ABSTRACT
Research on microcapsules has been conducted in recent years given trends in miniaturization and novel functionalization. In this work, we designed and prepared a series of unique shape memory polyurethane (SMPU) microcapsules with stimuli-responsive functions. The microcapsule has a core-shell structure in which the surface morphology can be adjusted, and it has a certain load-bearing capacity. In addition, the SMPU microcapsule has a stimuli-responsive function for shape memory and solvent response. The temperature of its shape recovery is approximately body temperature, and it can swell to rupture under the stimulation of organic solvents. Thus, the SMPU microcapsule has potential applications in biomedical fields, such as drug release.
GRAPHICAL ABSTRACT
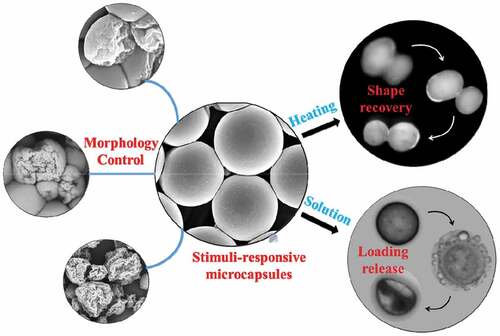
1. Introduction
Shape memory polymers (SMPs), as a class of smart polymers, can spontaneously recover from a temporary shape to a permanent shape when exposed to external stimuli [Citation1,Citation2], such as heat [Citation3], light [Citation4], electrical fields [Citation5] and magnetic fields [Citation6]. A heat-induced SMP can be made to a temporary shape by applying an external force upon heating above its transition temperature (Ttrans). The temporary shape can be fixed by cooling the SMP and then it will spontaneously recover to its permanent shape upon reheating above Ttrans [Citation7]. SMPs are used in aerospace [Citation8], biomedical applications [Citation9], and electronic devices [Citation10] owing to their excellent biodegradability, large shape deformability, and tailorable transition temperature. Among them, shape memory polyurethane (SMPU), with a molecular microphase structure that includes soft segments and hard segments, has been widely studied because the Ttrans can be conveniently adjusted by changing the ratio of the soft segment to the hard segment [Citation11]. Currently, SMPU, as a hydrolytically and enzymatically degradable polymer, is widely applied in biomedical fields owing to its adjustable Ttrans, good mechanical properties, and biocompatibility [Citation12].
A capsule is a structure in which a substance completely encloses a second substance or mixture of substances [Citation13–15]. It differs from homogeneous particles owing to the distinct outer layer, forming a core-shell structure [Citation16,Citation17]. This structural feature endows the capsule with many advantages. For example, the shell can protect the inner core substance from the external environment, improving stability and separating incompatible components [Citation18]. It is also possible to control and release encapsulated substances and contain liquids and gases with a solid shell [Citation19]. In addition, the capsule can retain the characteristics of the encapsulated substance, combine the properties of different materials, and even introduce new attributes to the system [Citation20–22]. The polymerization mechanism of the core-shell structure can be divided into interfacial polymerization (two reactive monomers in different phases) and in-situ polymerization (all monomers in the same phase) [Citation23–25]. In interfacial polymerization, the two monomers dispersed in different phases occur in polymerization reactions as they diffuse to the droplet interface. It is the most commonly used method for preparing polyamide, polyurethane (PU), and polyurea capsules [Citation26–29]. Owing to the flexibility of the preparation technology and the diversity of monomers and encapsulated materials, these capsules and microcapsules can be widely used in a variety of fields [Citation30–34], including paints, textiles, food, agriculture, drug delivery and release, catalysts, self-healing, and other areas [Citation35–38].
The surface morphology of capsules can vary considerably; intentional control of the surface morphology of the capsule has also attracted widespread attention. In 2007 and 2012, Xia et al. prepared microcapsules with pore structures on the surface. They controlled the porosity and pore size of the capsule surface by adjusting the concentration of the polymer solution and the degree of latex beads in the polymer solution [Citation39,Citation40]. The capsule morphology can be changed by improving the preparation method, such as using the layer-by-layer (LbL) process to deposit substances with opposite charges to obtain capsules with inorganic particles on the surface [Citation41] and using the template method to add external stimuli to obtain microcapsules with controlled morphology [Citation42]. Bielski et al. [Citation43] recently introduced various functional groups into monomers to retain them on the outer surface of capsules during interfacial polymerization so that they can be used for drug transport, pathogen removal, nutrient transfer, and antigen detection. Research on capsules is in progress. Yang et al. [Citation44] used the emulsion template method to prepare polyethylene glycol (PEG) capsules, which can be used for targeted drug delivery with high drug release rates. Jeyaraman et al. [Citation45] used PEG latex polymer capsules to encapsulate nanoparticles. Experiments confirmed that they did not participate in any immune response so that the capsules could be used for biological imaging. However, the shape of these capsules could not be easily changed. Zhang et al. [Citation46] prepared shape memory microcapsules that could be actively deformed.
Based on the current research, a series of core-shell microcapsules with controllable morphologies and high loadings was synthesized. The microcapsules were fabricated with interfacial polymerization at the oil/water interface. The resulting microcapsules demonstrated multi-stimuli-responsive functions, including shape memory behavior and solvent response behavior. The shape memory behavior of these microcapsules was studied by changing the temperature, thereby obtaining temporary and permanent shapes. The microcapsules also demonstrated swelling in organic solvents, which was not demonstrated in previous studies. Owing to these unique properties, the PU microcapsules have the potential for use in drug release and self-healing.
2. Experimental section
2.1 Materials
Urethane reaction (gelation) catalyst (1,4-Diazabicyclo[2.2.2]octane solution, Dabco® 33-LV), chain extender (1,4-Butanediol (BDO)), and aromatic diisocyanate (4,4’-Methylenebis(phenyl isocyanate) (MDI)) were obtained from Sigma-Aldrich. Polyethylene glycol (PEG), polyvinyl alcohol (PVA, 1788), dioctyl terephthalate (DOTP), toluene, Tin (II)2ethylhexanoate, ethanol and acetic acid were obtained from Aladdin.
2.2 Preparation of core/shell PU microcapsules
Firstly, 0.6 g MDI was added to the oil phase (10 mL) (DOTP, toluene, or DOTP: toluene mixture, all with a fluorescent dye to enable rapid detection of small capsules). Next, an appropriate amount of PVA was dissolved in deionized water to form a 1 wt% aqueous solution. 0.6 g PEG (molecular weight 1500) was then dissolved in the PVA solution. The water and oil components were mixed at a water-to-oil volume ratio of 80:10. To obtain a homogeneous emulsion, the solutions were stirred at different speeds (5000, 10 000, and15000rpm) for 15 min at 25°C. The emulsion was then purged with nitrogen at 65°C for 30 minutes. Finally, Tin (II)2ethylhexanoate, 1,4-Diazabicyclo[2.2.2]octane and 1,4-Butanediol (BDO) (0.18 g) were added to the emulsion that had been purged with nitrogen, and the temperature was raised to 80°C and maintained for 240 min. The resulting microcapsules were washed five times with deionized water and subsequently collected in a centrifuge (Thermo Scientific) for the separation of the water and the capsules (12 000 rpm, 30 min). The detail for the preparation of PU capsules is listed in .
Table 1. Capsules fabricated under different conditions.
2.3 Multistimuli-responsive behavior of microcapsules
To determine the shape memory behavior, the prepared shape memory polymer polyurethane (SMPU) microcapsules were first loaded in a 10 wt% PVA water solution. Subsequently, after evaporating water at room temperature, a solid PVA film was obtained, and the PVA film was stretched using DMA from room temperature to 90°C at 10°C/min. The temporary shape of the SMPU was obtained by dissolving the film in deionized water. When the temperature rises above the Ttrans, the microcapsules revert from the ellipse shape to the spherical shape.
2.4 Solvent response behavior
The microcapsules were placed in ethanol and acetic acid. It can be observed that the capsules have a specific response to organic solvents and gradually swell to rupture or recover to the original state after swelling as described in Section 3 Results and Discussion.
2.5 Characterization
The microcapsule was sputtered with Pt for 1 min, and SEM was used to observe the surface structure of the microcapsules. The average diameter of the microcapsules was calculated from Image J software under different conditions. The shape recovery process of the microcapsules was observed by optical microscopy (Zeiss optical microscopes with different magnifications). The shape memory properties of the stretched SMPU microcapsules were evaluated by placing them in water at a temperature higher than the Ttrans during the heating phase. The thermal properties were measured from −80°C to 100°C using differential scanning calorimetry (DSC, TA) at a heating and cooling rate of 10°C/min, under a nitrogen atmosphere. The solvent-responsive behavior of the microcapsules was evaluated by exposure to ethanol and acetic acid.
3. Results and discussion
3.1 Basic properties of composites
The microcapsules were fabricated with MDI and the structure of the reactive monomer and the obtained PU microcapsules are shown in Figure.S1. The preparation process is shown in . First, MDI was dispersed in the oil while PEG and BDO were dissolved in the water, and they were mixed in a flask under a nitrogen environment. This mixture was heated to 65°C for 30 minutes for the reactive monomers to undergo interfacial polymerization at the water-oil interface to form a polymer. Finally, after heating at 80°C for 4 h, the chain extender BDO and the polymer undergo secondary crosslinking to obtain the oil-in-water PU microcapsules with a core-shell structure.Owing to the structural properties of the monomer, this microcapsule has thermal excitation response behavior, that is, shape memory function. The MDI part forms the hard segment of the PU, which acts to fix the temporary shape, and the PEG part serves as the soft segment of the PU, which determines the shape recovery of the capsule.
Figure 1. (a) Schematic illustration of the fabrication of the microcapsules, (b) synthetic scheme and structure of the microcapsules.
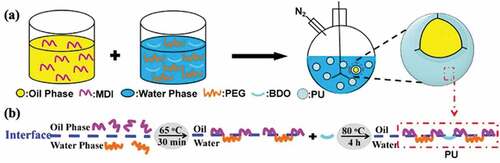
Maintaining other conditions, the surface morphology of the PU microcapsules prepared using different oil phase components was observed to be quite different (). SEM characterization revealed that only the surfaces of PU1 were completely smooth. In contrast, the surfaces of PU2, PU3, and PU4 all demonstrated different degrees of wrinkles. With an increase in the toluene solution content in the oil phase, the number of surface-wrinkled PU microcapsules increased, the degree of wrinkles increased, and the average diameter of the capsules decreased significantly. When the oil phase was a pure toluene solution, the surfaces of the PU microcapsules were all wrinkled (). It can be seen that the structure and surface morphology of the capsule can be effectively controlled by controlling the preparation process of the capsule, and this ability to tailor the structure broadens its application potential. For example, volume change can result in carrying capacity change, thereby better controlling the drug carrying capacity.
Figure 2. SEM images of microcapsules with different morphologies: (a) PU1, (b) PU2, (c) PU3, and (d) PU4.
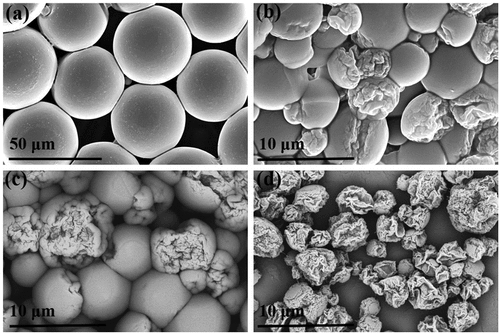
For the PU1 system, the oil phase is a single component. It can be seen from the SEM image that the surfaces of the microcapsules are extremely smooth. The diameter is approximately 50 μm (). The core-shell structure of the microcapsule and the small particle protrusion structure on the inner surface can be observed by mechanical crushing of the capsule or selecting the incompletely synthesized capsule. The thickness of the capsule shell is approximately 4.5 μm (). Because the microcapsules in other systems have wrinkles of different degrees, it affects the observation of their average diameter and thickness. When the microcapsule was pierced with a capillary pipette, the oil phase droplets could be withdrawn from the inside, thus verifying that it has an oil-in-water core-shell structure (FigureS2). The core-shell structure and the protrusion structure on the inner surface of the capsule are also well supported by the TEM image (). Microcapsules with a core-shell structure provide broader application potential for drug transport, catalyst delivery, and material encapsulation.
Figure 3. SEM images of microcapsules: (a) smooth surface, (b) broken capsules. The diameter is approximately 50 μm and the thickness is approximately 4.5 μm. The ratio between MDI, PEG, and BDO is 6:1:5; TEM images of microcapsules: (c) core-shell structure, (b) core-shell structure and convex structure.
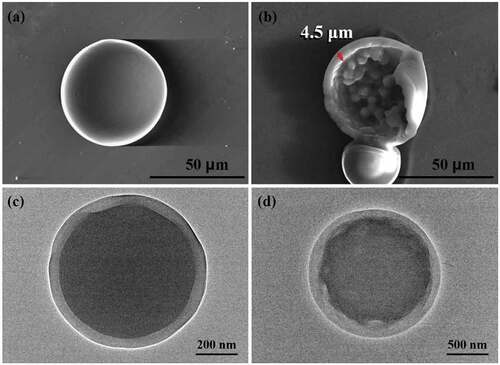
FTIR results shown in indicate that the new peaks of PU at 1720 cm−1 and 3310 cm−1 belong to the stretching vibration of C = O and N-H, respectively. The DSC result shown in demonstrates that the glass transition temperature (Tg) of the MDI-based capsules is approximately 35.4°C which can be triggered by body temperature without an external heat source to realize the shape memory function, and this feature provides potential applications in the biomedical field.
3.2 Shape memory behaviors
The MDI-based SMPU capsule film were deformed by stretching in a PVA film at 90°C (). PVA was selected as the film because it is a thermoplastic polymer that has good water solubility, high glass transition temperature Tg, and high stretchability at elevated temperature (T> Tg) [Citation47]. For this test, the microcapsules were dyed green with fluorescent dyes, dispersed in the PVA solution, and then evaporated to obtain the spherical microcapsules embedded in the PVA film. The morphology could be observed using an optical microscope (). To obtain microcapsules whose temporary shape is fixed to an ellipsoid, the PVA films embedded with microcapsules were heated to 90°C, stretched to 100% to make the microcapsules appear ellipsoidal (), cooled to room temperature, and then dissolved in water to remove the PVA film. Finally, when heated to 60°C, the microcapsules returned to a spherical shape (original shape). In addition, the shape recovery process of the MDI-based shape memory microcapsules was studied in detail. The ellipsoidal microcapsules (temporary shape) were placed into water and heated to 40°C. The shape of the microcapsules was recovered gradually and returned to the spherical shape (initial shape) within 60s (). Controlled wrinkles were also created on the surface of the microcapsules. When the temperature exceeded the transition temperature, the wrinkles disappeared gradually, the surface became smoother, and the capsule returned to its initial state (FigureS3).
Figure 5. Capsule memory behavior: (a) (i) Schematic illustration of SMPU microcapsule shape programming processes, (ii) original shape of optical microscope images of SMPU capsules with fluorescent dye, (iii) stretched shape of optical microscope images of SMPU capsules with fluorescent dye (stretching to 100% at 90°C), (b) Shape recovery behavior of MDI-based SMPU capsules at 40°C, (c) Shape recovery behavior of MDI-based SMPU capsules under the fluorescence microscope. The ratio of MDI, PEG, and BDO is 6:1:5.
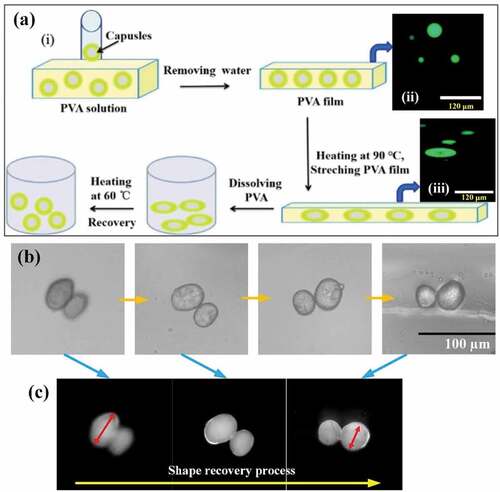
3.3 Solvent response behaviors
The microcapsules respond not only to thermal stimuli to achieve shape memory function but also to organic solvents. As shown in , when the ethanol solution was applied to the microcapsule, the capsule swelled, its volume increased, and the shell layer thickened. When it swells to a certain extent and then ruptures, the oil phase in the core can be observed to flow out. When the outward flow was complete, the capsules shrunk. After the microcapsules swelled under the action of ethanol, they could return to their original shape (Figure.S4). In addition, the response mechanism of PU capsules to ethanol was not unique. Acetic acid as an organic solvent could also stimulate the microcapsules to achieve the response effect of swelling to rupture, expelling the oil, and then shrinking ().
Figure 6. Solvent response behaviors of microcapsules: (a) ethanol-induced, (b) acetic acid-induced.
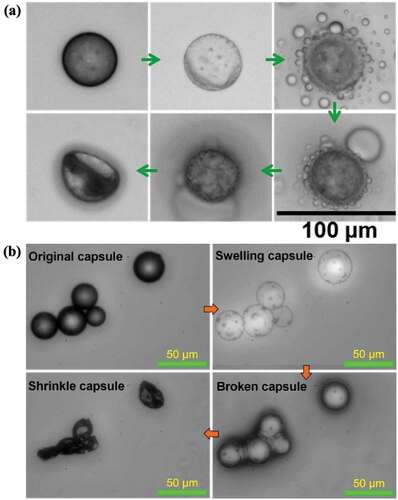
In contrast, the PU microcapsules demonstrated a larger response to the ethanol solution, and the swelling volume was greater. This response phenomenon of the microcapsules to organic solvents has the potential for use in drug release. The capsules can carry drugs to the affected area, and after arriving at the designated location, the organic solvent is stimulated to rupture the capsule to achieve a precise, controlled drug release. It was also demonstrated that the microcapsule can also release internal substances under the action of external pressure, but the capsule itself does not rupture (FigureS5).
The mechanism of the response of the microcapsules to organic solvents, that is, the reasons for the swelling of the capsules, is described in the following discussion. Swelling refers to the phenomenon in which solvent molecules enter the interior of the polymer through diffusion, causing its volume to expand. The microcapsule has an oil-in-water core-shell structure, the shell layer is composed of PU polymer, and the core is composed of an oil phase. Organic solvents such as ethanol have relatively small molecules and easily penetrate the PU shell of the capsule through diffusion to the capsule interior, thus causing the volume of the capsule to expand, that is, swelling (). At the same time, solvent molecules such as ethanol can form a hydrogen bond with the carbamate group (– NHCOO) of PU (). Studies have shown that hydrogen bonds can affect the swelling behavior of macromolecules [Citation48]. The swelling behavior in polar solvents mainly reflects the interaction between macromolecules and solvent molecules. The formation of hydrogen bonds can weaken the force between molecules, making the polymer more prone to swelling [Citation49]. Therefore, molecules, such as ethanol with a smaller volume, can directly enter the interior of the capsule through diffusion at the part that cannot form hydrogen bonds with PU but can form hydrogen bonds at the shell layer containing carbamate groups (– NHCOO) without entering the interior of the capsule. Therefore, when the capsule swells, its shell also thickens, and this combination can enhance the swelling effect of the capsule. After the capsule swells to a certain extent, the number of internal molecules increases sharply, and the shell is not strong enough to support the internal pressure and ruptures. The internal oil phase flows out in the form of droplets, and the organic solvent molecules are almost completely released (). As the oil phase flows out, the capsule gradually shrinks. When the oil phase completely flows out, the capsule shell shrinks, though some oil phase droplets remain attached (). Therefore, the response mechanism of the microcapsule to organic solvents provides a theoretical understanding for its application in precise drug release.
4. Conclusions
In summary, through interfacial polymerization, we prepared a series of oil-in-water core-shell structure PU microcapsules with multi-stimuli-responsive behavior. Their surface morphology can be adjusted and they have a specific carrying capacity. Compared to other capsules and particles, these microcapsules are more controllable and have novel shape memory functions. Their release temperature is 35.4°C, lower than body temperature, which can be used advantageously in the human body. Moreover, the microcapsule also has a unique solvent response behavior, and it can swell to rupture under the action of organic solvents, thereby releasing their internal substances. This feature can be applied to precisely controlled drug release, and the analysis of the swelling mechanism is sufficient to support the development of new systems. Other response behaviors of the microcapsule are worthy of further investigation and development. Hence, based on this, multi-stimuli-responsive microcapsules have great potential in biomedicine for applications such as drug release and self-healing.
Supplemental Material
Download MS Word (177.9 KB)Acknowledgments
This work is funded by the National Natural Science Foundation of China (Grant No. 11802075, 12072094). This work is also supported by the China Postdoctoral Science Foundation funded project.
Disclosure statement
No potential conflict of interest was reported by the author(s).
Supplementary material
Supplemental data for this article can be accessed online at https://doi.org/10.1080/19475411.2023.2166143
Additional information
Funding
References
- Hua C, Liu K, Wu Y, et al. An O-carborane derivative of perylene bisimide-based thin film displaying both electrochromic and electrofluorochromic properties. ACS Appl Mater Interfaces. 2021;13(41):49500–49508.
- Zhang G, Zhao Q, Zou W, et al. Unusual aspects of supramolecular networks: plasticity to elasticity, ultrasoft shape memory, and dynamic mechanical properties. Adv Funct Mater. 2016;26(6):931–937.
- Zhang Y F Y, Zhang N, Hingorani H, et al. Fast‐response, stiffness‐tunable soft actuator by hybrid multimaterial 3D printing. Adv Funct Mater. 2019;29(15):1806698.
- Wang L, Zhang F, Du S, et al. 4D printing of shape-changing structures based on IPN epoxy composites formed by UV post-curing and γ-ray radiation. Compos Part A Appl Sci Manuf. 2022;162:107146.
- Dong X, Zhang F, Wang L, et al. 4D printing of electroactive shape-changing composite structures and their programmable behaviors. Compos Part A Appl Sci Manuf. 2022;157:106925.
- Zhang F, Wang L, Zheng Z, et al. Magnetic programming of 4D printed shape memory composite structures. 2019;125:105571.
- Zhang F, Zhang Z, Liu Y, et al. Thermosetting epoxy reinforced shape memory composite microfiber membranes: fabrication, structure and properties. Compos Part A Appl Sci Manuf. 2015;76:54–61.
- Zhang D, Liu L, Leng J, et al. Ultra-light release device integrated with screen-printed heaters for CubeSat’s deployable solar arrays. Compos Struct. 2020;232:111561.
- Lin C, Lv J, Li Y, et al. 4D‐printed biodegradable and remotely controllable shape memory occlusion devices. Adv Funct Mater. 2019;29(51):1906569.
- Zhao Q, Wang Y, Cui H, et al. Bio-inspired sensing and actuating materials. J Mater Chem C. 2019;7(22):6493–6511.
- Yao Y, Zhou T, Wang J, et al. ‘Two way’shape memory composites based on electroactive polymer and thermoplastic membrane. Compos Part A Appl Sci Manuf. 2016;90:502–509.
- Xie R, Hu J, Hoffmann O, et al. Self-fitting shape memory polymer foam inducing bone regeneration: a rabbit femoral defect study. Biochim Biophys Acta. 2018;1862(4):936–945.
- Ballweg T, von Daake H, Hanselmann D, et al. Versatile triggered substance release systems via a highly flexible high throughput encapsulation technique. Appl Mater Today. 2018;11:231–237.
- Sun H, Cui J, Ju Y, et al. Tuning the properties of polymer capsules for cellular interactions. Bioconjug Chem. 2017;28(7):1859–1866.
- Kitayama Y, Takeuchi T. Fabrication of redox‐responsive degradable capsule particles by a shell‐selective photoinduced cross‐linking approach from spherical polymer particles. Chem–A Eur J. 2017;23(52):12870–12875.
- C BJA, M A-KM, M SA, et al. Achieving gastroresistance without coating: formulation of capsule shells from enteric polymers. Eur J Pharm Biopharm. 2019;144:174–179.
- Odrobińska J, Gumieniczek-Chłopek E, Szuwarzyński M, et al. Magnetically navigated core–shell polymer capsules as nanoreactors loadable at the oil/water interface. ACS Appl Mater Interfaces. 2019;11(11):10905–10913.
- N KB, C FB, K DS, et al. Nano-casting procedure for catalytic cobalt oxide bead preparation from calcium-alginate capsules: activity in ammonia borane hydrolysis reaction. Appl Mater Today. 2021;22:100952.
- Nguyen HVM, Kim CH, Kim JK. A case study of paraffin double-walled microencapsulation preparation using acrylic polymer and melamine polymer for thermal energy storage. J Korean Solar Energy Society. 2019;39(5):65–78.
- P ME, N BT, G YD, et al. Enzymatic degradation of the polymer capsules with a hydrophobic core in the presence of Langmuir lipid monolayer as a model of the cellular membrane. Colloids Surf B Biointerfaces. 2019;184:110464.
- Mironov EP, Borodina TN, Bukreeva TV. Interaction between polymer capsules with hydrophobic cores and a model cellular membrane at an air–water interface. Colloid J. 2017;79(4):508–514.
- Vázquez‐Mera N, Roscini C, Hernando J, et al. Liquid‐filled capsules as fast responsive photochromic materials. Adv Opt Mater. 2013;1(9):631–636.
- Santos ANB, Santos DJ, Carastan DJ. Microencapsulation of reactive isocyanates for application in self-healing materials: a review. J Microencapsul. 2021;38(5):338–356.
- Zhu DY, Rong MZ, Zhang MQ. Self-healing polymeric materials based on microencapsulated healing agents: from design to preparation. Prog Polym Sci. 2015;49:175–220.
- Cui J, Wang Y, Postma A, et al. Monodisperse polymer capsules: tailoring size, shell thickness, and hydrophobic cargo loading via emulsion templating. Adv Funct Mater. 2010;20(10):1625–1631.
- Quevedo E, Steinbacher J, McQuade DT. Interfacial polymerization within a simplified microfluidic device: capturing capsules. J Am Chem Soc. 2005;127(30):10498–10499.
- Zhang L, Liu P. Polyaniline micro/nano capsules via facile interfacial polymerization approach. Soft Mater. 2010;8(1):29–38.
- H KK, M SJ, Klinger D, et al. Particles with tunable porosity and morphology by controlling interfacial instability in block copolymer emulsions. ACS Nano. 2016;10(5):5243–5251.
- Liu W, He G, He Z. To prepare chitosan capsules via interfacial initiated chitosan macromonomer in situ polymerization. Polym Bull. 2012;68(6):1515–1524.
- Li Y, Guo Y, Ge J, et al. In situ silica nanoparticles-reinforced biodegradable poly (citrate-siloxane) hybrid elastomers with multifunctional properties for simultaneous bioimaging and bone tissue regeneration. Appl Mater Today. 2018;10:153–163.
- D RA, Marques J, Forte M, et al. Microencapsulation of citronella oil for solar-activated controlled release as an insect repellent. Appl Mater Today. 2016;5:90–97.
- C PN, Dinoro J, Ren J, et al. Additive manufacturing enables personalised porous high-density polyethylene surgical implant manufacturing with improved tissue and vascular ingrowth. Appl Mater Today. 2021;22:100965.
- Rahiminezhad Z, M TA, Borandeh S, et al. Janus nanoparticles: new generation of multifunctional nanocarriers in drug delivery, bioimaging and theranostics. Appl Mater Today. 2020;18:100513.
- Shen J, Wang W, Zhai X, et al. 3D-printed nanocomposite scaffolds with tunable magnesium ionic microenvironment induce in situ bone tissue regeneration. Appl Mater Today. 2019;16:493–507.
- Kudryavtseva V, Boi S, Read J, et al. Biodegradable defined shaped printed polymer microcapsules for drug delivery. ACS Appl Mater Interfaces. 2021;13(2):2371–2381.
- Kitayama Y, Harada A. Interfacial photo-cross-linking: simple but powerful approach for fabricating capsule polymer particles with tunable ph-responsive controlled release capability. ACS Appl Mater Interfaces. 2021;13(8):10359–10375.
- Udoh CE, Cabral JT, Garbin V. Nanocomposite capsules with directional, pulsed nanoparticle release. Sci Adv. 2017;3(12):eaao3353.
- A GF, Van Tittelboom K, Van Stappen J, et al. Integral procedure to assess crack filling and mechanical contribution of polymer-based healing agent in encapsulation-based self-healing concrete. Cem Concr Compos. 2017;77:68–80.
- Jeong U, H IS, C CPH, et al. Microscale fish bowls: a new class of latex particles with hollow interiors and engineered porous structures in their surfaces. Langmuir. 2007;23(22):10968–10975.
- Y BM, H MC, Zhang L, et al. A facile and general method for the encapsulation of different types of imaging contrast agents within micrometer‐sized polymer beads. Adv Funct Mater. 2012;22(4):764–770.
- Pei H, Bai Y, Guo J, et al. Tunable morphologies of polymer capsules templated from cuprous oxide particles for control over cell association. Chin Chem Lett. 2020;31(2):505–508.
- Guo X, S GK, Lynn DM. Templated synthesis of polymer-based yolk/shell particles with tunable morphologies. Chem Mater. 2019;31(18):7443–7452.
- Bielski R, Witczak ZJ, Newport JFL. Carbohydrate-based micro/nanocapsules with controlled external surface for medical applications. Front Chem. 2020;8:545.
- Yang S, Ding F, Gao Z, et al. Fabrication of poly (ethylene glycol) capsules via emulsion templating method for targeted drug delivery. Polymers. 2020;12(5):1124.
- Jeyaraman J, Malecka A, Billimoria P, et al. Immuno-silent polymer capsules encapsulating nanoparticles for bioimaging applications. J Mat Chem B. 2017;5(26):5251–5258.
- Zhang F, Zhao T, Ruiz-Molina D, et al. Shape memory polyurethane microcapsules with active deformation. ACS Appl Mater Interfaces. 2020;12(41):47059–47064.
- V PT, A EA, K BA, et al. Plasticity control of poly (vinyl alcohol)–graphene oxide nanocomposites. RSC Adv. 2020;10(40):24027–24036.
- Chen M, Coasne B, Guyer R, et al. Role of hydrogen bonding in hysteresis observed in sorption-induced swelling of soft nanoporous polymers. Nat Commun. 2018;9(1):1–7.
- Diez-Pena E, Quijada-Garrido I, Barrales-Rienda JM. Hydrogen-bonding effects on the dynamic swelling of P (N-iPAAm-co-MAA) copolymers. A case of autocatalytic swelling kinetics. Macromolecules. 2002;35(23):8882–8888.