ABSTRACT
Immortalized cell lines can be used for diverse in vitro experiments, providing invaluable data before conducting in vivo studies Callithrix jacchus, the common marmoset, is a non-human primate model utilized for studying various human diseases. However, only a few immortalized marmoset cell lines are currently available. In the present study, we reveal that CRISPR-Cas9-mediated targeting of the p53 gene or CDKN2A locus is an effective means for immortalizing primary marmoset skin fibroblasts. In addition to frameshift mutations that result in premature stop codons, in-frame mutations potentially destroying the DNA-binding motif of p53 are frequently detected in immortalized cells. Like Cdkn2a-deficient mouse cells, CDKN2A-deficient marmoset cells express wild-type p53 proteins normally respond to genotoxic stresses, including adriamycin and etoposide. Taken together, these findings indicate that Cas9- mediated gene targeting of the p53 gene or CDKN2A locus is an effective tool for establishing immortalized marmoset cell lines with defined genetic alterations.
Introduction
The common marmoset, Callithrix jacchus, is an emerging non-human primate model used in neuroscience, immunology, reproductive science, and toxicology studies (Kishi et al. Citation2014). Despite the increasing demand for these animals, their supply is limited, as common marmoset is an endangered species (Malukiewicz et al. Citation2015). The supply of immortalized cell lines is unlimited, and their use for in vitro experiments before animal studies is an essential tool to minimize the number of in vivo studies sacrificing animals (Dumenco et al. Citation1995). However, only a limited number of immortalized marmoset cell lines are currently available (Guo et al. Citation2018; Petkov et al. Citation2018). This necessitates development of immortalized marmoset cell lines from specific tissues to be studied.
Because cell immortalization is a critical process for carcinogenesis, numerous immortalized cell lines have been isolated from various human cancers. Different from mouse embryonic stem (ES) cells (Han et al. Citation2020), normal diploid human cells eventually enter replicative senescence after a finite number of cell divisions (Hayflick Citation1965) and thus, to become immortalized, cells must escape replicative senescence, acquiring the capability of infinite cell division. Replicative senescence is attributable to shortening of telomeres, the ends of chromosomes, with each cell division (Blackburn Citation2000). Because normal somatic cells lack the telomerase activity necessary for telomere elongation, telomeres are gradually shortened by successive cell divisions, and finally, critically short telomeres trigger replicative senescence (Shay et al. Citation2001). Consistently, exogenous telomerase overexpression causes elongation of telomeres in primary diploid cells, which then actively proliferate, accompanied by suppressed expression of senescence biomarkers (Bodnar et al. Citation1998). Similarly, primary marmoset skin fibroblasts can be immortalized using the human telomerase catalytic subunit (hTERT) gene (Petkov et al. Citation2018).
p53 is the most frequently mutated gene in human cancers, and approximately 90% of p53 mutations are missense mutations in the DNA-binding domain (Baugh et al. Citation2018). p53 mutations are common in spontaneously immortalized primary mouse embryonic fibroblasts (MEFs) (Harvey and Levine Citation1991), and overexpression of the mutant p53 gene (del329) immortalizes normal human mammary epithelial cells (MECs) (Gao et al. Citation1996). Similarly, cellular senescence does not occur in p53-deficient primary MEFs (Harvey et al. Citation1993).
The CDKN2A locus, also known as INK4A/ARF locus alternatively encodes two tumor suppressor proteins, p16INK4A and p14ARF (p19Arf in mice), and is one of the most frequently mutated genes in human cancers. Most mutations cause functional loss of both p16INK4A and p14ARF (Sharpless and DePinho Citation1999). In mice, genetic ablation of both p16Ink4a and p19Arf allows primary MEFs to consistently grow with no detectable senescent phase (Serrano et al. Citation1996). p19Arf-deficient MEFs with retention of p16Ink4a gene expression are also immortal (Kamijo et al. Citation1997), but immortalization of p16Ink4a-null MEFs is significantly lower than that of MEFs null for the Cdkn2a locus or p19Arf gene (Sharpless et al. Citation2001), indicating a predominant role for the p19Arf gene in cellular senescence.
In the present study, we determined if deficiencies in the p53 gene or CDKN2A locus could immortalize primary common marmoset skin fibroblasts. To induce null mutations in these genes, a CRISPR-Cas9 system from Streptococcus pyogenes (Cho et al. Citation2013; Cong et al. Citation2013; Mali et al. Citation2013) was adapted and provided a simple and robust means for efficient immortalization of primary skin cells from common marmosets. This platform can potentially be used to establish immortalized cell lines that are essential for in vitro studies prior to in vivo experiments using common marmoset monkeys, which are available in very limited numbers due to their status as an endangered species.
Materials and methods
Animal, biopsy, and primary skin cell culture
A 3-year-old female common marmoset was purchased from laboratory animal supplier, CLEA (Kawasaki, Japan). Animal procedures were approved by the Institutional Animal Care and Use Committee of the Biomedical Research Institute at the Seoul National University Hospital, an accredited research institute of the Association for Assessment and Accreditation of Laboratory Animal Care International (AAALAC; SNUH-IACUC No: 20-0161). An abdominal skin sample was collected during autopsy. Primary skin fibroblasts were isolated as described previously (Seluanov et al. Citation2010). Briefly, 7 days after digestion of skin tissues using LiberaseTM (Roche) in DMEM/F12 (Gibco), cells were transferred to EMEM (ATCC) containing 10% (v/v) FBS (Sigma-Adrich) and 10 unit/ml Penicillin/Streptomycin. Upon confluence, cells were detached using 0.05% Trypsin-EDTA (Invitrogen) and were then cultivated with 10% (v/v) FBS/DMEM media supplemented with Penicillin/Streptomycin.
Cloning of single guide RNAs (sgRNAs) and establishment of clonal cell lines
sgRNAs specific for marmoset p53 gene and CDKN2A locus were designed using Benchling (https://www.benchling.com), and the following oligomers were purchased from Macrogen, Inc.: 5′–CACCGTGTAACAGTTCCTGCATGGG–3′ and 5′–AAACCCCATGCAGGAACTGTTACAC–3′ for p53-specific sgRNA; 5′–CACCGACCGGTTCACGACGCTGCCC–3′ and 5′–AAACGGGCAGCGTCGTGAACCGGTC–3′ for CDKN2A locus-specific sgRNA. For the delivery of CRISPR-Cas9 into the cells, a lentiCRISPRv2 vector, a gift from Feng Zhang (Addgene plasmid #52961; http://n2t.net/addgene:52961; RRID:Addgene_52961), was used. Each pair of oligomers was annealed and cloned into lentiCRISPRv2 using BsmBI restriction enzymes from New England BioLabs, Inc. (NEB). Infectious lentiviral particles were produced as described previously (Sung et al. Citation2013). After lentivirus infection, cells were treated with 2 μg/ml puromycin (Sigma-Aldrich) to remove non-infected cells, and puromycin-resistant cells were used to establish clonal cell lines.
Genotype analysis
To determine the presence of indel mutations in pooled or clonal cells, genomic DNA samples were isolated and T7 endonuclease I (T7E1) assays were conducted as previously described (Oh et al. Citation2021). Briefly, genomic regions encompassing the target site were PCR-amplified, heteroduplex DNAs were generated by heat denaturation and reannealing, and products were purified using a PCR cleanup kit from iNtRON Biotechnology, Inc (Seongnam, Korea). After treatment with 5 units T7E1 (NEB) for 15 min at 37°C, samples were analyzed by agarose gel electrophoresis. The following oligomers were used for PCR amplification: 5′–TTGACCTCCCCATAATTCCA–3′ and 5′–TCGTCCTTTCTGGAGCCTAA–3′ (509 bp) for the TP53 gene; 5′–TTGTCCTTGTCTCACAGGGC–3′ and 5′–CTGATGATTGGCGCGATTGG–3′ (696 bp) for the CDKN2A locus. For Sanger sequencing, PCR products were subcloned using a T-blunt TA cloning kit (SolGent Co., Ltd., Seoul, Korea), and miniprep DNA samples were then prepared using a DNA-spin™ Plasmid DNA Purification Kit (iNtRON Biotechnology, Inc., Seongnam, Korea) and were sequenced at Macrogen (Seoul, Korea).
The following oligomers were used for PCR amplification for the CDKN2B locus: 5′–CCAGACACCGGAGACTTGAA–3′ and 5′–CCCCTTTTGCAGCCTTCATC–3′ (498 bp). For Sanger sequencing, PCR products were purified and sequenced using the following oligomer: 5′–GACTTGAACACCTCTGCACTG–3′.
In vitro growth and senescence-associated β-galactosidase (SAβ-gal) assays
To measure cumulative population doublings, cells were plated at 3 × 105 cells/60 mm dish or 1 × 106 cells/100 mm dish. Confluent cultures were trypsinized, viable cells were counted, and cells were replated at the same density. For viable cell counting, aliquots of trypsinized cells were stained with Trypan Blue staining solution (Invitrogen) and were counted using a Countess II cell counter (Invitrogen) equipped with a CountessTM cell counting chamber slide (Invitrogen). If tissue cultures were not confluent, cells were grown until confluence after adding fresh media. To count senescent cells, SAβ-gal assays were conducted using a Senescence β-Galactosidase Staining Kit (Cell Signaling) according to the manufacturer’s instructions (Kim et al. Citation2020). Photographs were taken using a Nikon eclipse Ts2 microscope (Nikon, Tokyo, Japan) equipped with an HK3.1 CMOS digital camera and HKBASIC Software (KOPTIC).
Semi-quantitative reverse transcription (RT)-polymerase chain reaction (RT-PCR)
Total RNAs were isolated using TRIzol Reagent (Thermo Fisher Scientific, Waltham, MA) according to the manufacturer’s instructions, and the SuperScript III First-strand Synthesis System (Invitrogen, Waltham, MA) was used for reverse transcription (RT). Semi-quantitative RT-PCR reactions were conducted using RT products as template and the following primers: 5′–GATCTGCCTTCCCAAAGGGC–3′ and 5′–AGTGAGGCATTAGGGTGCAA–3′ for p21WAF1 gene (125 bp, XM_035294655); 5′–ACCCTGTGCAAGACCTACAG–3′ and 5′–TGGCGTTTTCTTTGCCGTTC–3′ for MDM2 gene (142 bp, XM_009004180); 5′–GCTGCTCACCTCTGGTGCCA–3′ and 5′–TTCAATCGGGGACGTCTGAG–3′ for p14ARF gene (583 bp, XM_035306720); and 5′–TCGGAGTCAACGGATTTGGTC–3′ and 5′–TTCCCGTTCTCAGCCTTGAC–3′ for GAPDH gene (181 bp, XM_035255536); 5′– CAGAGCTAAAGGAGCTGCTGACC–3′ and 5′–CCTGGAAGTCCACCTCGTTGTC–3′ for S100A4 gene (126 bp, XM_035279100); 5′–CTGGATTCACTCCCTCTGGTTG –3′ and 5′–CATGATGCTGAGAAGTTTCGTTG –3′ for VIM gene (106 bp, XM_002750069); 5′–AGAAAGTGGATGCCGCCTTTAA–3′ and 5′–CATTCCAGGCATCCGCGATGAG –3′ for MMP2 gene (138 bp, XM_017966916); 5-GCCACTCCTACAACCAATATTCTC–3′ and 5′–GCTTGTTCCTCTGGGTTGGAAAG–3′ for FN1 gene (147 bp, XM_008999454).
Western blot analysis
Cells were treated with adriamycin (Sigma-Aldrich) or etoposide (Sigma-Aldrich), and SDS protein samples were then prepared by directly lysing the cells in SDS sample buffer (Elpisbiotech. Inc., Daejeon, Korea) after washing with ice-cold phosphate-buffered saline (PBS).
Western blots were conducted using antibodies against MDM2 (Santa Cruz Biotechnology, Inc., #sc-965), p21WAF1 (Santa Cruz Biotechnology, Inc., #sc-6246), p53 (Santa Cruz Biotechnology, Inc., #sc-126), p16INK4A (Santa Cruz Biotechnology, Inc., #sc-1661), p14ARF (Cell Signaling #74560), and GAPDH (Cell Signaling #2118).
Results
Targeted inactivation of the p53 gene in primary marmoset skin fibroblasts using CRISPR-Cas9
Because most mutations of the p53 DNA-binding domain cause reduction or complete loss of p53 transcriptional activity (Baugh et al. Citation2018), we designed a sgRNA specific for exon 7, which encodes critical amino acid residues of the p53 protein DNA-binding domain ((A)). We could not find any potential off-target sites with less than three mismatches from the sgRNA on the marmoset genome. When this sgRNA was expressed with Cas9 using a lentiviral vector, indel mutations were robustly induced in primary marmoset skin fibroblasts ((B)). As polyclonal marmoset skin fibroblasts actively proliferated and contained diverse indel mutations (Figure S1), we established multiple clonal cell lines with in-frame and/or frameshift mutations ((C)).
Figure 1. Cas9-mediated p53 gene targeting in primary skin fibroblasts isolated from a common marmoset monkey. (A) Schematic representation of the p53-specific sgRNA on exon 7. Target and PAM sequences are denoted by red and blue colors, respectively. Dotted boxes indicate the amino acids critical for the DNA-binding function of p53 protein as a transcription factor. (B) Endonuclease activity induced by p53-specific sgRNA (sgp53). After infection with lentivirus expressing both Cas9 and p53-specific sgRNA, Cas9-induced indel mutations was examined using genomic DNA samples from parental primary skin fibroblasts (−) and puromycin-selected cells (+) by T7E1 assay. (C) Mutated p53 sequences observed in the mutant cell clones. − denotes deleted nucleotides; sequences in lower case denote nucleotide insertions. (D) Western blot analysis of p53 proteins in primary skin fibroblasts (parental; Pa) and p53-/- skin fibroblasts (clone #4 in c).
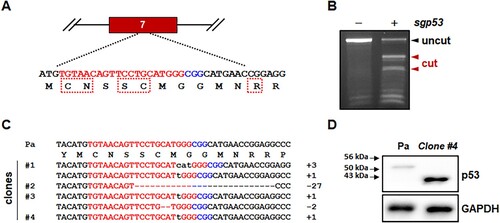
Among the p53-mutated clones, we selected mutant clone #4, which was homozygous for a 1 bp insertion in the target sequence ((C)). Because of the frameshift mutation, clone #4 expressed a mutant p53 protein comprised of ∼1–244 amino acid residues of wild-type marmoset p53 protein (395 amino acid residues, XM_002747948) and 20 aberrant amino acid residues. Accordingly, while Western blot analysis detected a wild-type p53-specific signal in parental primary skin fibroblasts, p53 mutant cells strongly expressed a smaller mutant p53 protein that is approximately 43 kDa, but not wild-type p53 protein ((D)).
Targeted mutations of the CDKN2A locus in primary marmoset skin fibroblasts using CRISPR-Cas9
The CDKN2A locus encodes two proteins, p16INK4A and p14ARF, with exon 1 sequences, 1α and 1β, respectively, and alternative reading frames of the common exons in humans and mice (Quelle et al. Citation1995). The CDKN2A locus in the marmoset genome also encodes the p16INK4A gene ((A)). Although marmoset p14ARF protein and transcript have not yet been annotated, the marmoset genome harbors a putative exon 1β that is upstream of exon 1α ((A)). Accordingly, the predicted transcript variant X2 (XM_035306720), which harbors putative exon 1β, alternatively encodes the putative marmoset p14ARF protein (Figure S2A), which is highly homologous to human p14ARF protein (Figure S2B).
Figure 2. Generation of mutant marmoset skin fibroblasts deficient in both p16INK4A and p14ARF genes using CRISPR-Cas9. (A) Schematic representation of CDKN2A-specific sgRNA on exon 2, which is common to both p16INK4A and p14ARF. Target and PAM sequences are denoted by red and blue colors, respectively. (B) Endonuclease activity of Cas9 induced by common exon 2-specific sgRNA (sgCDKN2A). T7E1 assays were conducted using genomic DNA samples from parental primary skin fibroblasts (−) and puromycin-selected cells (+) after infection with lentivirus expressing both Cas9 and sgCDKN2A. (C) Mutated CDKN2A sequences observed in the clones. A polymorphic nucleotide is shaded in gray. Ref, reference genomic DNA sequence of the CDKN2A locus; Pa, parental marmoset skin fibroblasts; − denotes deleted nucleotides; sequences in lower case denote nucleotide insertions. (D) Western blot analysis of p16INK4A and p14ARF proteins in p53-/- cells ( clone #4) and CDKN2A-/- cells (clone #1 in c). Short exp., short exposure time; long exp., long exposure time.
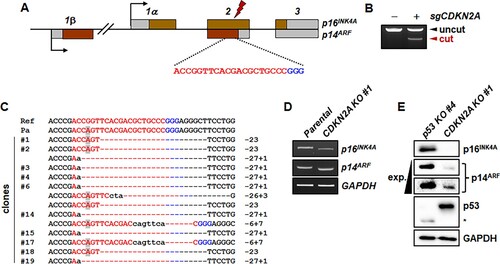
To inactivate both the p16INK4A and p14ARF genes simultaneously, we selected an sgRNA specifically targeting the common exon 2 ((A)) and confirmed that the sgRNA actively induced indel mutations in primary marmoset skin fibroblasts ((B)). The sgRNA has an off-target site with a 1-bp mismatch on the highly homologous CDKN2B gene. Except for this off-target site, there is no potential off-target sites with less than three mismatches from our CDKN2A gene-specific sgRNA on the marmoset genome (Tsai et al. Citation2015; Kim et al. Citation2016). The CDKN2A-targeted marmoset skin fibroblasts were used to establish clonal cells deficient for both p16INK4A and p14ARF. We detected a sequence variation, XM_035306720 c.286G > A (p.Gly96Ser), which was prevalent in our wild-type marmosets (Figure S3). However, the 1 bp mismatch between the target sequence and the sgRNA did not compromise the action of CRISPR-Cas9, and thus most of the clones had both insertions and deletions resulting in simultaneous frameshifts in the p16INK4A and p14ARF genes ((C)). For example, the 23 bp-deleted clone #1 encoded mutant p16INK4A protein was composed of 76 amino acid residues of wild-type p16INK4A protein at the N-terminus and 28 amino acid residues of p14ARF protein at the C-terminus (Figure S4A), reflecting the alternative reading frame in the CDKN2A locus. For the p14ARF gene, the frameshift mutation of clone #1 involved the third reading frame that was not used by either the p16INK4A or p14ARF genes, and thus the open reading frame encoded a mutant p14ARF protein containing 97 amino acid residues of wild-type p14ARF protein at the N-terminus and 103 mutated amino acid residues at the C-terminus (Figure S4B).
We confirmed expression of p16INK4A and p14ARF in CDKN2A-mutant clone #1. When compared with those of parental cells, transcription of the p16INK4A gene was slightly decreased, while transcription of the p14ARF gene was unchanged ((D)). Due to the 23 bp deletions in CDKN2A-mutant clone #1, the sizes of the p16INK4A and p14ARF cDNAs were slightly smaller than those of the parental cells ((D)). We also measured expression of p16INK4A and p14ARF proteins in the CDKN2A-mutant cells using p53-mutant clone #4 as a positive control ((E)). p16INK4A protein was robustly expressed in p53-mutant cells, but not in CDKN2A-mutant cells ((E)). Although a weak non-specific signal was present, p53-mutant cells robustly expressed p14ARF protein, but CDKN2A-mutant cells did not ((E)).
We also monitored the off-target mutation of the CDKN2B gene (Figure S5). The sequencing result showed the co-existence of wild-type and 9-bp deleted mutant alleles (Figure S5).
Characterization of p53- and CDKN2A-mutant marmoset cells
We examined whether p53- and CDKN2A-mutant marmoset cells continued to divide without developing senescence. Cumulative population doubling levels (PDLs) of mutant marmoset cells were measured and compared with that of primary marmoset cells ((A,B)). The proliferation of primary cells gradually slowed and eventually ceased ((A,B)). For p53-mutant marmoset cells, both polyclonal and monoclonal cells continuously proliferated without decreased growth rates and had similar growth characteristics ((A)). p53-mutant cells (clone #4) were thawed at passage 8 and cell growth was measured from passage 10 to passage 33, resulting in 17 cumulative population doublings (PDs). For CDKN2A-mutant cells, the growth rate of polyclonal cells did not decrease ((B)). Compared with polyclonal cells, CDKN2A-mutant clone #1 exhibited an increased growth rate ((B)) with 63 cumulative PD when cell growth was measured from passage 18 to passage 35. CDKN2A-mutant cells retained higher proliferation capacities than those of p53-mutant cells ((A,B)). Most parental cells were enlarged and flattened by passage 18 compared with passage 8, a characteristic feature of senescent cells. In addition, p53 and CDKN2A-mutant cells did not lose their fibroblast characters ((C) and Figure S6). Notably, senescence-associated β-galactosidase (SA-βgal)-positive cells were frequently present among primary marmoset cells at passage 18, but not in p53 (passage 23)- and CDKN2A (passage 24)- mutant clones ((C)). Taken together, these data support establishment of genetically defined immortalized marmoset cell lines.
Figure 3. Immortalized phenotypes observed in both p53- and CDKN2A-deficient marmoset skin fibroblasts. (A, B) Cumulative population doublings (PDs) of p53-deficient (A) and CDKN2A-deficient (B) marmoset skin fibroblasts. Growth of polyclonal cells (purple triangles) and monoclonal mutant cells (red rectangles; p53-/- clone #4 and CDKN2A-/- clone #1) was compared with that of parental primary skin fibroblasts (blue circle). (C) Senescence-associated β-galactosidase (SAβ-gal) assays conducted with marmoset skin fibroblasts and immortalized p53- and CDKN2A-deficient clones at the denoted passages. Scale bar, 200 μm.
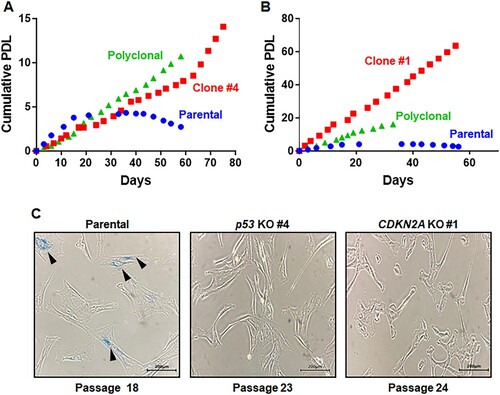
Genotoxic stresses activate p53 and would thus be expected to increase expression of p53 target genes in the CDKN2A-mutant cell line with intact p53. We treated CDKN2A- and p53-mutant cell lines with adriamycin and etoposide and measured expression of p53 and its target genes (). Adriamycin stabilized p53 protein and increased MDM2 and p21WAF1 protein levels in the CDKN2A-mutant marmoset cell line, which did not occur in the p53-mutant marmoset cell line ((A)). Transcript levels of p53 target genes MDM2 and p21WAF1 were concomitantly increased in the CDKN2A-mutant cell line, but not in the p53-mutant cell line ((B)). Consistently, etoposide treatment also increased p53 protein levels and target gene expressions ((C,D)).
Figure 4. Induction of p53 target genes by genotoxic stresses in immortalized CDKN2A-deficient marmoset skin fibroblasts. (A) Western blot analysis of p53 and its target gene products, MDM2 and p21WAF1, in immortalized p53- and CDKN2A-deficient marmoset skin fibroblasts treated with 200 ng/ml adriamycin for 4 h. (B) Assessment of adriamycin-induced p53 target gene (MDM2 and p21WAF1) transcription by semi-quantitative RT-PCR. Immortalized marmoset cells were treated as in a. The asterisk indicates truncated marmoset p53 proteins. (C) Western blot analysis of p53 and its target gene products, MDM2 and p21WAF1, in immortalized p53- and CDKN2A-deficient marmoset skin fibroblasts treated with 0.5 μM etoposide for 4 h. The asterisk indicates truncated marmoset p53 proteins. (D) Etoposide induction of p53 target gene (MDM2 and p21WAF1) transcription in immortalized marmoset cells. Semi-quantitative RT-PCR reactions were conducted with cells treated as in c.
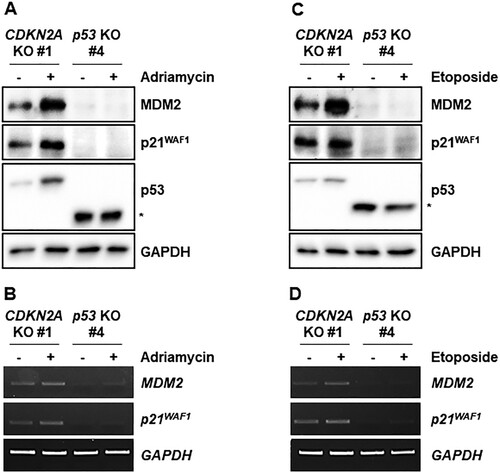
Cumulatively, these findings demonstrated that CRISPR-Cas9-mediated knockout of the p53 gene and CDKN2A locus is a simple means to establish immortalized cell lines from marmoset skin biopsies. In turn, the resulting combination of p53-proficient (CDKN2A-mutant) and p53-deficient cells is an excellent in vitro model for the study of genotoxic stresses.
Discussion
Immortalized human cell lines are essential for in vitro experiments and have contributed enormously to our understanding of the cellular and biochemical mechanisms underlying biological phenomena and pathological conditions. Common marmosets are a versatile non-human primate model suitable for studying a broad range of human diseases and generating gene-edited animal models. Because extensive in vitro experiments should be conducted prior to in vivo experiments using live marmosets due to limited animal availability and ethical considerations, immortalized cell lines derived from marmoset tissues of interest will greatly enhance the efficiency of these in vitro studies.
Recently, several reports have described successful generation of immortalized marmoset cell lines, and our study will provide an alternative and complementary method (Guo et al. Citation2018; Petkov et al. Citation2018; Orimoto et al. Citation2022). As in normal human cells that undergo a finite number of cell divisions (Bodnar et al. Citation1998), piggyBac transposon-mediated expression of the hTERT gene successfully immortalizes marmoset skin fibroblasts (Petkov et al. Citation2018). By contrast, hTERT expression alone is not sufficient for immortalization of marmoset muscle fibroblasts; a mutant form of cyclin-dependent kinase 4 (CDK4R24C) and overexpression of wild-type Cyclin D1 are also required (Orimoto et al. Citation2022). CDK4R24C mutation predisposes humans to hereditary melanoma and abolishes the ability of CDK4 protein to bind p16INK4A protein (Wolfel et al. Citation1995; Zuo et al. Citation1996). Therefore, it can be reasonably expected that the p16INK4A-mediated senescence signal was perturbed by expression of CDK4R24C in the immortalized marmoset muscle fibroblasts. Based on our results, CDKN2A gene knockout could exert a comparable effect to introducing the CDK4R24C transgene.
As previously reported in human-induced hepatocytes (hiHeps) (Huang et al. Citation2014), the simian virus 40 (SV40) large T-antigen also immortalizes marmoset hepatic progenitor cells (Guo et al. Citation2018). SV40 large T-antigen is effective for cellular immortalization but inactivates the p53 gene (Ahuja et al. Citation2005). This suggests that SV40 large T-antigen-immortalized cells would not be suitable for study of p53-dependent cellular responses. Therefore, marmoset cells immortalized by CDKN2A gene targeting are preferable, as these cells retain functional p53. In support of this, we demonstrated that genotoxic stress-induced gene expression could be analyzed using CDKN2A- and p53-deficient immortalized cells as a pair.
The present study contributes to the development of novel marmoset cell lines from tissue types of interest. Our strategy can be improved by avoiding the off-target mutation detected in the highly homologous CDKN2B gene (Figure S5). It will be accomplished by targeting the exon 1β to generate p14ARF-deficient but p16INK4A-proficient cell lines as p19Arf deficiency alone makes primary MEFs immortal (Kamijo et al. Citation1997). In addition, vector-free or adeno-associated virus (AAV) vector systems are suitable for minimizing off-target effects induced by the constitutive expression of the CRISPR-Cas9 system. These cell lines will expedite biomedical studies using common marmoset monkeys and minimize the scale of animal experiments by allowing conduct of extensive in vitro experiments prior to initiating in vivo studies.
Supplemental Material
Download PDF (1.9 MB)Disclosure statement
The authors have submitted a patent application based on the results reported in this paper.
Additional information
Funding
References
- Ahuja D, Saenz-Robles MT, Pipas JM. 2005. SV40 large T antigen targets multiple cellular pathways to elicit cellular transformation. Oncogene. 24(52):7729–7745.
- Baugh EH, Ke H, Levine AJ, Bonneau RA, Chan CS. 2018. Why are there hotspot mutations in the TP53 gene in human cancers? Cell Death Differ. 25(1):154–160.
- Blackburn EH. 2000. The end of the (DNA) line. Nat Struct Biol. 7(10):847–850.
- Bodnar AG, Ouellette M, Frolkis M, Holt SE, Chiu CP, Morin GB, Harley CB, Shay JW, Lichtsteiner S, Wright WE. 1998. Extension of life-span by introduction of telomerase into normal human cells. Science. 279(5349):349–352.
- Cho SW, Kim S, Kim JM, Kim JS. 2013. Targeted genome engineering in human cells with the Cas9 RNA-guided endonuclease. Nat Biotechnol. 31(3):230–232.
- Cong L, Ran FA, Cox D, Lin S, Barretto R, Habib N, Hsu PD, Wu X, Jiang W, Marraffini LA, et al. 2013. Multiplex genome engineering using CRISPR/Cas systems. Science. 339(6121):819–823.
- Dumenco L, Oguey D, Wu J, Messier N, Fausto N. 1995. Introduction of a murine P53 mutation corresponding to human codon-249 into a murine hepatocyte cell-line results in growth advantage, but not in transformation. Hepatology. 22(4):1279–1288. English.
- Gao Q, Hauser SH, Liu XL, Wazer DE, Madoc-Jones H, Band V. 1996. Mutant p53-induced immortalization of primary human mammary epithelial cells. Cancer Res. 56(13):3129–3133.
- Guo Z, Jing R, Rao Q, Zhang L, Gao Y, Liu F, Wang X, Hui L, Yin H. 2018. Immortalized common marmoset (Callithrix jacchus) hepatic progenitor cells possess bipotentiality in vitro and in vivo. Cell Discov. 4:23.
- Han NR, Baek S, Kim HY, Lee KY, Yun JI, Choi JH, Lee E, Park CK, Lee ST. 2020. Generation of embryonic stem cells derived from the inner cell mass of blastocysts of outbred ICR mice. Anim Cells Syst. 24(2):91–98.
- Harvey DM, Levine AJ. 1991. P53 alteration is a common event in the spontaneous immortalization of primary BALB/c murine embryo fibroblasts. Genes Dev. 5(12B):2375–2385.
- Harvey M, Sands AT, Weiss RS, Hegi ME, Wiseman RW, Pantazis P, Giovanella BC, Tainsky MA, Bradley A, Donehower LA. 1993. In vitro growth characteristics of embryo fibroblasts isolated from p53-deficient mice. Oncogene. 8(9):2457–2467.
- Hayflick L. 1965. The limited in vitro lifetime of human diploid cell strains. Exp Cell Res. 37:614–636.
- Huang P, Zhang L, Gao Y, He Z, Yao D, Wu Z, Cen J, Chen X, Liu C, Hu Y, et al. 2014. Direct reprogramming of human fibroblasts to functional and expandable hepatocytes. Cell Stem Cell. 14(3):370–384.
- Kamijo T, Zindy F, Roussel MF, Quelle DE, Downing JR, Ashmun RA, Grosveld G, Sherr CJ. 1997. Tumor suppression at the mouse INK4a locus mediated by the alternative reading frame product p19ARF. Cell. 91(5):649–659.
- Kim D, Kim S, Kim S, Park J, Kim JS. 2016. Genome-wide target specificities of CRISPR-Cas9 nucleases revealed by multiplex digenome-seq. Genome Res. 26(3):406–15.
- Kim DY, Moon SH, Han JH, Kim MJ, Oh SJ, Bharti D, Lee SH, Park JK, Rho GJ, Jeon BG. 2020. Terminal differentiation into adipocyte and growth inhibition by PPARgamma activation in human A549 lung adenocarcinoma cells. Anim Cells Syst. 24(6):329–340.
- Kishi N, Sato K, Sasaki E, Okano H. 2014. Common marmoset as a new model animal for neuroscience research and genome editing technology. Dev Growth Differ. 56(1):53–62.
- Mali P, Yang L, Esvelt KM, Aach J, Guell M, DiCarlo JE, Norville JE, Church GM. 2013. RNA-guided human genome engineering via Cas9. Science. 339(6121):823–826.
- Malukiewicz J, Boere V, Fuzessy LF, Grativol AD, Silva IDE, Pereira LCM, Ruiz-Miranda CR, Valenca YM, Stone AC. 2015. Natural and anthropogenic hybridization in two species of eastern Brazilian marmosets (Callithrix jacchus and C. penicillata). PLoS One. 10(6):e0127268. English.
- Oh SH, Lee HJ, Ahn MK, Jeon MY, Yoon JS, Jung YJ, Kim GN, Baek IJ, Kim I, Kim KM, et al. 2021. Multiplex gene targeting in the mouse embryo using a Cas9-Cpf1 hybrid guide RNA. Biochem Biophys Res Commun. 539:48–55.
- Orimoto A, Shinohara H, Eitsuka T, Nakagawa K, Sasaki E, Kiyono T, Fukuda T. 2022. Immortalization of common marmoset-derived fibroblasts via expression of cell cycle regulators using the piggyBac transposon. Tissue Cell. 77:101848.
- Petkov S, Kahland T, Shomroni O, Lingner T, Salinas G, Fuchs S, Debowski K, Behr R. 2018. Immortalization of common marmoset monkey fibroblasts by piggyBac transposition of hTERT. PLoS One. 13(9):e0204580.
- Quelle DE, Zindy F, Ashmun RA, Sherr CJ. 1995. Alternative reading frames of the INK4a tumor suppressor gene encode two unrelated proteins capable of inducing cell cycle arrest. Cell. 83(6):993–1000.
- Seluanov A, Vaidya A, Gorbunova V. 2010. Establishing primary adult fibroblast cultures from rodents. J Vis Exp. 44:e2033.
- Serrano M, Lee H, Chin L, Cordon-Cardo C, Beach D, DePinho RA. 1996. Role of the INK4a locus in tumor suppression and cell mortality. Cell. 85(1):27–37.
- Sharpless NE, Bardeesy N, Lee KH, Carrasco D, Castrillon DH, Aguirre AJ, Wu EA, Horner JW, DePinho RA. 2001. Loss of p16Ink4a with retention of p19Arf predisposes mice to tumorigenesis. Nature. 413(6851):86–91.
- Sharpless NE, DePinho RA. 1999. The INK4A/ARF locus and its two gene products. Curr Opin Genet Dev. 9(1):22–30.
- Shay JW, Zou Y, Hiyama E, Wright WE. 2001. Telomerase and cancer. Hum Mol Genet. 10(7):677–685.
- Sung YH, Jin Y, Kang Y, Devkota S, Lee J, Roh JI, Lee HW. 2013. Ei24, a novel E2F target gene, affects p53-independent cell death upon ultraviolet C irradiation. J Biol Chem. 288(43):31261–31267.
- Tsai SQ, Zheng Z, Nguyen NT, Liebers M, Topkar VV, Thapar V, Wyvekens N, Khayter C, Iafrate AJ, Le LP, et al. 2015. GUIDE-seq enables genome-wide profiling of off-target cleavage by CRISPR-Cas nucleases. Nat Biotechnol. 33(2):187–197.
- Wolfel T, Hauer M, Schneider J, Serrano M, Wolfel C, Klehmann-Hieb E, De Plaen E, Hankeln T, Meyer zum Buschenfelde KH, Beach D. 1995. A p16INK4a-insensitive CDK4 mutant targeted by cytolytic T lymphocytes in a human melanoma. Science. 269(5228):1281–1284.
- Zuo L, Weger J, Yang Q, Goldstein AM, Tucker MA, Walker GJ, Hayward N, Dracopoli NC. 1996. Germline mutations in the p16INK4a binding domain of CDK4 in familial melanoma. Nat Genet. 12(1):97–99.