr Polym. 2016;147:79–88.
- Abdel-Halim ES, Al-Deyab SS. Removal of heavy metals from their aqueous solutions through adsorption onto natural polymers. Carbohydr Polym. 2011;84(1):454–458.
- Doke SM, Yadav GD. Novelties of combustion synthesized titania ultrafiltration membrane in efficient removal of methylene blue dye from aqueous effluent. Chemosphere. 2014;117:760–765.
- Chafi M, Gourich B, Essadki AH, et al. Comparison of electrocoagulation using iron and aluminium electrodes with chemical coagulation for the removal of a highly soluble acid dye. Desalination. 2011;281:285–292.
- Fan L, Luo C, Li X, et al. Fabrication of novel magnetic chitosan grafted with graphene oxide to enhance adsorption properties for methyl blue. Journal of Hazardous Materials 215-216. 2012;215-216:272–279.
- Svetozarević M, Šekuljica N, Onjia A, et al. Biodegradation of synthetic dyes by free and cross-linked peroxidase in microfluidic reactor. Environ Technol Innovation. 2022;26:102373.
- Senthil Rathi B, Senthil Kumar P. Sustainable approach on the biodegradation of azo dyes: a short review. Current Opin Green Sustainable Chem. 2022;33:100578.
- Zaidi SZJ, Harito C, Bavykin DV, et al. Photocatalytic degradation of methylene blue dye on reticulated vitreous carbon decorated with electrophoretically deposited TiO2 nanotubes. Diam Relat Mater. 2020;109:108001.
- Bangari RS, Yadav A, Bharadwaj J, et al. Boron nitride nanosheets incorporated polyvinylidene fluoride mixed matrix membranes for removal of methylene blue from aqueous stream. J Environ Chem Eng. 2022;10(1):107052.
- Hien Tran T, Le AH, Pham TH, et al. A sustainable, low-cost carbonaceous hydrochar adsorbent for methylene blue adsorption derived from corncobs. Environ Res. 2022;212:113178.
- Karthi S, Sangeetha RK, Arumugam K, et al., Removal of methylene blue dye using shrimp shell chitin from industrial effluents, Materials Today: Proceedings Moodbdri, Karnataka, India, (2022). 10.1016/j.matpr.2022.05.428.
- Duman O, Ayranci E. Adsorption characteristics of benzaldehyde, sulphanilic acid, and p‐phenolsulfonate from water, acid, or base solutions onto activated carbon cloth. Sep Sci Technol. 2006;41(16):3673–3692.
- Cao X, Liu M, Bi W, et al. Direct carboxylation of cellulose in deep eutectic solvent and its adsorption behavior of methylene blue. Carbohydrate Polymer Technologies and Applications. 2022;4:100222.
- Mahmoud ME, El-Bahy SM, Elweshahy SMT. Decorated Mn-ferrite nanoparticle@Zn–Al layered double hydroxide@cellulose@ activated biochar nanocomposite for efficient remediation of methylene blue and mercury (II. Bioresour Technol. 2021;342:126029.
- Jawad AH, Saud Abdulhameed A, Wilson LD, et al. High surface area and mesoporous activated carbon from KOH-activated dragon fruit peels for methylene blue dye adsorption. Optimization and Mechanism Study, Chinese Journal of Chemical Engineering. 2021;32:281–290.
- Abdulhameed AS, Firdaus Hum NNM, Rangabhashiyam S, et al. Statistical modeling and mechanistic pathway for methylene blue dye removal by high surface area and mesoporous grass-based activated carbon using K2CO3 activator. J Environ Chem Eng. 2021;9(4):105530.
- Mondal A, Arora M, Kumar Dubey B, et al. Comparative assessment of the characteristics and Cr(VI) removal activity of the bimetallic Fe/Cu nanoparticles pre- and post-coated with carboxymethyl cellulose. Chem Eng J. 2022;444:136343.
- He X, Jia H, Sun N, et al. Fluorescent hydrogels based on oxidized carboxymethyl cellulose with excellent adsorption and sensing abilities for Ag+. Int J Biol Macromol. 2022;213:955–966.
- Xu D, Kong Q, Wang X, et al. Preparation of carboxymethyl cellulose/chitosan-CuO giant vesicles for the adsorption and catalytic degradation of dyes. Carbohydr Polym. 2022;291:119630.
- Zhao H, Liang Z-X, Gao -Z-Z. Facile preparation of floatable carboxymethyl cellulose-based composite hydrogel for efficient removal of organic dyes. Colloid Interface Sci Commun. 2022;49:100637.
- Chen Y, Long Y, Li Q, et al. Synthesis of high-performance sodium carboxymethyl cellulose-based adsorbent for effective removal of methylene blue and Pb (II. Int J Biol Macromol. 2019;126:107–117.
- Liu H, Tian X, Xiang X, et al. Preparation of carboxymethyl cellulose/graphene composite aerogel beads and their adsorption for methylene blue. Int J Biol Macromol. 2022;202:632–643.
- Eltaweil AS, Elgarhy GS, El-Subruiti GM, et al. Carboxymethyl cellulose/carboxylated graphene oxide composite microbeads for efficient adsorption of cationic methylene blue dye. Int J Biol Macromol. 2020;154:307–318.
- Sirajudheen P, Nikitha MR, Karthikeyan P, et al. Perceptive removal of toxic azo dyes from water using magnetic Fe3O4 reinforced graphene oxide–carboxymethyl cellulose recyclable composite: adsorption investigation of parametric studies and their mechanisms. Surf Interfaces. 2020;21:100648.
- Hong H-J, Lim JS, Hwang JY, et al. Carboxymethlyated cellulose nanofibrils(CMCNFs) embedded in polyurethane foam as a modular adsorbent of heavy metal ions. Carbohydr Polym. 2018;195:136–142.
- Selvasembian R, Gwenzi W, Chaukura N, et al. Recent advances in the polyurethane-based adsorbents for the decontamination of hazardous wastewater pollutants. J Hazard Mater. 2021;417:125960.
- Pongmuksuwan P, Harnnarongchai W, Katabunyanont S. Influence of temperature, time and crosslinking agent on structure and properties of polyurethane gel. Key Eng Mater. 2020;856:253–260.
- Mashkoor F, Nasar A. Preparation, characterization and adsorption studies of the chemically modified Luffa aegyptiaca peel as a potential adsorbent for the removal of malachite green from aqueous solution. J Mol Liq. 2019;274:315–327.
- Ho YS, McKay G. A comparison of chemisorption kinetic models applied to pollutant removal on various sorbents. Process SafEnviron Prot. 1998;76(4):332–340.
- Ho YS, McKay G. Pseudo-second order model for sorption processes. Process Biochem. 1999;34(5):451–465.
- Ye X, Wu L, Zhu M, et al. Lotus pollen-derived hierarchically porous carbons with exceptional adsorption performance toward reactive black 5: isotherms, kinetics and thermodynamics investigations. Sep Purif Technol. 2022;300:121899.
- He Z, Qin M, Han C, et al. Pectin/graphene oxide aerogel with bamboo-like structure for enhanced dyes adsorption. Colloids Surf A Physicochem Eng Asp. 2022;652:129837.
- Fan X, Deng L, Li K, et al. Adsorption of malachite green in aqueous solution using sugarcane bagasse-barium carbonate composite. Colloid Interface Sci Commun. 2021;44:100485.
- Tran HN, Lima EC, Juang R-S, et al. Thermodynamic parameters of liquid–phase adsorption process calculated from different equilibrium constants related to adsorption isotherms: a comparison study. J Environ Chem Eng. 2021;9(6):106674.
- Anju M, Renuka NK. Magnetically actuated graphene coated polyurethane foam as potential sorbent for oils and organics. Arabian J Chem. 2020;13(1):1752–1762.
- Rivera-Armenta JL, Heinze T, Mendoza-Martínez AM. New polyurethane foams modified with cellulose derivatives. Eur Polym J. 2004;40(12):2803–2812.
- Justi M, de Freitas MP, Silla JM, et al. Molecular structure features and fast identification of chemical properties of metal carboxylate complexes by FTIR and partial least square regression. J Mol Struct. 2021;1237:130405.
- Hu XS, Liang R, Sun GX. Super-adsorbent hydrogel for removal of methylene blue dye from aqueous solution. ?J Mater Chem A. 2018;6(36):17612–17624.
- Zhao J, Huang Q, Liu M, et al. Synthesis of functionalized MgAl-layered double hydroxides via modified mussel inspired chemistry and their application in organic dye adsorption. J Colloid Interface Sci. 2017;505:168–177.
- Liu M, Chen C, Hu J, et al. Synthesis of magnetite/graphene oxide composite and application for cobalt(II) removal. J Phys Chem C. 2011;115(51):25234–25240.
- McCafferty E. Relationship between the isoelectric point (pHpzc) and the potential of zero charge (Epzc) for passive metals. Electrochim Acta. 2010;55(5):1630–1637.
- Ren L, Tang Z, Du J, et al. Recyclable polyurethane foam loaded with carboxymethyl chitosan for adsorption of methylene blue. J Hazard Mater. 2021;417:126130.
- Duman O, Ayranci E. Adsorptive removal of cationic surfactants from aqueous solutions onto high-area activated carbon cloth monitored by in situ UV spectroscopy. J Hazard Mater. 2010;174(1):359–367.
- Ayranci E, Duman O. Structural effects on the interactions of benzene and naphthalene sulfonates with activated carbon cloth during adsorption from aqueous solutions. Chem Eng J. 2010;156(1):70–76.
- Duman O, Tunç S, Polat TG. Determination of adsorptive properties of expanded vermiculite for the removal of C. I. Basic Red 9 from aqueous solution: kinetic, isotherm and thermodynamic studies. Appl Clay Sci. 2015;109-110:22–32. 109-110.
- Wei X, Chen D, Wang L, et al. Carboxylate-functionalized hollow polymer particles modified polyurethane foam for facile and selective removal of cationic dye. Appl Surf Sci. 2022;579:152153.
- Duman O, Polat TG, Diker CÖ, et al. Agar/κ-carrageenan composite hydrogel adsorbent for the removal of methylene blue from water. Int J Biol Macromol. 2020;160:823–835.
- Duman O, Tunç S, Bozoğlan BK, et al. Removal of triphenylmethane and reactive azo dyes from aqueous solution by magnetic carbon nanotube-κ-carrageenan-Fe3O4 nanocomposite. J Alloys Compd. 2016;687:370–383.
- Li K, Zheng Z, Li Y. Characterization and lead adsorption properties of activated carbons prepared from cotton stalk by one-step H3PO4 activation. J Hazard Mater. 2010;181(1):440–447.
- Tang S, Yang J, Lin L, et al. Construction of physically crosslinked chitosan/sodium alginate/calcium ion double-network hydrogel and its application to heavy metal ions removal. Chem Eng J. 2020;393:124728.
- Long W, Yang C, Wang G, et al. Effective adsorption of Hg(II) ions by new ethylene mimine polymer/β-cyclodextrin crosslinked functionalized magnetic composite. Arabian J Chem. 2022;104439. 10.1016/j.arabjc.2022.104439
- Deng S-Q, Miao Y-L, Tan Y-L, et al. An anionic nanotubular metal–organic framework for high-capacity dye adsorption and dye degradation in darkness. Inorg Chem. 2019;58(20):13979–13987.
- Wang Y, Wang S, Li Z, et al. Synthesis of UiO-66 in Supercritical CO2 and its application in dye adsorption. Ind Eng Chem Res. 2021;60(1):771–780.
- He Q, Liu X, Wang Y, et al. Circular conversion of waste rectorite@dye to efficient and pH-resistant heterogeneous silicate adsorbents for cyclic and complete dye removal. Appl Clay Sci. 2022;225:106556.
- Albadarin AB, Collins MN, Naushad M, et al. Activated lignin-chitosan extruded blends for efficient adsorption of methylene blue. Chem Eng J. 2017;307:264–272.
- Chen H, Zhao J, Zhong A, et al. Removal capacity and adsorption mechanism of heat-treated palygorskite clay for methylene blue. Chem Eng J. 2011;174(1):143–150.
- Ngulube T, Gumbo JR, Masindi V, et al. Preparation and characterisation of high performing magnesite-halloysite nanocomposite and its application in the removal of methylene blue dye. J Mol Struct. 2019;1184:389–399.
- Janbooranapinij K, Yimponpipatpol A, Ngamthanacom N, et al. Conversion of industrial carpet waste into adsorbent materials for organic dye removal from water. Cleaner Engineering and Technology. 2021;4:100150.
- Wang S, Ma Q, Zhu ZH. Characteristics of coal fly ash and adsorption application. Fuel. 2008;87(15):3469–3473.
- Wang R-F, Deng L-G, Li K, et al. Fabrication and characterization of sugarcane bagasse–calcium carbonate composite for the efficient removal of crystal violet dye from wastewater. Ceram Int. 2020;46(17):27484–27492.