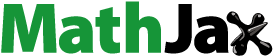
Abstract
The occurrence of the Ms6.8 Luding earthquake on 5 September 2022 filled the seismic gap in the southeastern segment of the Xianshuihe fault zone and transferred stress to the surrounding faults, which attracted much more attention to the seismic hazard. In this study, we calculate the coseismic Coulomb stress changes caused by the Ms6.8 Luding earthquake and analyze its relationship with the relocated aftershocks, and further explore the stress evolution before the Luding earthquake and seismic hazards on the surrounding faults after it. The results show significant coseismic Coulomb stress increases in the northern Anninghe fault zone and the coseismic unruptured section of the southeastern Xianshuihe fault zone. Aftershocks are highly correlated with the positive coseismic Coulomb stress change, wherein eight out of eleven aftershocks of M ≥ 3.0 occurred in areas with significant stress increases ranging from 0.03 MPa to 0.44 MPa, indicating the triggering effect. The Moxi fault experiences co- and post-seismic Coulomb stress changes due to historical M ≥ 7.0 strong earthquakes since 1515, which help understand the seismogenic mechanism of the Luding earthquake. Moreover, the Luding earthquake advanced the potential earthquakes in the northern section of the Anninghe fault zone by approximately 60 years, further highlighting the potential seismic hazards.
1. Introduction
On 5 September 2022, a Ms6.8 earthquake struck Luding County in Sichuan Province, Western China. According to the China Earthquake Network Center, the epicenter (102.08°E, 29.59°N) was located on the Moxi fault in the southeastern segment of the Xianshuihe Fault (), with a hypocenter depth of 16 km. The focal mechanism was dominated by left-lateral strike-slip faulting with a high dip angle (). Seismic inversion indicated that the earthquake was a unilateral rupture event that propagated toward the southeast, and extended approximately 55 km (Zhang et al. Citation2023b). Aftershock relocation showed that the aftershocks were distributed in three clusters and concentrated at depths of 5–20 km (), and the focal mechanism solutions of M ≥ 3.0 aftershocks were dominated by left-lateral strike-slip motion (Guo et al. Citation2023, Yang et al. Citation2022, Yi et al. Citation2023). The occurrence of the Ms6.8 Luding earthquake filled the seismic gap that had lasted for more than 200 years in the southeastern segment of the Xianshuihe fault zone (Wen et al. Citation2008) and meanwhile transferred stress to the surrounding faults (Stein Citation1999), which made the seismic hazard of the surrounding faults attract much more attention.
Figure 1. Tectonic setting of the Xianshuihe fault and its adjacent region. (a) The black beach balls represent the focal mechanism solutions of the historic M ≥ 7.0 earthquakes since 1515 (Li et al. Citation2020), while the green beach ball represents the focal mechanism solution of the Ms6.8 Luding earthquake. (b) Aftershocks (until 29 September 2022) of the Luding earthquake are displayed in different colors (Zhang et al. Citation2023a).
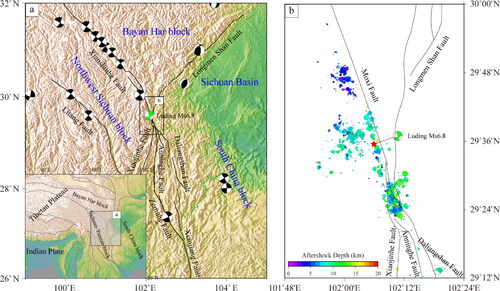
Table 1. Focal mechanism solutions of the Ms6.8 Luding earthquake.
The Coulomb stress change has been used previously to explore the relationship between main- and aftershocks and to assess the seismic hazard of the surrounding faults following strong earthquakes (King et al. Citation1994; Stein et al. Citation1997; Papadimitriou et al. Citation2004; Wan and Shen Citation2010; Xiong et al. Citation2010; He et al. Citation2011; Gupta et al. Citation2015; Shan et al. Citation2015; Shao et al. Citation2016; Gunawan et al. Citation2018; Citation2019; Li, Huang et al. Citation2021). Xu et al. (Citation2013) analyzed the stress evolution of the Xianshuihe Fault associated with the historical M ≥ 6.7 earthquakes since 1893 and showed a significant Coulomb stress increase (≥0.1 MPa) of the Moxi section of the southeastern Xianshuihe Fault. Considering more historical strong earthquakes, Li et al. (Citation2020) calculated the stress change caused by M ≥ 7.0 earthquakes in the Sichuan-Yunnan region and the surrounding great M ≥ 8.0 earthquakes since the M7.8 Yongsheng earthquake in 1515 and found that the Luding earthquake occurred on the Moxi fault with a significant increase of the Coulomb stress. These studies underline the importance of stress transfer and further confirm the effectiveness of the Coulomb stress change in deciphering the potential fault rupture plane with high seismic hazards (Stein et al. Citation1997; Stein Citation1999; Nalbant et al. Citation2002). In this regard, our concern is how the Coulomb stress change associated with the Ms6.8 Luding earthquake will affect potential seismic hazards on the surrounding faults.
To address the above questions, based on elastic dislocation theory and the stratified viscoelastic model and constrained by the coseismic rupture model of the Ms6.8 Luding earthquake, we employ the PSGRN/PSCMP program (Wang et al. Citation2006) to calculate the coseismic Coulomb stress change and explore its relationship with aftershocks. Combined with the cumulative Coulomb stress change caused by strong historical earthquakes, we discuss the seismogenic mechanism of the Ms6.8 Luding earthquake and the change in seismic hazards of the surrounding faults after it occurred, which provides a basis for analysis of the potential rupture plane of the eastern boundary faults of the Sichuan-Yunnan block.
2. Tectonic setting
With the continuous collision between the Indian and Eurasian plates, the Sichuan-Yunnan rhombic block, the Bayan Har block, and the South China block show significant kinematic differences, as indicated by the high strike-slip rate and shear strain rate of the block-boundary Xianshuihe-Xiaojiang fault system (Xu et al. Citation2003; Bai et al. Citation2018; Li et al. Citation2019; Wang et al. Citation2021). The Ms6.8 Luding earthquake occurred on the Moxi fault in the southeastern segment of the Xianshuihe Fault (Shan et al. Citation2023). To the southeast side of the Moxi fault is the strong South China block, while to the west side of the Moxi fault is the northwestern Sichuan block, where the large-scale eastward escape of the plateau occurs (Tapponnier et al. Citation1982; Zhang et al. Citation2003; Citation2022). The velocity structure shows that the low velocity develops in the mid-lower crust of the northwestern Sichuan block (Liu et al. Citation2014), and the lateral velocity difference between the two sides of the Moxi fault is obvious at a depth of 15 km (Han et al. Citation2022; Song et al. Citation2023; Li et al. Citation2023b). Meanwhile, the magnetotelluric electrical structure also shows that there is a low-resistivity anomaly zone in the mid-lower crust on the west side of the fault and a high-resistivity body on the east side, and the Ms6.8 Luding earthquake occurred in the transition zone (Jiang et al. Citation2022). The significant lateral heterogeneity of the velocity structure benefits the rapid accumulation of the stress-strain energy in the transition zone, providing an ideal environment for the generation of large earthquakes (Dong and Yang Citation2022; Zhang, Li et al. Citation2022).
The strain rate fields show that the Xianshuihe-Xiaojiang fault system demonstrates a high maximum shear strain rate of 60 ∼ 80 nanostrain/yr (Li et al. Citation2019; Wang and Shen Citation2020), and the maximum shear strain rate on the Moxi fault gradually increased before the Ms6.8 Luding earthquake (Li, Wu et al. Citation2022). The modeled tectonic stress field shows that the stress state on the Xianshuihe fault zone exhibits obvious inhomogeneity, and the change in the fault strike causes a higher stress build-up on the Moxi fault (Li, Gao et al. 2022). Additionally, the frequent strong earthquakes on the Xianshuihe Fault, including the 1786 M73/4 Moxi earthquake (Wen et al. Citation2008), cause significant Coulomb failure stress changes (≥0.01 MPa) on the Moxi fault (Li et al. Citation2020), which jointly control the occurrence of earthquakes along the Moxi fault.
3. Method and model
3.1. Coulomb stress change
The Coulomb stress change was calculated based on the concept of a critical Coulomb failure stress on a receiver fault (Harris Citation1998)
(1)
(1)
where
is the shear stress change (positive in the fault slip direction),
is the normal stress change (positive for fault unclamping), and
is the apparent friction coefficient, which varies from 0 to 0.8 (King et al. Citation1994). In this study, we assume the apparent friction coefficient
=0.4 (Xiong et al. Citation2010) and employ the PSGRN/PSCMP program (Wang et al. Citation2006) to calculate the stress change at different depths on the adjacent receiving fault plane and discuss the possible influence from the different apparent friction coefficients.
3.2. Stratified viscoelastic model
Based on the deep seismic sounding profile across the west Sichuan region and the viscosity inversions (Shen et al. Citation2003; Wang et al. Citation2003; Shao et al. Citation2008; Shi and Cao Citation2008), we determined the thickness of the crustal layers, the density, the velocity structures, and the transient and steady-state viscosities, as shown in , which are consistent with Li et al. (Citation2020). In contrast to the linear Maxwell model, which overestimates the contribution of the post-seismic viscoelastic relaxation effect when the transient viscosity is used to represent the rheology of the lower crust and upper mantle, and underestimates it when the steady-state viscosity is used (Pratama et al. Citation2017; Shi et al. Citation2020), we choose the Burgers model since it can well simulate the transient elastic response, the short exponentially decaying time response, and the long linearly increasing time response (Wang et al. Citation2012; Shao et al. Citation2016; Pratama et al. Citation2017).
3.3. Coseismic rupture model and fault parameters
After the Ms6.8 Luding earthquake, based on the broadband waveforms and finite fault model, Zhang et al. (Citation2023a) determined the coseismic slip distribution. The results show that this earthquake occurred on a dominant left-lateral strike-slip fault, with the slip concentrated at a depth of 5–10 km and the peak slip reached 1.5 m (Guo et al. Citation2023). Geometric parameters (strike/dip/rake) of the receiving faults were determined based on field investigations and focal mechanism solutions of historic earthquakes (see Table S1 for details), which are consistent with Li et al. (Citation2020).
4. Results
4.1. Coulomb stress change and coseismic displacement
Based on the coseismic slip distribution of the Ms6.8 Luding earthquake, we calculate the coseismic Coulomb stress change when the apparent friction coefficient is 0.4 (). From the Coulomb stress changes at depths of 5, 10, 15, and 20 km, we find that the patterns of the Coulomb stress change at different depths are similar. Owing to the concentrated coseismic slip, the coseismic stress change is greater at depths of 5–10 km than at depths of 15–20 km. At a depth of 10 km, the occurrence of the Ms6.8 Luding earthquake caused a significant coseismic stress increase in the northern segment of the Anninghe fault zone, the unruptured section of the southeastern segment of the Xianshuihe fault zone, the southwest segment of the Longmenshan fault zone, and the northern segment of the Xiaojinhe fault zone. Specifically, the maximum coseismic Coulomb stress change in the Xianshuihe fault zone reached 0.9 MPa, 0.15 MPa in the northern section of the Anninghe fault zone, 0.1 MPa in the northern section of the Xiaojinhe fault zone, and 0.05 MPa in the southwest segment of the Longmenshan fault zone. The coseismic stress changes in these fault zones all exceeded the threshold of 0.01 MPa, which suggests a triggering effect on potential earthquakes, which further highlights the seismic hazards of strong earthquakes on these faults. In contrast, the Ms6.8 Luding earthquake also casts negative Coulomb stress on the northern segment of the Daliangshan fault zone, the Mabian fault zone, and the Litang fault zone, delaying the occurrence of strong earthquakes on the faults.
Furthermore, we analyze the postseismic Coulomb stress change on the fault plane at 10 km depth by integrating the viscoelastic relaxation of the lower crust and upper mantle. Our results show a significant postseismic stress increase in the northern segment of the Anninghe fault zone, the unruptured section of the southeastern segment of the Xianshuihe fault zone, and the northern segment of the Xiaojinhe fault zone (). Meanwhile, the postseismic Coulomb stress change gradually increases following the Luding earthquake, indicating that the postseismic effect transfers more viscoelastic stress to these faults with time and further increases the seismic hazard on them.
Figure 4. The postseismic Coulomb stress change on the fault plane at a depth of 10 km. (a) and (b) represent the postseismic Coulomb stress changes 10 and 50 years after the Ms6.8 Luding earthquake, respectively.
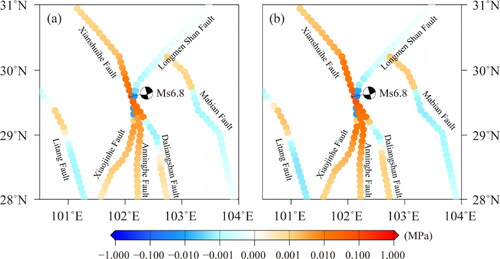
Additionally, the modeled coseismic displacement shows that the direction around the Xianshuihe Fault zone is predominantly SE and EW (), which is consistent with the tectonic motion reflected by the GPS observations, suggesting that the occurrence of the Ms6.8 Luding earthquake promoted stress accumulation on the Xianshuihe Fault and the Anninghe Fault due to the consistency between the direction of the modeled displacement and the real GPS observations. These inherited kinematics support the additional stress buildup on the Xianshuihe Fault and the Anninghe Fault faults, as indicated by the significant coseismic stress increase on these faults (). In contrast, the coseismic displacement and GPS observations show the opposite direction around the northern segment of the Daliangshan Fault and the Mabian Fault, suggesting that the occurrence of the Ms6.8 Luding earthquake reduced the stress level, as indicated by the coseismic stress change decrease shown in .
Figure 5. Coseismic displacement caused by the Ms6.8 Luding earthquake. The GPS data are from Wang and Shen (Citation2020). the dashed green line represents the surface fault trace of the Luding earthquake.
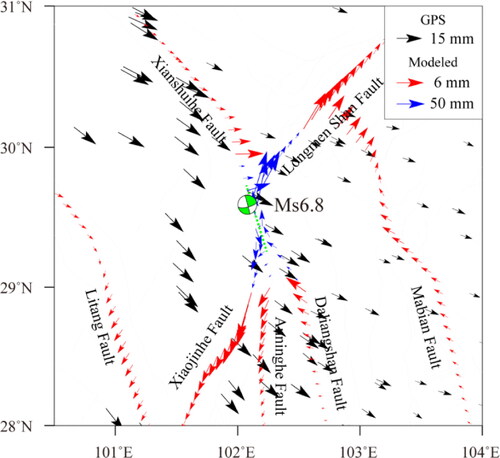
4.2. Relations between the mainshock and aftershocks
We calculate the coseismic Coulomb stress change at a depth of 10 km, by projecting the stress tensors on the fault plane of the mainshock and find that the distribution of aftershocks is highly correlated with the positive coseismic Coulomb stress change (), especially the two clusters of aftershocks located outward of the rupture fault. In particular, we analyze the possible relation between the mainshock and the eleven M ≥ 3.0 aftershocks by further projecting the stress tensor on the principal nodal plane of the focal mechanism solution of these aftershocks (). The results show that eight out of the eleven aftershocks occurred in the coseismic Coulomb stress increase region and the Coulomb stress change varies by 0.03 MPa∼0.44 MPa, which is greater than the triggering threshold of 0.01 MPa (King et al. Citation1994), suggesting that the main shock triggers the occurrence of these aftershocks. However, for the other three events closely spatially located to the rupture plane of the mainshock, they show negative coseismic Coulomb stress changes, which may reflect the delayed ongoing strain energy release of the rupturing fault.
Figure 6. Coseismic Coulomb stress change at the hypocenter of the aftershocks caused by the Ms6.8 Luding earthquake. (a) Coseismic Coulomb stress change caused by the Ms6.8 Luding earthquake. The black circles show the relocated aftershocks that occurred within 5 days after the mainshock from Zhang et al. (Citation2023a). (b) The green lines on the beach ball show the rupture plane, and the color denotes the Coulomb stress changes at the epicenter of the M ≥ 3.0 aftershocks of the Ms6.8 Luding earthquake (Yang et al. Citation2022).
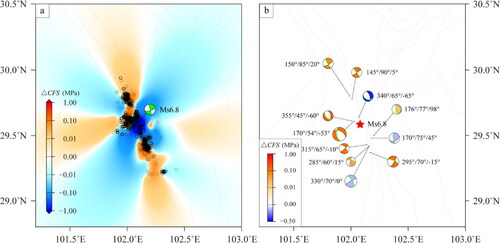
5. Discussion
The occurrence of earthquakes transfers stress to the surrounding faults, thereby adjusting the Coulomb stress changes on the surrounding faults and helping to delineate segments where large earthquakes may potentially occur (Harris Citation1998; Stein Citation1999). However, the Coulomb stress change is dependent on the apparent friction coefficient (Xu et al. Citation2020), which influences the stability of the potential seismic hazard analysis.
5.1. Robustness of the Coulomb stress change
According to formula (1), the Coulomb stress change is dependent on the normal stress change, shear stress change, and apparent friction coefficient. In previous studies, for a large-scale strike-slip fault, the apparent friction coefficient is assumed to be lower, even less than 0.1 (He et al. Citation2009; Zhu et al. Citation2022), far lower than the empirical value of 0.4 ∼ 0.6 (King et al. Citation1994; Xiong et al. Citation2010). In this study, to ascertain the possible influence of the different apparent friction coefficients () and the robustness of the Coulomb stress change, we calculate the coseismic Coulomb stress change on the fault plane at a depth of 10 km assuming different
values of 0.2, 0.4, 0.6 and 0.8 () and further analyze the independent normal and shear stress change on the fault plane (). The results show that the stress patterns with the lower
(0.2) are very similar to those calculated with a higher
(0.8). The stress pattern remains unaffected, without allowing for the discrimination of the different values of
which is in agreement with previous studies (Papadimitriou Citation2002; Li, Huang et al. Citation2021). In particular, the significant coseismic shear stress change is distributed in the northern segment of the Anninghe fault zone, the unruptured section of the southeastern segment of the Xianshuihe fault zone, the southwestern segment of the Longmenshan fault zone, and the northern segment of the Xiaojinhe fault zone (), which is much larger than the coseismic normal stress change (). In this scenario, the effective normal stress change contributes less to the Coulomb stress change, and the positive Coulomb stress change on the fault plane is mainly controlled by the shear stress change. According to the Coulomb failure criterion, we can conclude that the assumed different apparent friction coefficients do not affect the polarity of the Coulomb stress change associated with the Ms6.8 Luding earthquake, as shown in .
Figure 7. Coseismic Coulomb stress change caused by the Ms6.8 Luding earthquake on the fault plane at a depth of 10 km with different apparent friction coefficients
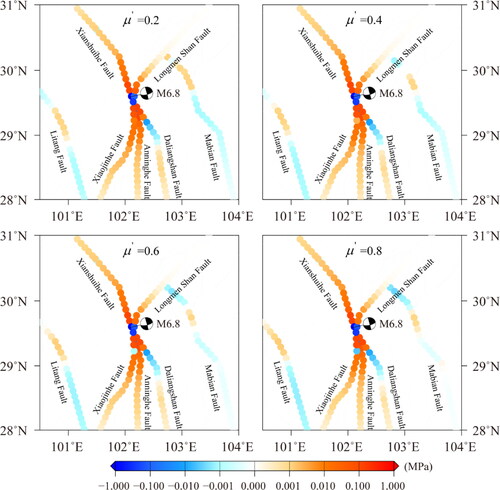
Figure 8. Normal stress change, shear stress change, and principal stress distribution associated with the Ms6.8 Luding earthquake at a depth of 10 km. The arrows represent the principal stress.
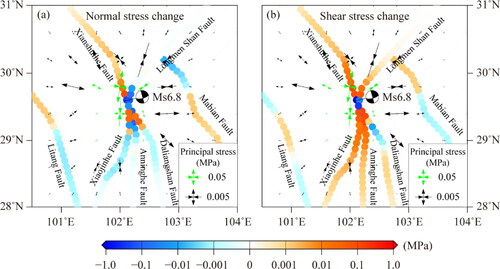
Meanwhile, the principal stress regime associated with the Ms6.8 Luding earthquake shows that the coseismic maximum principal compressive stress rotated clockwise from the NE direction to the nearly NS direction along the Xianshuihe-Anninghe fault system (), as also indicated by the P and T axes of these aftershocks (Yi et al. Citation2023). This subsidiary coseismic stress regime benefits the left-lateral strike-slip motion of these faults, and the coseismic shear stress change on the fault plane is consistent with that derived from the tectonic loading, suggesting a higher shear stress level on the fault plane than before the Ms6.8 Luding earthquake.
It should be noted that, since the postseismic afterslip model is unavailable, we do not discuss its influence on the Coulomb stress change of the fault plane. Nevertheless, previous studies have shown that the afterslip distribution has a complementary pattern to the coseismic slip distribution (He et al. Citation2021; Xiong et al. Citation2022; Fauziyyah et al. Citation2023), and both show similar slip behaviors (Freed et al. Citation2017) and generate consistent stress change patterns to the surrounding faults. In this scenario, we can determine that the sign of the Coulomb stress change on the fault plane is stable.
5.2. Implications for the seismic hazard of the Anninghe fault
Multidisciplinary data, including geological seismic gaps, geodetic locking rates, scares of small seismicity, and Coulomb stress changes, have been incorporated into the assessment of the potential rupture regions with high seismic hazards (Shao et al. Citation2023). The occurrence of the Ms6.8 Luding earthquake filled the seismic gap that had lasted for more than 200 years in the southeastern segment of the Xianshuihe fault zone (Wen et al. Citation2008; Qiao and Zhou Citation2021) and broke the 41-year quiescence of M ≥ 6.7 earthquakes on the eastern boundary of the Sichuan-Yunnan block, which attracted more attention to the seismic hazards of surrounding active faults. Our modeled coseismic Coulomb stress change shows significant increases (≥0.01 MPa) in the northern segment of the Anninghe fault zone, the coseismic unruptured section of the southeastern segment of the Xianshuihe fault zone, the southwest segment of the Longmenshan fault zone, and the northern segment of the Xiaojinhe fault zone.
The Anninghe fault zone experienced historical seismic activity but has remained quiescent since the M63/4 Mianning earthquake in 1952 (Wen et al. Citation2008). The low b value and the relocation of small earthquakes show that this fault is a first-order seismic gap with a high level of background stress (Yi et al. Citation2008). Paleoseismic investigation reveals that the elapsed time of the strong earthquakes in the northern section of the Anninghe fault zone is close to or even exceeds the average recurrence interval revealed by historical strong earthquakes (Ran et al. Citation2008; Wang et al. Citation2017; Xu et al. Citation2017). Geodetic inversion shows high locking rates and slip deficits along the Anninghe fault zone (Jiang et al. Citation2015; Li et al. Citation2021; Citation2023a). Stress modeling indicates that the background stress level in the northern section of the Anninghe fault zone is higher (Xu and Zeng Citation2022), and the strong historical earthquakes before the Ms6.8 Luding earthquake further caused a significant stress increase in the southeastern segment of the Xianshuihe fault zone and the Anninghe fault zone (Li et al. Citation2020) (). Moreover, the occurrence of the Ms6.8 Luding earthquake further cast stress loading on the Anninghe fault zone (), and the likelihood for the failure of this gap is even higher than before 2022. A previous study showed that the annual interseismic tectonic loading rate along the Anninghe fault zone is ∼2500 Pa/a (Shan et al. Citation2013). Assuming the Anninghe fault zone fits the characteristic earthquake recurrence pattern, the occurrence of the Ms6.8 Luding earthquake advanced the occurrence of earthquakes in the northern section of the Anninghe fault zone by approximately 60 years.
Figure 9. Cumulative Coulomb stress change on the fault plane of the 2022 Ms6.8 Luding earthquake caused by historic M ≥ 7.0 strong earthquakes since 1515 (Li et al. Citation2020). The stress tensors were projected on the fault plane at a depth of 10 km.
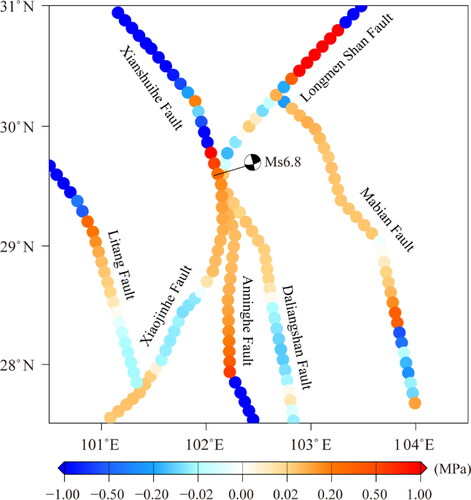
Meanwhile, some studies demonstrate that as the Daliangshan fault zone gradually matures, it will replace the Anninghe and Zemuhe fault zones in the Xianshuihe-Xiaojiang fault system, and the two fault zones will probably die out with continuous clockwise rotation (He et al. Citation2008). Numerical modeling also shows that the interaction between the Anninghe-Zemuhe fault and the Daliangshan fault zone makes the strain transfer to the Daliangshan fault zone from the previous strain-concentrated Anning-Zemuhe fault zones (Wang et al. Citation2010). However, once the Daliangshan fault matures, it will absorb significant strain energy and cause less strain energy accumulation on the Mabian fault located east of the Daliangshan fault, which makes it difficult to explain its strong historical seismic activity (Zhu et al. Citation2022), suggesting that the Anninghe fault zone is still the eastern boundary fault of the Sichuan-Yunnan block. Consequently, we highlight the considerable seismic hazard in the Anninghe fault zone.
6. Conclusions
We calculate the coseismic Coulomb stress changes associated with the Ms6.8 Luding earthquake and explore the possible relationship between the mainshock and aftershocks of the Ms6.8 Luding earthquake, the stress evolution before the Ms6.8 Luding earthquake, and the seismic hazards on the surrounding faults following it. Our results show that the occurrence of the Ms6.8 Luding earthquake caused significant Coulomb stress increases in the northern segment of the Anninghe fault zone, the unruptured section of the southeastern segment of the Xianshuihe fault zone, the southwest segment of the Longmenshan fault zone, and the northern segment of the Xiaojinhe fault zone, all of which exceed 0.01 MPa. In contrast, the coseismic stress decreased in the northern segment of the Daliangshan fault. Aftershocks were highly correlated with the positive coseismic Coulomb stress change, wherein eight out of the eleven aftershocks of M ≥ 3.0 occurred in areas with significant coseismic Coulomb stress increases, indicating the triggering effect between the mainshock and aftershocks. The Ms6.8 Luding earthquake occurred in the section with significant cumulative Coulomb stress changes caused by strong historical earthquakes, and the occurrence of earthquakes in the northern section of the Anninghe fault zone advanced approximately 60 years. These results help in the understanding of the seismogenic mechanism of the Ms6.8 Luding earthquake and highlight the potential seismic hazards around the eastern boundary of the Sichuan-Yunnan block.
Supplemental Material
Download MS Word (28.3 KB)Acknowledgments
The authors thank Fuqiang Shi and Lianwang Chen for their helpful discussion to improve the manuscript and Xu Zhang for providing the coseismic rupture model of the Luding earthquake. All figures in this paper were plotted using the GMT software package (Wessel et al. Citation2013).
Disclosure statement
No potential conflict of interest was reported by the author(s).
Data availability statement
The data used in this article are available from the corresponding author (Yujiang Li) upon request.
Additional information
Funding
References
- Bai M, Chevalier M-L, Pan J, Replumaz A, Leloup PH, Métois M, Li H. 2018. Southeastward increase of the late Quaternary slip-rate of the Xianshuihe fault, eastern Tibet. Geodynamic and seismic hazard implications. Earth Planet Sci Lett. 485:19–31. doi:10.1016/j.epsl.2017.12.045.
- Dong X, Yang D. 2022. Positions of large earthquakes revealed from velocity heterogeneities and radial anisotropy in the eastern Tibetan Plateau. Sci Bull. 67(20):2026–2029. doi:10.1016/j.scib.2022.09.019.
- Fauziyyah, Risya, Gunawan, Endra, Widiyantoro, Sri, Meilano, Irwan, Syamsuddin,. 2023. Early postseismic deformation of the 2018 Lombok, Indonesia, earthquake sequence constrained by GPS data. J Geodyn.156:101971. doi:10.1016/j.jog.2023.101971.
- Freed AM, Hashima A, Becker TW, Okaya DA, Sato H, Hatanaka Y. 2017. Resolving depth-dependent subduction zone viscosity and afterslip from postseismic displacements following the 2011 Tohoku-oki, Japan earthquake. Earth Planet Sci Lett. 459:279–290. doi:10.1016/j.epsl.2016.11.040.
- Gunawan E, Widiyantoro S, Marliyani GI, Sunarti E, Ida R, Gusman AR. 2019. Fault source of the 2 September 2009 Mw 6.8 Tasikmalaya intraslab earthquake, Indonesia: analysis from GPS data inversion, tsunami height simulation, and stress transfer. Phys Earth Planet Inter. 291:54–61. doi:10.1016/j.pepi.2019.04.004.
- Gunawan E, Widiyantoro S, Rosalia S, Daryono MR, Meilano I, Supendi P, Ito T, Tabei T, Kimata F, Ohta Y, et al. 2018. Coseismic Slip Distribution of the 2 July 2013 Mw 6.1 Aceh, Indonesia, Earthquake and Its Tectonic Implications. Bull Seismol Soc Am. 108(4):1918–1928. doi:10.1785/0120180035.
- Guo R, Li L, Zhang W, Zhang Y, Tang X, Dai K, Li Y, Zhang L, Wang J. 2023. Kinematic slip evolution during the 2022 Ms 6.8 Luding, China, Earthquake: compatible With the Preseismic Locked Patch. Geophys Res Lett. 50:e2023GL103164.
- Gupta DK, Bhowmick D, Roy PNS. 2015. Himalayan hazard study on the basis of stress and strain state of 1991 Uttarkashi earthquake using coulomb stress transfer model. Geomatics Nat Hazards Risk. 6(2):131–148. doi:10.1080/19475705.2013.820797.
- Han S, Zhang H, Xin H, Shen W, Yao H. 2022. USTClitho2.0: updated Unified Seismic Tomography Models for Continental China Lithosphere from Joint Inversion of Body‐Wave Arrival Times and Surface‐Wave Dispersion Data. Seismol Res Lett. 93(1):201–215. doi:10.1785/0220210122.
- Harris RA. 1998. Introduction to special section: stress triggers, stress shadows, and implications for seismic hazard. J Geophys Res. 103(B10):24347–24358. doi:10.1029/98JB01576.
- He H, Ikeda Y, He Y, Togo M, Chen J, Chen C, Tajikara M, Echigo T, Okada S. 2008. Newly-generated Daliangshan fault zone-Shortcutting on the central section of Xianshuihe-Xiaojiang fault system. Sci China Ser D-Earth Sci. 51(9):1248–1258. doi:10.1007/s11430-008-0094-4.
- He J, Lu S, Wang X. 2009. Mechanical relation between crustal rheology, effective fault friction, and strike-slip partitioning among the Xiaojiang fault system, southeastern Tibet. J Asian Earth Sci. 34(3):363–375. doi:10.1016/j.jseaes.2008.06.003.
- He J, Xia W, Lu S, Qian H. 2011. Three-dimensional finite element modeling of stress evolution around the Xiaojiang fault system in the southeastern Tibetan plateau during the past ∼500 years. Tectonophysics. 507(1-4):70–85. doi:10.1016/j.tecto.2011.05.009.
- He L, Feng G, Wu X, Lu H, Xu W, Wang Y, Liu J, Hu J, Li Z. 2021. Coseismic and early postseismic slip models of the 2021 Mw 7.4 Maduo Earthquake (Western China) estimated by space-based geodetic data. Geophys Res Lett. 48:e2021–GL095860.
- Jiang F, Chen X, Unsworth MJ, Cai J, Han B, Wang L, Dong Z, Cui T, Zhan Y, Zhao G. 2022. Mechanism for the Uplift of Gongga Shan in the Southeastern Tibetan Plateau Constrained by 3D Magnetotelluric Data. Geophys Res Lett. 49:e2021–GL097394.
- Jiang G, Xu X, Chen G, Liu Y, Fukahata Y, Wang H, Yu G, Tan X, Xu C. 2015. Geodetic imaging of potential seismogenic asperities on the Xianshuihe-Anninghe-Zemuhe fault system, southwest China, with a new 3-D viscoelastic interseismic coupling model. JGR Solid Earth. 120(3):1855–1873. doi:10.1002/2014JB011492.
- King GC, Stein RS, Lin J. 1994. Static stress changes and the triggering of earthquakes. Bull Seismol Soc Am. 84:935–953.
- Li L, Wu Y, Li Y, Zhan W, Liu X. 2022. Dynamic deformation and fault locking of the Xianshuihe Fault Zone, Southeastern Tibetan Plateau: implications for seismic hazards. Earth Planets Space. 74(1):35. doi:10.1186/s40623-022-01591-9.
- Li X, Gao K, Feng Y, Zhang C. 2022. 3D geomechanical modeling of the Xianshuihe fault zone, SE Tibetan Plateau: implications for seismic hazard assessment. Tectonophysics. 839:229546. doi:10.1016/j.tecto.2022.229546.
- Li Y, Nocquet JM, Shan X, Jian H. 2021. Heterogeneous interseismic coupling along the Xianshuihe‐Xiaojiang fault system. Eastern Tibet J Geophys Res. 126:e2020JB021187.
- Li Y, Shan X, Gao Z, Huang X. 2023a. Interseismic coupling, asperity distribution and earthquake potential on major faults in southeastern tibet. Geophys Res Lett. 50:e2022GL101209.
- Li Y, Tian J, Li X, Li S, Wang Q, Gao Y. 2023b. Deep tectonic pattern of the Luding MS6.8 earthquake on 5th September 2022 in Sichuan Province, China. Chinese Journal of Geophysics (in Chinese). 66:1385–1396.
- Li Y, Huang L, Ding R, Yang S, Liu L, Zhang S, Liu H. 2021. Coulomb stress changes associated with the M7.3 Maduo earthquake and implications for seismic hazards. Natural Hazards Res. 1(2):95–101. doi:10.1016/j.nhres.2021.06.003.
- Li Y, Liu M, Li Y, Chen L. 2019. Active crustal deformation in southeastern Tibetan Plateau: the kinematics and dynamics. Earth Planet Sci Lett. 523:115708. doi:10.1016/j.epsl.2019.07.010.
- Li Y, Shi F, Zhang H, Wei W, Xu J, Shao Z. 2020. Coulomb stress change on active faults in Sichuan-Yunnan region and its implications for seismic hazard. Seismol Geol. 42:526–546.
- Liu Q, van der Hilst RD, Li Y, Yao H, Chen J, Guo B, Qi S, Wang J, Huang H, Li S, et al. 2014. Eastward expansion of the Tibetan Plateau by crustal flow and strain partitioning across faults. Nature Geosci. 7(5):361–365. doi:10.1038/ngeo2130.
- Nalbant SS, McCloskey J, Steacy S, Barka AA. 2002. Stress accumulation and increased seismic risk in eastern Turkey. Earth Planet Sci Lett. 195(3-4):291–298. doi:10.1016/S0012-821X(01)00592-1.
- Papadimitriou E, Wen X, Karakostas V, Jin X. 2004. Earthquake triggering along the Xianshuihe fault zone of western Sichuan, China. Pure Appl Geophys. 161(8):1683–1707. doi:10.1007/s00024-003-2471-4.
- Papadimitriou EE. 2002. Mode of strong earthquake recurrence in the Central Ionian Islands (Greece): possible triggering due to coulomb stress changes generated by the occurrence of previous strong shocks. Bull Seismol Soc Am. 92(8):3293–3308. doi:10.1785/0120000290.
- Pratama C, Ito T, Sasajima R, Tabei T, Kimata F, Gunawan E, Ohta Y, Yamashina T, Ismail N, Nurdin I, et al. 2017. Transient rheology of the oceanic asthenosphere following the 2012 Indian Ocean Earthquake inferred from geodetic data. J Asian Earth Sci. 147:50–59. doi:10.1016/j.jseaes.2017.07.049.
- Qiao X, Zhou Y. 2021. Geodetic imaging of shallow creep along the Xianshuihe fault and its frictional properties. Earth Planet Sci Lett. 567:117001. doi:10.1016/j.epsl.2021.117001.
- Ran Y, Chen L, Cheng J, Gong H. 2008. Late Quaternary surface deformation and rupture behavior of strong earthquake on the segment north of Mianning of the Anninghe fault. Sci China Ser D-Earth Sci. 51(9):1224–1237. doi:10.1007/s11430-008-0104-6.
- Shan B, Xiong X, Wang R, Zheng Y, Yadav RBS. 2015. Stress evolution and seismic hazard on the Maqin-Maqu segment of East Kunlun Fault zone from co-, post-and interseismic stress changes. Geophys J Int. 200(1):244–253. doi:10.1093/gji/ggu395.
- Shan B, Xiong X, Wang R, Zheng Y, Yang S. 2013. Coulomb stress evolution along Xianshuihe–Xiaojiang Fault System since 1713 and its interaction with Wenchuan earthquake, May 12, 2008. Earth Planet Sci Lett. 377-378:199–210. doi:10.1016/j.epsl.2013.06.044.
- Shan X, Li Y, Gao Z, Hua J, Huang X, Gong W, Qu C, Zhao D, Chen J, Huang C, et al. 2023. Coseismic deformation of the 2022 Luding Ms6.8 earthquake and seismic potential along adjacent major faults. Chin Sci Bull. 68(8):944–953. doi:10.1360/TB-2022-0954.
- Shao Z, Wu Y, Ji L, Diao F, Shi F, Li Y, Long F, Zhang H, Wang W, Wei W, et al. 2023. Assessment of strong earthquake risk in the Chinese mainland from 2021 to 2030. Earthquake Res Adv. 3(1):100177. doi:10.1016/j.eqrea.2022.100177.
- Shao ZG, Fu R, Xue T, Huang J. 2008. Numerical simulation and discussion on the mechanism of postseismic deformation after Kunlun Ms8.1 earthquake. Chin J Geophys. 51:805–816.
- Shao Z, Xu J, Ma H, Zhang L. 2016. Coulomb stress evolution over the past 200years and seismic hazard along the Xianshuihe fault zone of Sichuan, China. Tectonophysics. 670:48–65. doi:10.1016/j.tecto.2015.12.018.
- Shen Z, Wan Y, Gan W, Zeng Y, Ren Q. 2003. Viscoelastic Triggering Between Large Earthquakes along the East Kunlun Fault System. Chinese J Geophys. 46(6):1125–1138. doi:10.1002/cjg2.432.
- Shi F, Zhang H, Shao Z, Xu J, Shao H, Li Y. 2020. Coulomb stress evolution and stress interaction among strong earthquakes in North China. Chin J Geophys. 63:3338–3354.
- Shi Y, Cao J. 2008. Effective viscosity of China continental lithosphere. Earth Sci Front. 15(3):82–95. doi:10.1016/S1872-5791(08)60064-0.
- Song X, Lei J, Zou K. 2023. The 2022 Luding, Sichuan, China, M 6.8 earthquake: a fluid-related earthquake? J Asian Earth Sci. 105543. doi:10.1016/j.jseaes.2023.105543.
- Stein RS. 1999. The role of stress transfer in earthquake occurrence. Nature. 402(6762):605–609. doi:10.1038/45144.
- Stein RS, Barka AA, Dieterich JH. 1997. Progressive failure on the North Anatolian fault since 1939 by earthquake stress triggering. Geophys J Int. 128(3):594–604. doi:10.1111/j.1365-246X.1997.tb05321.x.
- Tapponnier P, Peltzer G, Le Dain AY, Armijo R, Cobbold P. 1982. Propagating extrusion tectonics in Asia: new insights from simple experiments with plasticine. Geol. 10(12):611–616. doi:10.1130/0091-7613(1982)10<611:PETIAN>2.0.CO;2.
- Wan Y, Shen Z. 2010. Static Coulomb stress changes on faults caused by the 2008 Mw 7.9 Wenchuan, China earthquake. Tectonophysics. 491(1-4):105–118. doi:10.1016/j.tecto.2010.03.017.
- Wang C, Chan WW, Mooney WD. 2003. Three‐dimensional velocity structure of crust and upper mantle in southwestern China and its tectonic implications. J Geophys Res. 108(B9). doi:10.1029/2002JB001973.
- Wang H, Liu J, Shen X, Liu M, Li Q, Shi Y, Zhang G. 2010. Influence of fault geometry and fault interaction on strain partitioning within western Sichuan and its adjacent region. Sci China Earth Sci. 53(7):1056–1070. doi:10.1007/s11430-010-3062-8.
- Wang H, Ran Y, Chen L, Li Y. 2017. Paleoearthquakes on the Anninghe and Zemuhe fault along the southeastern margin of the Tibetan Plateau and implications for fault rupture behavior at fault bends on strike-slip faults. Tectonophysics. 721:167–178. doi:10.1016/j.tecto.2017.08.030.
- Wang K, Hu Y, He J. 2012. Deformation cycles of subduction earthquakes in a viscoelastic Earth. Nature. 484(7394):327–332. doi:10.1038/nature11032.
- Wang M, Shen Z. 2020. Present-Day Crustal Deformation of Continental China Derived From GPS and Its Tectonic Implications. J Geophys Res. 125:e2019JB018774.
- Wang R, Lorenzo-Martín F, Roth F. 2006. PSGRN/PSCMP—a new code for calculating co-and post-seismic deformation, geoid and gravity changes based on the viscoelastic-gravitational dislocation theory. Comp Geosci. 32(4):527–541. doi:10.1016/j.cageo.2005.08.006.
- Wang W, Qiao X, Ding K. 2021. Present‐day kinematics in southeastern tibet inferred from GPS measurements. J Geophys Res. 126:e2020JB021305.
- Wen X, Ma S, Xu X, He Y. 2008. Historical pattern and behavior of earthquake ruptures along the eastern boundary of the Sichuan-Yunnan faulted-block, southwestern China. Phys Earth Planet Inter. 168(1-2):16–36. doi:10.1016/j.pepi.2008.04.013.
- Wessel P, Smith WHF, Scharroo R, Luis J, Wobbe F. 2013. Generic mapping tools: improved version released. Eos Trans AGU. 94(45):409–410. doi:10.1002/2013EO450001.
- Xiong W, Chen W, Wang D, Wen Y, Nie Z, Liu G, Dijin W, Yu P, Qiao X, Zhao B, et al. 2022. Coseismic slip and early afterslip of the 2021 Mw 7.4 Maduo, China earthquake constrained by GPS and InSAR data. Tectonophysics. 840:229558. doi:10.1016/j.tecto.2022.229558.
- Xiong X, Shan B, Zheng Y, Wang R. 2010. Stress transfer and its implication for earthquake hazard on the Kunlun Fault, Tibet. Tectonophysics. 482(1-4):216–225. doi:10.1016/j.tecto.2009.07.020.
- Xu D, Xiao J, He J, Wang W. 2020. Strong earthquake clustering around the eastern Tibetan Plateau after the 2008 Mw 7.9 Wenchuan earthquake. Sci China Earth Sci. 63(7):999–1012. doi:10.1007/s11430-019-9581-x.
- Xu J, Shao Z, Ma H. 2013. Evolution of Coulomb stress and stress interaction among strong earthquakes along the Xianshuihe fault zone. Chin J Geophys. 56:1146–1158.
- Xu J, Zeng X. 2022. Tectonic Stress Redistribution Induced by Geothermal Gradient Difference: numerical Modeling of Stress Around the Anninghe Seismic Gap in the Southeastern Tibetan Plateau. Pure Appl Geophys. 179(10):3713–3726. doi:10.1007/s00024-022-03162-1.
- Xu X, Wen X, Zheng R, Ma W, Song F, Yu G. 2003. Pattern of latest tectonic motion and its dynamics for active blocks in Sichuan-Yunnan region, China. Sci China Ser D-Earth Sci. 46(S2):210–226. doi:10.1360/03dz0017.
- Xu X, Wu X, Yu G, Tan X, Li K. 2017. Seismo-geological signatures for identifying M≥ 7.0 earthquake risk areas and their premilimary application in mainland China. Seismol Geol. 39:219–275.
- Yang Z, Dai D, Zhang Y, Zhang X, Liu J. 2022. Rupture process and aftershock mechanisms of the 2022 Luding M6.8 earthquake in Sichuan, China. Earthquake Sci. 35:474–484.
- Yi G, Long F, Liang M, Zhao M, Zhang H, Zhou R, Li Y, Liu H, Wu P, Wang S, et al. 2023. Seismogenic structure of the 5 September 2022 Sichuan Luding Ms6.8 earthquake sequence. Chin J Geophys. 66:1363–1384.
- Yi G, Wen X, Su Y. 2008. Study on the potential strong‐earthquake risk for the eastern boundary of the Sichuan‐Yunnan active faulted‐block, China. Chin J Geophys. 51:1719–1725.
- Zhang G, Li Y, Hu X. 2022. Nucleation mechanism of the 2021 Mw 7.4 Maduo earthquake, NE Tibetan Plateau: insights from seismic tomography and numerical modeling. Tectonophysics. 839:229528. doi:10.1016/j.tecto.2022.229528.
- Zhang L, Zhou Y, Zhang X, Zhu A, Li B, Wang S, Liang S, Jiang C, Wu J, Li Y, et al. 2023a. 2022 Mw 6.6 Luding, China, earthquake: a strong continental event illuminating the moxi seismic gap. Seismol Res Lett. 94(5):2129–2142. doi:10.1785/0220220383.
- Zhang P, Deng Q, Zhang G, Ma J, Gan W, Min W, Mao F, Wang Q. 2003. Active tectonic blocks and strong earthquakes in the continent of China. Sci China Ser D-Earth Sci. 46(S2):13–24. doi:10.1360/03dz0002.
- Zhang Z, Fang L, Xu L. 2023b. Primary source characteristics of the 2022 Sichuan Luding Ms6.8 Earthquake. Chin J Geophys. 3:100201.
- Zhang Z, Yao H, Wang W, Liu, C. 2022. 3-D crustal azimuthal anisotropy reveals multi-stage deformation processes of the Sichuan Basin and its adjacent area, SW China. J Geophys Res. 127:e2021–JB023289.
- Zhu X, He J, Wang X, Xiao J. 2022. Three-dimensional finite element modelling on strain localization around the Mabian earthquake swarm, Sichuan Province. Geophys J Int. 230(3):1852–1863. doi:10.1093/gji/ggac153.