ABSTRACT
Circadian rhythm regulates physiological cycles of awareness and sleepiness. Melatonin production is primarily regulated by circadian regulation of gene expression and is involved in sleep homeostasis. If the circadian rhythm is abnormal, sleep disorders, such as insomnia and several other diseases, can occur. The term ‘autism spectrum disorder (ASD)’ is used to characterize people who exhibit a certain set of repetitive behaviors, severely constrained interests, social deficits, and/or sensory behaviors that start very early in life. Because many patients with ASD suffer from sleep disorders, sleep disorders and melatonin dysregulation are attracting attention for their potential roles in ASD. ASD is caused by abnormalities during the neurodevelopmental processes owing to various genetic or environmental factors. Recently, the role of microRNAs (miRNAs) in circadian rhythm and ASD have gained attraction. We hypothesized that the relationship between circadian rhythm and ASD could be explained by miRNAs that can regulate or be regulated by either or both. In this study, we introduced a possible molecular link between circadian rhythm and ASD. We performed a thorough literature review to understand their complexity.
Relationship between circadian rhythms and ASD
Circadian rhythm
The day-night cycle affects almost all living creatures on Earth, and their physiological functions follow a roughly 24-hour cyclic pattern. The term ‘circadian rhythm’ refers to this physiological, 24-hour cycle that exhibits an endogenous and entrainable oscillation (Dibner et al. Citation2010; Patke et al. Citation2020). In vivo, circadian rhythms are mainly regulated by environmental signals such as light, food, and arousal stimuli (Lee and Kim Citation2021). Circadian rhythm is generated by the molecular clock system. Notably, the mammalian circadian clock is conceptualized as a hierarchical system in which the brain clock located in the suprachiasmatic nucleus acts as a master regulator that synchronizes or tunes other peripheral clocks distributed throughout the body (Takahashi Citation2017). Circadian rhythm plays an important role in the central nervous system and has a great impact on the physiology of organisms. It is important to maintain the appropriate circadian rhythm to maintain homeostasis; dysregulated circadian rhythms can increase the potential of developing dangerous diseases such as cancer and can have a notable impact on the development of brain diseases such as degenerative neurological diseases (Sulli et al. Citation2019; Shin Citation2020). Variations in light are captured by the optic nerves that feed the signals in the brain, thereby, forming the circadian rhythm that is essential for day-to-day life (Sulli et al. Citation2018).
Sleep homeostasis is linked to the 24-hour cycle and is controlled by melatonin. Melatonin is a powerful antioxidant molecule involved in the regulation of the 24-hour cycle, seasonal rhythms, and immune functions (Brzezinski Citation1997). Patients with Magenis syndrome often show symptoms of autism spectrum disorder (ASD). And patients with Megenis syndrome have sleep disturbance as one of their major problems (Trickett et al. Citation2018). And in patients with ASD, abnormal concentrations of melatonin have been observed and are thought to impact human behavior (Trickett et al. Citation2018; Wu et al. Citation2020b; Martinez-Cayuelas et al. Citation2022).
Autism spectrum disorder
Autism spectrum disorder is a prevalent, highly heritable, and heterogeneous neurodevelopmental disorder with underlying cognitive characteristics and frequently co-occurs with other illnesses. Manifestations of autism include difficulties with social interaction and communication, abnormal sensory experiences, repetitive behaviors, and varied degrees of intellectual disability. Along with these core symptoms, co-occurring psychiatric or neurological disorders are frequent in individuals with autism, with attention-deficit/hyperactivity disorder (ADHD), anxiety, depression, and epilepsy being the most common (Kas et al. Citation2014; Herrero et al. Citation2020; Lord et al. Citation2020; Park and Jung Citation2022).
Over the last decade, considerable research has been conducted to identify the causes of ASD, and great progress has been made in understanding the genetics of ASD. The ASD probability that may occur as a genetic factor is estimated to be 17% to 52% of the total incidence of ASD (Iakoucheva et al. Citation2019; Zhou et al. Citation2019). New mutations have been identified, including copy number modifications and point mutations that are likely to disrupt protein-coding genes and lead to ASD (Rylaarsdam and Guemez-Gamboa Citation2019; Dell'Osso et al. Citation2022). Several studies have focused on the role of the 24-hour periodic rhythm in ASD since aberrant melatonin levels, patterns, and sleep problems have been associated with the ASD (Melke et al. Citation2008; Doyen et al. Citation2011; Rossignol and Frye Citation2011; Wu et al. Citation2020b; Martinez-Cayuelas et al. Citation2022).
Relationship between circadian rhythm and ASD
There have been several studies that have reported disrupted circadian rhythms in individuals with ASD. These disruptions can manifest in a variety of ways, such as difficulty falling asleep, difficulty staying asleep, and an overall change in the timing of sleep. Additionally, some research has found that individuals with ASD may have a reduced sensitivity to light, which can further disrupt their circadian rhythms. This is associated with the autonomic nervous system and circadian rhythms. Sleep disorders are correlated with the severity of ASD symptoms. SHANK3, a candidate gene for predicting ASD risk, has been shown to regulate the expression of circadian and sleep transcription factors such as PER3, Bhlhe41, Hlf, Tef, and Nr1d1 in mice, providing molecular basis for sleep problems in patients with ASD (Ingiosi et al. Citation2019). In addition to genetic factors, the circadian rhythm-controlled hormones, such as melatonin and cortisol, affect ASD. Melatonin disruptions, especially in patients with ASD, appears to represent changes associated with sleep disorders, gastrointestinal motor changes, behavioral and emotional defects, and sensory protein dysfunctions (Dell'Osso et al. Citation2022) ().
Figure 1. The mechanism of circadian rhythm and ASD. Explaining the molecular mechanism of the circadian clock system and the relationship between circadian rhythms and ASD. The core clock genes, BMAL1 and CLOCK, form the positive arm of transcriptional/translational feedback loops by heterodimerizing and binding to the E-box element on circadian target genes to activate transcription, including period (PER) (homologs: 1-3), cryptochrome (CRY) (homologs: 1 and 2), retinoid-related orphan receptor (ROR), Rev-Erb, and other genes in output pathways. The negative feedback loop is created by the complex formed by PER and CRY, which blocks BMAL1/CLOCK-driven transcription. The expression of the BMAL1 gene is modulated by ROR and Rev-Erb, respectively, which also affects the activity of the loops. Other circadian-controlled genes, such as AANAT, the primary enzyme for melatonin production, are also rhythmically expressed in response to the circadian clock genes. Controlled circadian cycles consequently influence the rhythmicity and expression level of melatonin, which also influences the quantity and quality of sleep. Dysregulation of melatonin will cause sleep problems and aberrant miRNA levels as well as gene expression patterns. Genes linked to ASD may have altered expression levels due to abnormal miRNA expression. Alterations in brain metabolism may also be brought on by ASD-mediated altered gene expression, including changes in miRNA levels. Created with BioRender.com.
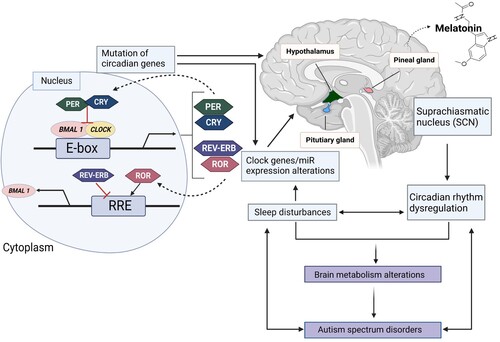
Sleep disorders are more common in children with ASD than in those with developing and intellectual disabilities (Yenen and Çak Citation2020). Most studies agree that sleep problems are associated with behavioral disorders in children with ASD (Malow et al. Citation2006). While less than 30% of the actual child population have sleep problems, approximately 50–80% of children with ASD are estimated to have sleep problems. Recent studies have shown abnormal melatonin secretion in children with ASD, suggesting that a disrupted internal clock and a dysregulated 24-hour cycle may be associated with ASD. It is found that children with ASD had significantly lower melatonin levels with also altered melatonin synthesis compared to typically developing children, which may contribute to sleep difficulties (Melke et al. Citation2008; Doyen et al. Citation2011; Tordjman et al. Citation2013; Gringras et al. Citation2017; Wu et al. Citation2020b). And children with ASD had a delay in the timing of their melatonin onset, which may contribute to difficulty falling asleep (Rossignol and Frye Citation2011; Martinez-Cayuelas et al. Citation2022). Indeed, studies using different methods and independent autism samples indicate that abnormally low melatonin levels are a frequent characteristic of ASD (Ritvo et al. Citation1993; Tordjman et al. Citation2005; Melke et al. Citation2008; Tordjman et al. Citation2012). Melatonin, which affects these circadian rhythms, is the backbone of sleep regulation. It also plays an important role in neurodevelopment. During pregnancy, the establishment of regular sleep patterns and circadian rhythms of the fetus, which are crucial for appropriate neurodevelopment, is dependent on the melatonin hormone (Voiculescu et al. Citation2014). The melatonin crosses the placenta into the fetal circulation and transmits photoperiodic information to the fetus. A fetus’s neurodevelopment is disrupted by an aberrant sleep pattern, such as disturbed REM sleep brought on by an irregular melatonin level, rhythm, or sensitivity (Morrissey et al. Citation2004; Tamura et al. Citation2008; Voiculescu et al. Citation2014; Jin et al. Citation2018). Therefore, abnormal melatonin secretion is associated with neurodevelopmental abnormalities, including circadian disorders and ASD (Jin et al. Citation2018). And it was also found that children with ASD had lower cortisol levels in the morning and higher at night compared to typically developing children, which may indicate a disruption in the HPA axis and the circadian rhythm with sleep difficulties (Gunnar and Vazquez Citation2001; Corbett et al. Citation2008; Corbett et al. Citation2009; Hughes Citation2009).
Some genetic factors may predispose some individuals more with ASD than others. In one study, the researchers screened single nucleotide polymorphisms in eleven clock/circadian-controlled genes in 110 individuals with ASD and their parents. Significant allele associations were detected for clock genes Per1 and Npas2 (Nicholas et al. Citation2007). It was found that a specific clock gene called BMAL1 mutant mice showed autistic-like behavior with disruptions in the circadian rhythm (Liu et al. Citation2022a). Indeed, missense mutation of BMAL1 was found in ASD and haploinsufficiency of BMAL1 caused altered circadian rhythm and autism-like behavior in mice (Yang et al. Citation2016; Singla et al. Citation2022). Additionally, clock genes period1 (PER1) and cryptochrome1 (CRY1) were disrupted in a mouse model of Fragile X Syndrome (FXS), which is one of the most known genetic causes of autism (Lewis et al. Citation2007; Zhang et al. Citation2008; Spencer et al. Citation2011; Dolan et al. Citation2013; Sare et al. Citation2017).
In recent studies, microRNAs (miRNAs) have attracted attention as important biomarkers for identifying ASD (Hu et al. Citation2017; Ortega et al. Citation2021). miRNAs function as gene expression regulators. They are a common class of endogenous short, non-coding RNAs that primarily regulate the expression of protein-coding genes at the mRNA level via translational repression and/or degradation of their target mRNAs (Iwakawa and Tomari Citation2015; O'Brien et al. Citation2018). The Argonaute (AGO) proteins attach to the short (20–22 nucleotide [nt]) miRNAs, which then direct the AGO-associated RNA-induced silencing complex (RISC) to specific mRNA targets (O'Brien et al. Citation2018; Muller et al. Citation2019). It was observed that >60% of protein-coding genes are under selective pressure to retain pairing with miRNAs (Shu et al. Citation2017). AGO2 then facilitates the miRNA-dependent interaction of the RISC complex with mRNAs. This suggests that the majority of protein-coding genes are very susceptible to regulation by miRNAs. Recent evidence suggests that the genes regulating the circadian rhythm are regulated by miRNA that consists of 20–22 short, small non-coding nucleotides (Kim et al. Citation2009). The functions of miRNAs are diverse and include mRNA stability, control of translation, and regulation of heterochromatin formation. Through a wide range of miRNA functional roles across the genome and transcriptome, miRNAs are involved in almost all biological processes, including during the developmental stages, cell differentiation, cell proliferation, cell death, metabolic regulation, transfusion silence, and antiviral defense. A number of miRNAs have aberrant expression patterns in ASD, and miRNAs are indeed involved in the regulation of ASD-related genes (Kinoshita et al. Citation2020).
Therefore, ASD and circadian rhythm are closely related, and this study aimed to investigate the association between ASD and circadian rhythm using miRNAs.
MicroRNAs influencing both circadian rhythm and ASD
Circadian rhythm-related miRNAs
The mammalian tissues and cells have autonomous 24-hour cycle oscillators to generate circadian rhythm. The circadian rhythm is generated by a transcription-translation feedback loop that is combined with post-transcription and post-translational modifications. The mechanism of a circadian clock system consists of several feedback loop systems that include transcription and translation steps. The first loop includes positive elements, such as CLOCK and BMAL1. The heterodimers of CLOCK and BMAL1 activate the transcription of target genes, including the E-box cis-modulating enhancer sequences, period (PER1-3), and cryptochrome (CRY1, 2) genes of the CLOCK family. Negative feedback is achieved by the PER and CRY heterodimer proteins acting on the CLOCK/BMAL1 complex in the nucleus to inhibit its transcription. CLOCK can be replaced by the neuron PAS protein 2 (NPAS2), an analog of CLOCK that dimerizes with BMAL1 to form transcriptionally active complexes (Landgraf et al. Citation2016). NPAS2 can compensate for the loss of CLOCK in peripheral cells and SCNs. The second loop includes retinoic acid-related orphan nuclear receptors (RORs), which work through enhancers of the ROR reaction element (RORE) and REV-ERB (Takahashi Citation2017).
These circadian clock genes are regulated at different levels including transcriptional, translational, post-transcriptional, and post-translational modifications (Treiber et al. Citation2019). Several miRNAs, in particular, target circadian-controlled genes and regulate their expression ().
Table 1. MicroRNAs that directly target circadian clock genes.
miRNA expression also can be controlled by the circadian system. CLOCK-BMAL1 directly regulates the expression of a number of miRNAs, including miR-219 (Cheng et al. Citation2007; Kojima et al. Citation2011). Additionally, in reaction to light, some miRNA, including miR-132, are activated by mitogen-activated protein kinase and a cAMP response element binding-dependent mechanism (Cheng et al. Citation2007; Kojima et al. Citation2011).
Direct transcriptional activation of miR-219 by CLOCK protein results in rhythmic miR-219 expression (Cheng et al. Citation2007). The level of miR-219 expression affects the length of the mouse circadian period (Cheng and Obrietan Citation2007; Cheng et al. Citation2007). The miR-183/96/182 cluster is highly expressed in the nervous system, especially in the sensory organs such as the inner ear and retina (Fan et al. Citation2017). Retinal degeneration occurs when the members of the miR-183/96/182 cluster are disrupted. The retina is not only a sensory organ but also a self-maintained 24-hour cycle clock. It is essential in setting the circadian rhythm as it is the only organ with light receptors and transports the signal to the SCN via the optic nerves (Zhou et al. Citation2021). Additionally, a photic signal induces miR-132 transcription in a CREB-dependent manner, which is followed by circadian time-dependent expression (Cheng et al. Citation2007). Translation regulatory genes and chromatin remodeling genes such as MECP2 are examples of mRNAs that miR-132 specifically targets (Alvarez-Saavedra et al. Citation2011). Through chromatin remodeling and protein translation, miR-132 then fine-tunes circadian rhythm entrainment. As a result, miR-132 has the potential to modify SCN’s capacity to relay photic signals for the production of other circadian-controlled genes.
In contrast, some miRNAs affect sleep by targeting the pineal glands of the brain. miR-132 is also associated with processes related to sleep control. In addition to miR-132, various miRNAs, such as miR-138, miR-let-7b, and miR-125a-5p, affect sleep in the brain (Davis et al. Citation2012). There are also reports that naturally occurring mutations of miR-182 target circadian-controlled genes, causing insomnia. Because genetic variations in the form of precursors of miR-182 cause major depression in patients with late insomnia, these miRNAs may be involved in 24-hour cycles and sleep functions (Saus et al. Citation2010). In addition, abnormal miRNAs such as miR-130a, miR-26a, miR-30c, and miR-let-7f are commonly detected in the plasma of patients with narcolepsy and idiopathic hypersomnia (Holm et al. Citation2014).
Melatonin is an important hormone that affects various bodily processes in the body and is secreted by the pineal gland in the brain (Kennaway and Wright Citation2002; Cipolla-Neto and Amaral Citation2018). Melatonin synthesis and secretion by pineal gland are under SCN control and are influenced by the circadian rhythm. Melatonin is synthesized from serotonin after the enzymatic actions of aryl alkyl amine N-acetyl transferase (AANAT) and acetylacetone O-methyltransferase (ASMT) (Rath et al. Citation2016). AANAT is the rate-limiting enzyme in melatonin synthesis pathways, and the circadian rhythm controls its expression (Coon et al. Citation1995). Melatonin synthesized by AANAT has a regulatory effect on certain pathways leading to diseases/disorders, such as cancer, brain conditions, liver fibrosis, ASD, and atherosclerosis, through functional interactions with specific miRNAs (Su et al. Citation2018). Several miRNAs, such as miR-483, can affect AANAT mRNA stability, AANAT activity level, and ultimately, melatonin level. The decrease in AANAT activity induced by miR-483 may reflect a decrease in AANAT protein expression (Coon et al. Citation1995).
Circadian-controlled genes inversely regulate the expression of several miRNAs (Ma et al. Citation2020). For example, 24-hour cycle initiators CLOCK and BMAL1 show a negative correlation with the 24-hour cycle expression patterns for miR-181d and miR-191. In contrast, the 24-hour periodic inhibitors PER, CRY, CKIe, and Rev-erb are positively correlated with miR-181d and miR-191 (Na et al. Citation2009).
ASD-related miRNAs
The majority of ASD cases appear to be brought on by mutations in any of the ASD risk genes and arise early in embryonic development (Fernandez and Scherer Citation2017). More than 100 gene mutations associated with brain development and neuronal activity have been identified in patients with ASD and are believed to be biomarkers for ASD (Liu et al. Citation2014; Herrero et al. Citation2020). ASD-related genetic variants have been identified in miRNA, miRNA biosynthesis, and miRNA target genes (Hu et al. Citation2017). And provides an overview of miRNAs exhibiting aberrant expression patterns linked to ASD ().
Table 2. Upward or down-regulated microRNAs in ASD patients.
Many studies have argued for the genetic pathologies of ASD, particularly those associated with synaptic cell adhesion molecules NLGN3, NLGN4, and NRXN1, and the postsynaptic scaffold protein SHANK3. One of the upstream factors that can control these genes might be circadian rhythm-controlled gene (Sarowar et al. Citation2016).
miR-146 is a strong candidate as an ASD biomarker since it has altered expression across a variety of tissues in individuals with autism. miR-153 is an important miRNA extensively studied in ASD, and it is shown that LEPR is a target gene of miR-153 in autism. miR-34 is another extensively studied miRNA in ASD. Recent studies have reported its vital role in neuronal development and disorders. Moreover, it has a strong influence on the regulation of MET, which has been reported as a risk gene in ASD. Other miRNAs, such as miR-106, miR-130, miR-320, and miR-451, have also shown altered expression levels in the brain and biofluids of individuals with ASD. The target genes (e.g. TGF-β, MECP2, NLGN3, PTEN, AUTS2, TSC1, SLITRK, NFkB, MAPK, AKT, ERK, and VEGF) of these miRNAs have been implicated in the pathogenesis of ASD as well as in neurodevelopment and neuronal functions (Vasu et al. Citation2019). hsa-miR-106b has been shown to be associated with autism and a variety of brain disorders (Zadehbagheri et al. Citation2019). Among the aforementioned ASD risk genes, TNRC6B, PTEN, AGO1, AGO2, SKI, and SMAD4 were the most commonly expressed, and are targeted by miR-92a-3p miRNAs for their regulation. In addition, this miRNA is involved with ASD risk genes and in a variety of pathways, including circadian rhythms, long-term depression, mTORs, and estrogen signaling pathways. miR-7-5p inhibits the expression of the ASD-related gene PAX6, an important transcription factor in neuronal tissue development that regulates dopaminergic neuronal differentiation (de Chevigny et al. Citation2012). Overexpression or inhibition of miR-7 and miR-504 also modulates the expression of the ASD risk gene Shank3 and affects the development of hippocampal neurons (Choi et al. Citation2015). miR-155 adversely affects the brain–blood-barrier function during neuroinflammation by targeting cell–cell complex molecules, such as AA2, claudin-1, and molecules that are critical in cell-to-extracellular matrix (ECM) interactions, including dedicators of cytokinesis 1 and syntenin-1 (Lopez-Ramirez et al. Citation2014). This means that miRNAs may contribute to the dysfunction of adherent junctions, the brain–blood barrier, and intestinal epithelial barrier in ASD (Fiorentino et al. Citation2016). Cell nutrient and energy detection by mTOR signaling regulate almost every aspect of metabolism and mitochondrial biosynthesis and play an important role in glucose homeostasis, lipid homeostasis, immune function, brain function, and cancer (Saxton and Sabatini Citation2017). miR-107 and miR-103 regulate insulin signaling and glucose homeostasis to help detect cellular nutrients and energy via mTOR signaling. The mTOR signal contains several ASD risk genes, including IGF1, MTOR, PIK3R2, PTEN, RHEB, TSC1, and TSC2. This may partly explain the causes of mitochondrial dysfunction and various clinical symptoms in ASD (Trajkovski et al. Citation2011).
Among the miRNAs mentioned above, those listed in are involved in both circadian rhythms and ASD. To explain the selected miRNAs, the most downregulated miR-219 in ASD patients was found to be involved in the control of the circadian rhythm in the SCN. miR-219 can directly target polo-like kinase 2 (PLK2), and PLK2 overexpression can reduce synaptic strength and neuroexcitability, leading to synaptic dysfunction in patients with ASD (Sarachana et al. Citation2010; Li et al. Citation2022). miR-219 is a target of the master circadian regulators CLOCK and BMAL1 (brain and muscle ANT-like 1) complexes, shows strong circadian rhythmic expression, and finely adjusts the length of the circadian rhythm in mice (Cheng et al. Citation2007; Kojima et al. Citation2011). In the case of miR-29b, it can influence the expression of Per1 and Per3 by directly targeting their 3′UTR (Mellios and Sur Citation2012; Hong et al. Citation2014; Zhao et al. Citation2014; Wu et al. Citation2020).
Table 3. MicroRNAs that are involved in the circadian rhythm and are abnormally controlled in patients with ASD.
Discussion
In this review, we have attempted to explain the effect of circadian rhythm on the expression of ASD-related genes through miRNAs. Circadian rhythms affect various processes such as growth, immunity, sleep patterns, and ASD. When the circadian-controlled gene that controls the circadian rhythm is mutated, the circadian rhythm can be disrupted, causing sleep disturbances. Many patients with ASD suffer from sleep disorders, and there have been studies that show that there is limited production and secretion of melatonin in their brains (Melke et al. Citation2008; Doyen et al. Citation2011; Rossignol and Frye Citation2011; Gringras et al. Citation2017; Wu et al. Citation2020; Martinez-Cayuelas et al. Citation2022). This is an example of how ASD is linked to abnormal sleep patterns and melatonin expression, and it could be an example of the relationship between circadian rhythm and ASD.
We hypothesized that ASD might be closely associated with a circadian rhythm disorder via miRNAs. In this work, we found that a number of miRNAs, including miR-219, miR-132, and miR-146, may serve as crucial linkages for circadian rhythm disruption in ASD patients. The miR-146 family consists of the two evolutionarily conserved miRNAs miR-146a and miR-146b (Williams et al. Citation2008; Matsumoto et al. Citation2016). Since they are expressed in neurons, miR-146a and miR-146b have a role in neuronal development and the control of inflammation in the nervous system (Nguyen et al. Citation2018; Chithanathan et al. Citation2022). According to one study, gene networks that miR-146a targets are connected to ASD and can be utilized to predict the type and severity of ASD expression in addition to just diagnosing the ASD presence (Nguyen et al. Citation2018). The circadian gene is also expected to have an impact on the expression of miR-146a (Wang et al. Citation2014). Other research has suggested that the circadian genes PER3, CRY1, and Timeless may be targeted and regulated by miR-146b (Dell'Osso et al. Citation2022). In conclusion, miR-146a and miR-146b expression can be influenced by circadian rhythms and is connected to ASD and neural development.
As summarized in , some miRNAs that control specific genes of the circadian clock system are also expressed abnormally in patients with ASD at the same time. However, we also believe that miRNAs that are transcriptionally regulated by the circadian rhythm or not mentioned in can directly or indirectly affect the expression of ASD inducers. Based on this, focusing on aspects of the circadian clock system, recent studies have shown that RORA-deficient mice exhibit limited behavior similar to mice with ASD, such as limited maze patrols, abnormal spatial learning, reduced search, and patience, compared to wild-type mice (Goodall and Gheusi Citation1987; Nguyen et al. Citation2010). RORA genes are dynamically regulated by several miRNAs, and miRNA-mediated RORA gene regulation may also affect ASD. For example, miR-18a negatively regulates RORA expression by binding to RORA’s 3′-UTR (Jiang et al. Citation2020), and it could also be an inducer of ASD. In conclusion, miRNA, which is transcriptionally regulated by the regulation of the clock gene of the circadian rhythm, might have a considerable effect on ASD. In addition to the known circadian-controlled or clock-gene-regulating miRNAs, additional miRNAs could be found, and their target genes and functions could be identified. The new targets and functions of previously reported miRNAs should also be investigated.
Although several miRNAs have been proposed to explain the association between circadian rhythm and ASD, studies have shown that ASD can be caused by various miRNAs. And in respect with ASD-related genes, behavioral tests using the SHANK2-KO mouse model with exon 6–7 deleted showed a decrease in interaction and social communication, memory deficit and spatial learning, hyperactivity, and anxiety-related behavior. This provides evidence that SHANK2 can cause ASD (Schmeisser et al. Citation2012). In this SHANK 2 gene, a single miR-137 binding site was identified: upregulation of miR-137 decreased SHANK2 expression level. miR-137 overexpression can induce ASD by downregulating SHANK2 (de Sena Cortabitarte et al. Citation2018). Furthermore, miR-137 has a potential association with circadian rhythm as well as with ASD. There have been studies reporting that miR-137 regulates Hypocretin (Hcrt) expression in the Hcrt neuronal cells that inhibits awakening. Hcrt neuropeptides regulate sleep and awakening stability, and Hcrt’s disorder can cause sleep disorders. Conversely, downregulation of miR-137 increases arousal in mice. The interaction between miR-137 and Hcrt is preserved across mice and humans, and studies have shown that miR-137 is genetically related to human sleep time (Holm et al. Citation2022). Another study found that miR-137 is involved in neuroplasticity by partially regulating glucocorticoid receptor-dependent signaling (Davis et al. Citation2012). Glucocorticoids form part of the awakening hormone cortisol that can affect the control of the circadian rhythm (Chung et al. Citation2011). In conclusion, miR-137 is a potential candidate that has the potential to affect gene expressions related to ASD, thereby, leading to ASD and to control sleep-waking rhythms to affect the circadian rhythm. Finding novel candidates such as miR-137 likely to be associated with circadian rhythm and ASD will be a challenge ahead.
miRNA transcription, Drosha and Dicer action, and RISC loading are important processes in miRNA production, and various factors promote, assist, or inhibit these processes (Treiber et al. Citation2019). In addition to studies that identify the function of miRNAs, recent studies have focused on regulators of miRNA expression (Debnath et al. Citation2017). Among various studies on miRNA-modulating substances, phytochemicals have been reported to play an important role in regulation of miRNA expression associated with changes in carcinogens, tumor inhibitors, and cancer-related protein expression. Therefore, identifying phytochemicals that can control the expression of miRNAs targeting the circadian clock and ASD-related genes might be valuable for future research. Further studies are needed to investigate the genetic effects of miRNAs associated with circadian rhythms in ASD. This can help researchers to develop treatment for ASD.
Acknowledgements
Conceptualization, K. H. Lee. and J. Y. Kim.; data curation, J. Y. Kim; writing – original draft preparation, J. Y. Kim.; writing – review and editing, K. H. Lee and W. Kim.; visualization, J. Y. Kim.; supervision, project administration and funding acquisition, K. H. Lee. All authors have read and agreed to the published version of the manuscript.
Disclosure statement
No potential conflict of interest was reported by the author(s).
Additional information
Funding
References
- Abu-Elneel K, Liu T, Gazzaniga FS, Nishimura Y, Wall DP, Geschwind DH, Lao K, Kosik KS. 2008. Heterogeneous dysregulation of microRNAs across the autism spectrum. Neurogenetics. 9(3):153–161.
- Alamdari AF, Rahnemayan S, Rajabi H, Vahed N, Kashani HRK, Rezabakhsh A, Sanaie S. 2021. Melatonin as a promising modulator of aging related neurodegenerative disorders: role of microRNAs. Pharmacol Res. 173:105839.
- Ali AAH, Tundo-Lavalle F, Hassan SA, Pfeffer M, Stahr A, von Gall C. 2020. Impact of targeted deletion of the circadian clock gene bmal1 in excitatory forebrain neurons on adult neurogenesis and olfactory function. Int J Mol Sci. 21:4.
- Alvarez-Saavedra M, Antoun G, Yanagiya A, Oliva-Hernandez R, Cornejo-Palma D, Perez-Iratxeta C, Sonenberg N, Cheng HY. 2011. miRNA-132 orchestrates chromatin remodeling and translational control of the circadian clock. Hum Mol Genet. 20(4):731–751.
- Ander BP, Barger N, Stamova B, Sharp FR, Schumann CM. 2015. Atypical miRNA expression in temporal cortex associated with dysregulation of immune, cell cycle, and other pathways in autism spectrum disorders. Mol Autism. 6:37.
- Arnes M, Kim YA, Lannes J, Alaniz ME, Cho JD, McCabe BD, Santa-Maria I. 2019. MiR-219 deficiency in Alzheimer’s disease contributes to neurodegeneration and memory dysfunction through post-transcriptional regulation of tau-kinase network. bioRxiv.607176.
- Baranova J, Dragunas G, Botellho M, Ayub ALP, Bueno-Alves R, Alencar RR, Papaiz DD, Sogayar MC, Ulrich H, Correa RG. 2021. Autism spectrum disorder: signaling pathways and prospective therapeutic targets. Cell Mol Neurobiol. 41(4):619–649.
- Bhatwadekar AD, Yan Y, Stepps V, Hazra S, Korah M, Bartelmez S, Chaqour B, Grant MB. 2015. miR-92a corrects CD34+ cell dysfunction in diabetes by modulating core circadian genes involved in progenitor differentiation. Diabetes. 64(12):4226–4237.
- Brzezinski A. 1997. Melatonin in humans. N Engl J Med. 336(3):186–195.
- Bu Y, Yoshida A, Chitnis N, Altman BJ, Tameire F, Oran A, Gennaro V, Armeson KE, McMahon SB, Wertheim GB. 2018. A PERK–miR-211 axis suppresses circadian regulators and protein synthesis to promote cancer cell survival. Nat Cell Biol. 20(1):104–115.
- Charrier A, Olliac B, Roubertoux P, Tordjman S. 2017. Clock genes and altered sleep-wake rhythms: their role in the development of psychiatric disorders. Int J Mol Sci. 18(5):938.
- Chatterjee N, Espinosa-Diez C, Anand S. 2020. A miR-494 dependent feedback loop regulates ER stress. bioRxiv.2020.2005.2012.088856.
- Chen R, D’Alessandro M, Lee C. 2013. miRNAs are required for generating a time delay critical for the circadian oscillator. Curr Biol. 23(20):1959–1968.
- Chen W, Liu Z, Li T, Zhang R, Xue Y, Zhong Y, Bai W, Zhou D, Zhao Z. 2014. Regulation of drosophila circadian rhythms by miRNA let-7 is mediated by a regulatory cycle. Nat Commun. 5(1):5549.
- Cheng HY, Obrietan K. 2007. Revealing a role of microRNAs in the regulation of the biological clock. Cell Cycle. 6(24):3034–3035.
- Cheng H-YM, Papp JW, Varlamova O, Dziema H, Russell B, Curfman JP, Nakazawa T, Shimizu K, Okamura H, Impey S, et al. 2007. microRNA modulation of circadian-clock period and entrainment. Neuron. 54(5):813–829.
- Chithanathan K, Somelar K, Jürgenson M, Žarkovskaja T, Periyasamy K, Yan L, Magilnick N, Boldin MP, Rebane A, Tian L, et al. 2022. Enhanced cognition and neurogenesis in miR-146b deficient mice. Cells. 11(13):2002.
- Choi SY, Pang K, Kim JY, Ryu JR, Kang H, Liu Z, Kim WK, Sun W, Kim H, Han K. 2015. Post-transcriptional regulation of SHANK3 expression by microRNAs related to multiple neuropsychiatric disorders. Mol Brain. 8(1):74.
- Chu C, Zhao Z. 2013. MicroRNA in the molecular mechanism of the circadian clock in mammals. FBL. 18(2):441–446.
- Chung S, Son GH, Kim K. 2011. Circadian rhythm of adrenal glucocorticoid: its regulation and clinical implications. Biochim Biophys Acta. 1812(5):581–591.
- Cipolla-Neto J, Amaral FGD. 2018. Melatonin as a hormone: new physiological and clinical insights. Endocr Rev. 39(6):990–1028.
- Coon SL, Roseboom PH, Baler R, Weller JL, Namboodiri MA, Koonin EV, Klein DC. 1995. Pineal serotonin N-acetyltransferase: expression cloning and molecular analysis. Science. 270(5242):1681–1683.
- Corbett BA, Mendoza S, Wegelin JA, Carmean V, Levine S. 2008. Variable cortisol circadian rhythms in children with autism and anticipatory stress. J Psychiatry Neurosci. 33(3):227–234.
- Corbett BA, Schupp CW, Levine S, Mendoza S. 2009. Comparing cortisol, stress, and sensory sensitivity in children with autism. Autism Res. 2(1):39–49.
- Daimiel-Ruiz L, Klett-Mingo M, Konstantinidou V, Micó V, Aranda JF, García B, Martínez-Botas J, Dávalos A, Fernández-Hernando C, Ordovás JM. 2015. Dietary lipids modulate the expression of miR-107, an miRNA that regulates the circadian system. Mol Nutr Food Res. 59(3):552–565.
- Dalgaard LT, Sorensen AE, Hardikar AA, Joglekar MV. 2022. The microRNA-29 family: role in metabolism and metabolic disease. Am J Physiol Cell Physiol. 323(2):C367–C377.
- Davis CJ, Clinton JM, Krueger JM. 2012. MicroRNA 138, let-7b, and 125a inhibitors differentially alter sleep and EEG delta-wave activity in rats. J Appl Physiol (1985). 113(11):1756–1762.
- Debnath T, Deb Nath NC, Kim EK, Lee KG. 2017. Role of phytochemicals in the modulation of miRNA expression in cancer. Food Funct. 8(10):3432–3442.
- de Chevigny A, Core N, Follert P, Gaudin M, Barbry P, Beclin C, Cremer H. 2012. miR-7a regulation of Pax6 controls spatial origin of forebrain dopaminergic neurons. Nat Neurosci. 15(8):1120–1126.
- Dell'Osso L, Massoni L, Battaglini S, Cremone IM, Carmassi C, Carpita B. 2022. Biological correlates of altered circadian rhythms, autonomic functions and sleep problems in autism spectrum disorder. Ann Gen Psychiatry. 21(1):13.
- de Paz AM, Sanchez-Mut JV, Samitier-Marti M, Petazzi P, Saez M, Szczesna K, Huertas D, Esteller M, Ausio J. 2015. Circadian cycle-dependent MeCP2 and brain chromatin changes. PLoS One. 10(4):e0123693.
- de Sena Cortabitarte A, Berkel S, Cristian FB, Fischer C, Rappold GA. 2018. A direct regulatory link between microRNA-137 and SHANK2: implications for neuropsychiatric disorders. J Neurodev Disord. 10(1):15.
- Dibner C, Schibler U, Albrecht U. 2010. The mammalian circadian timing system: organization and coordination of central and peripheral clocks. Annu Rev Physiol. 72:517–549. eng.
- Ding H, Huang Z, Chen M, Wang C, Chen X, Chen J, Zhang J. 2016. Identification of a panel of five serum miRNAs as a biomarker for Parkinson’s disease. Parkinsonism Relat Disord. 22:68–73.
- Dolan BM, Duron SG, Campbell DA, Vollrath B, Shankaranarayana Rao BS, Ko HY, Lin GG, Govindarajan A, Choi SY, Tonegawa S. 2013. Rescue of fragile X syndrome phenotypes in Fmr1 KO mice by the small-molecule PAK inhibitor FRAX486. Proc Natl Acad Sci U S A. 110(14):5671–5676.
- Doyen C, Mighiu D, Kaye K, Colineaux C, Beaumanoir C, Mouraeff Y, Rieu C, Paubel P, Contejean Y. 2011. Melatonin in children with autistic spectrum disorders: recent and practical data. Eur Child Adolesc Psychiatry. 20(5):231–239.
- Du NH, Arpat AB, De Matos M, Gatfield D. 2014. MicroRNAs shape circadian hepatic gene expression on a transcriptome-wide scale. Elife. 3:e02510.
- Duffield GE, Watson NP, Mantani A, Peirson SN, Robles-Murguia M, Loros JJ, Israel MA, Dunlap JC. 2009. A role for Id2 in regulating photic entrainment of the mammalian circadian system. Curr Biol. 19(4):297–304.
- Dumortier O, Hinault C, Van Obberghen E. 2013. MicroRNAs and metabolism crosstalk in energy homeostasis. Cell Metab. 18(3):312–324.
- Elzein S, Goodyer CG. 2014. Regulation of human growth hormone receptor expression by microRNAs. Mol Endocrinol. 28(9):1448–1459.
- Fan J, Jia L, Li Y, Ebrahim S, May-Simera H, Wood A, Morell RJ, Liu P, Lei J, Kachar B, et al. 2017. Maturation arrest in early postnatal sensory receptors by deletion of the miR-183/96/182 cluster in mouse. Proc Natl Acad Sci U S A. 114(21):E4271–E4280.
- Fernandez BA, Scherer SW. 2017. Syndromic autism spectrum disorders: moving from a clinically defined to a molecularly defined approach. Dialogues Clin Neurosci. 19(4):353–371.
- Fernández-Santiago R, Iranzo A, Gaig C, Serradell M, Fernández M, Tolosa E, Santamaría J, Ezquerra M. 2015. Micro RNA association with synucleinopathy conversion in rapid eye movement behavior disorder. Ann Neurol. 77(5):895–901.
- Fiorentino M, Sapone A, Senger S, Camhi SS, Kadzielski SM, Buie TM, Kelly DL, Cascella N, Fasano A. 2016. Blood-brain barrier and intestinal epithelial barrier alterations in autism spectrum disorders. Mol Autism. 7:49.
- Ganesan H, Balasubramanian V, Iyer M, Venugopal A, Subramaniam MD, Cho SG, Vellingiri B. 2019. mTOR signalling pathway – a root cause for idiopathic autism? BMB Rep. 52(7):424–433.
- Gao Q, Zhou L, Yang SY, Cao JM. 2016. A novel role of microRNA 17-5p in the modulation of circadian rhythm. Sci Rep. 6:30070. eng.
- Ghahramani Seno MM, Hu P, Gwadry FG, Pinto D, Marshall CR, Casallo G, Scherer SW. 2011. Gene and miRNA expression profiles in autism spectrum disorders. Brain Res. 1380:85–97.
- Goodall G, Gheusi G. 1987. Abnormal patterns of maze patrolling in the mutant mouse staggerer. Behav Neural Biol. 47(3):307–320.
- Gringras P, Nir T, Breddy J, Frydman-Marom A, Findling RL. 2017. Efficacy and safety of pediatric prolonged-release melatonin for insomnia in children with autism spectrum disorder. J Am Acad Child Adolesc Psychiatry. 56(11):948–957e944.
- Gunnar MR, Vazquez DM. 2001. Low cortisol and a flattening of expected daytime rhythm: potential indices of risk in human development. Dev Psychopathol. 13(3):515–538.
- Guo X, Zhu Y, Hong X, Zhang M, Qiu X, Wang Z, Qi Z, Hong X. 2017. miR-181d and c-myc-mediated inhibition of CRY2 and FBXL3 reprograms metabolism in colorectal cancer. Cell Death Dis. 8(7):e2958.
- Halepoto DM, Bashir S, L-Ayadhi LA. 2014. Possible role of brain-derived neurotrophic factor (BDNF) in autism spectrum disorder: current status. J Coll Physicians Surg Pak. 24(4):274–278.
- Herrero MJ, Velmeshev D, Hernandez-Pineda D, Sethi S, Sorrells S, Banerjee P, Sullivan C, Gupta AR, Kriegstein AR, Corbin JG. 2020. Identification of amygdala-expressed genes associated with autism spectrum disorder. Mol Autism. 11(1):39.
- Hicks SD, Ignacio C, Gentile K, Middleton FA. 2016. Salivary miRNA profiles identify children with autism spectrum disorder, correlate with adaptive behavior, and implicate ASD candidate genes involved in neurodevelopment. BMC Pediatr. 16:52.
- Holm A, Bang-Berthelsen CH, Knudsen S, Kornum BR, Modvig S, Jennum P, Gammeltoft S. 2014. miRNA profiles in plasma from patients with sleep disorders reveal dysregulation of miRNAs in narcolepsy and other central hypersomnias. Sleep. 37(9):1525–1533.
- Holm A, Possovre ML, Bandarabadi M, Moseholm KF, Justinussen JL, Bozic I, Lemcke R, Arribat Y, Amati F, Silahtaroglu A, et al. 2022. The evolutionarily conserved miRNA-137 targets the neuropeptide hypocretin/orexin and modulates the wake to sleep ratio. Proc Natl Acad Sci U S A. 119(17):e2112225119.
- Hong Z, Feng Z, Sai Z, Tao S. 2014. PER3, a novel target of miR-103, plays a suppressive role in colorectal cancer in vitro. BMB Rep. 47(9):500–505.
- Horii R, Honda M, Shirasaki T, Shimakami T, Shimizu R, Yamanaka S, Murai K, Kawaguchi K, Arai K, Yamashita T, et al. 2019. MicroRNA-10a impairs liver metabolism in hepatitis C virus-related cirrhosis through deregulation of the circadian clock gene brain and muscle aryl hydrocarbon receptor nuclear translocator-like 1. Hepatol Commun. 3(12):1687–1703.
- Hu Y, Ehli EA, Boomsma DI. 2017. MicroRNAs as biomarkers for psychiatric disorders with a focus on autism spectrum disorder: current progress in genetic association studies, expression profiling, and translational research. Autism Res. 10(7):1184–1203.
- Huang F, Long Z, Chen Z, Li J, Hu Z, Qiu R, Zhuang W, Tang B, Xia K, Jiang H. 2015. Investigation of gene regulatory networks associated with autism spectrum disorder based on MiRNA expression in China. PLoS One. 10(6):e0129052.
- Hughes JR. 2009. Update on autism: a review of 1300 reports published in 2008. Epilepsy Behav. 16(4):569–589.
- Iakoucheva LM, Muotri AR, Sebat J. 2019. Getting to the cores of autism. Cell. 178(6):1287–1298.
- Ingiosi AM, Schoch H, Wintler T, Singletary KG, Righelli D, Roser LG, Medina E, Risso D, Frank MG, Peixoto L. 2019. Shank3 modulates sleep and expression of circadian transcription factors. Elife. 8:e42819.
- Iwakawa HO, Tomari Y. 2015. The functions of MicroRNAs: mRNA decay and translational repression. Trends Cell Biol. 25(11):651–665.
- Jiang W, Zhao S, Shen J, Guo L, Sun Y, Zhu Y, Ma Z, Zhang X, Hu Y, Xiao W, et al. 2018. The MiR-135b–BMAL1–YY1 loop disturbs pancreatic clockwork to promote tumourigenesis and chemoresistance. Cell Death Dis. 9(2):149.
- Jiang Y, Zhou J, Zhao J, Hou D, Zhang H, Li L, Zou D, Hu J, Zhang Y, Jing Z. 2020. MiR-18a-downregulated RORA inhibits the proliferation and tumorigenesis of glioma using the TNF-alpha-mediated NF-kappaB signaling pathway. EBioMedicine. 52:102651.
- Jin Y, Choi J, Won J, Hong Y. 2018. The relationship between autism spectrum disorder and melatonin during fetal development. Molecules. 23(1):198.
- Jyonouchi H, Geng L, Streck DL, Dermody JJ, Toruner GA. 2017. MicroRNA expression changes in association with changes in interleukin-1ss/interleukin10 ratios produced by monocytes in autism spectrum disorders: their association with neuropsychiatric symptoms and comorbid conditions (observational study). J Neuroinflammation. 14(1):229.
- Kas MJ, Glennon JC, Buitelaar J, Ey E, Biemans B, Crawley J, Ring RH, Lajonchere C, Esclassan F, Talpos J, et al. 2014. Assessing behavioural and cognitive domains of autism spectrum disorders in rodents: current status and future perspectives. Psychopharmacology (Berl). 231(6):1125–1146.
- Kennaway DJ, Wright H. 2002. Melatonin and circadian rhythms. Curr Top Med Chem. 2(2):199–209.
- Kim VN, Han J, Siomi MC. 2009. Biogenesis of small RNAs in animals. Nat Rev Mol Cell Biol. 10(2):126–139.
- Kinoshita C, Okamoto Y, Aoyama K, Nakaki T. 2020. MicroRNA: a key player for the interplay of circadian rhythm abnormalities, sleep disorders and neurodegenerative diseases. Clocks Sleep. 2(3):282–307.
- Knarr M, Nagaraj AB, Kwiatkowski LJ, DiFeo A. 2019a. miR-181a modulates circadian rhythm in immortalized bone marrow and adipose derived stromal cells and promotes differentiation through the regulation of PER3. Sci Rep. 9(1):1–13.
- Knarr M, Nagaraj AB, Kwiatkowski LJ, DiFeo A. 2019b. miR-181a modulates circadian rhythm in immortalized bone marrow and adipose derived stromal cells and promotes differentiation through the regulation of PER3. Sci Rep. 9(1):307.
- Kochan DZ, Ilnytskyy Y, Golubov A, Deibel SH, McDonald RJ, Kovalchuk O. 2015. Circadian disruption-induced microRNAome deregulation in rat mammary gland tissues. Oncoscience. 2(4):428–442. eng.
- Kojima S, Shingle DL, Green CB. 2011. Post-transcriptional control of circadian rhythms. J Cell Sci. 124(Pt 3):311–320.
- Landgraf D, Wang LL, Diemer T, Welsh DK. 2016. NPAS2 compensates for loss of CLOCK in peripheral circadian oscillators. PLoS Genet. 12(2):e1005882.
- Lee K-H, Kim S-H, Lee H-R, Kim W, Kim D-Y, Shin J-C, Yoo S-H, Kim K-T. 2013. MicroRNA-185 oscillation controls circadian amplitude of mouse Cryptochrome 1 via translational regulation. Mol Biol Cell. 24(14):2248–2255.
- Lee SH, Kim EY. 2021. Short-term maintenance on a high-sucrose diet alleviates aging-induced sleep fragmentation in drosophila. Anim Cells Syst (Seoul). 25(6):377–386.
- Lewis MH, Tanimura Y, Lee LW, Bodfish JW. 2007. Animal models of restricted repetitive behavior in autism. Behav Brain Res. 176(1):66–74.
- Li A, Lin X, Tan X, Yin B, Han W, Zhao J, Yuan J, Qiang B, Peng X. 2013. Circadian gene clock contributes to cell proliferation and migration of glioma and is directly regulated by tumor-suppressive miR-124. FEBS Lett. 587(15):2455–2460.
- Li J, Xu X, Liu J, Zhang S, Tan X, Li Z, Zhang J, Wang Z. 2022. Decoding microRNAs in autism spectrum disorder. Mol Ther Nucleic Acids. 30:535–546.
- Liang J, Liu C, Qiao A, Cui Y, Zhang H, Cui A, Zhang S, Yang Y, Xiao X, Chen Y, et al. 2013. MicroRNA-29a-c decrease fasting blood glucose levels by negatively regulating hepatic gluconeogenesis. J Hepatol. 58(3):535–542.
- Liu D, Nanclares C, Simbriger K, Fang K, Lorsung E, Le N, Amorim IS, Chalkiadaki K, Pathak SS, Li J, et al. 2022a. Autistic-like behavior and cerebellar dysfunction in Bmal1 mutant mice ameliorated by mTORC1 inhibition. Mol Psychiatry.
- Liu L, Lei J, Sanders SJ, Willsey AJ, Kou Y, Cicek AE, Klei L, Lu C, He X, Li M, et al. 2014. DAWN: a framework to identify autism genes and subnetworks using gene expression and genetics. Mol Autism. 5(1):22.
- Liu Q, Di R, Ren C, He X, Wang X, Xia Q, Chu M, Zhang Z. 2022b. Screening of differentially expressed genes and miRNAs in hypothalamus and pituitary gland of sheep under different photoperiods. Genes (Basel). 13(6):1091.
- Lopez-Ramirez MA, Wu D, Pryce G, Simpson JE, Reijerkerk A, King-Robson J, Kay O, de Vries HE, Hirst MC, Sharrack B, et al. 2014. MicroRNA-155 negatively affects blood-brain barrier function during neuroinflammation. FASEB J. 28(6):2551–2565.
- Lord C, Brugha TS, Charman T, Cusack J, Dumas G, Frazier T, Jones EJH, Jones RM, Pickles A, State MW, et al. 2020. Autism spectrum disorder. Nat Rev Dis Primers. 6(1):5.
- Lundy S. 2018. A possible role for the circadian clock in chlamydial pathogenesis [M.S.B.R.]. Ann Arbor: Morehouse School of Medicine.
- Ma Q, Mo G, Tan Y. 2020. Micro RNAs and the biological clock: a target for diseases associated with a loss of circadian regulation. Afr Health Sci. 20(4):1887–1894. eng.
- Malow BA, Marzec ML, McGrew SG, Wang L, Henderson LM, Stone WL. 2006. Characterizing sleep in children with autism spectrum disorders: a multidimensional approach. Sleep. 29(12):1563–1571.
- Martinez I, Cazalla D, Almstead LL, Steitz JA, DiMaio D. 2011. miR-29 and miR-30 regulate B-Myb expression during cellular senescence. Proc Natl Acad Sci USA. 108(2):522–527.
- Martinez-Cayuelas E, Gavela-Perez T, Rodrigo-Moreno M, Merino-Andreu M, Vales-Villamarin C, Perez-Nadador I, Garces C, Soriano-Guillen L. 2022. Melatonin rhythm and its relation to sleep and circadian parameters in children and adolescents with autism spectrum disorder. Front Neurol. 13:813692.
- Matamala JM, Arias-Carrasco R, Sanchez C, Uhrig M, Bargsted L, Matus S, Maracaja-Coutinho V, Abarzua S, van Zundert B, Verdugo R, et al. 2018. Genome-wide circulating microRNA expression profiling reveals potential biomarkers for amyotrophic lateral sclerosis. Neurobiol Aging. 64:123–138.
- Matsumoto CS, Almeida LO, Guimarães DM, Martins MD, Papagerakis P, Papagerakis S, Leopoldino AM, Castilho RM, Squarize CH. 2016. PI3K-PTEN dysregulation leads to mTOR-driven upregulation of the core clock gene BMAL1 in normal and malignant epithelial cells. Oncotarget. 7(27):42393.
- Mauvoisin D, Wang J, Jouffe C, Martin E, Atger F, Waridel P, Quadroni M, Gachon F, Naef F. 2014. Circadian clock-dependent and-independent rhythmic proteomes implement distinct diurnal functions in mouse liver. Proc Natl Acad Sci USA. 111(1):167–172.
- Mehta N, Cheng HY. 2013. Micro-managing the circadian clock: the role of microRNAs in biological timekeeping. J Mol Biol. 425(19):3609–3624.
- Melke J, Goubran Botros H, Chaste P, Betancur C, Nygren G, Anckarsater H, Rastam M, Stahlberg O, Gillberg IC, Delorme R, et al. 2008. Abnormal melatonin synthesis in autism spectrum disorders. Mol Psychiatry. 13(1):90–98.
- Mellios N, Sur M. 2012. The emerging role of microRNAs in schizophrenia and autism spectrum disorders. Front Psychiatry. 3:39.
- Mor M, Nardone S, Sams DS, Elliott E. 2015. Hypomethylation of miR-142 promoter and upregulation of microRNAs that target the oxytocin receptor gene in the autism prefrontal cortex. Mol Autism. 6:46.
- Morrissey MJ, Duntley SP, Anch AM, Nonneman R. 2004. Active sleep and its role in the prevention of apoptosis in the developing brain. Med Hypotheses. 62(6):876–879.
- Muller M, Fazi F, Ciaudo C. 2019. Argonaute proteins: from structure to function in development and pathological cell fate determination. Front Cell Dev Biol. 7:360.
- Mundalil Vasu M, Anitha A, Thanseem I, Suzuki K, Yamada K, Takahashi T, Wakuda T, Iwata K, Tsujii M, Sugiyama T, et al. 2014. Serum microRNA profiles in children with autism. Mol Autism. 5:40.
- Na Y-J, Sung JH, Lee SC, Lee Y-J, Choi YJ, Park W-Y, Shin HS, Kim JH. 2009. Comprehensive analysis of microRNA-mRNA co-expression in circadian rhythm. Exp Mol Med. 41(9):638–647.
- Nagarajan RP, Hogart AR, Gwye Y, Martin MR, LaSalle JM. 2006. Reduced MeCP2 expression is frequent in autism frontal cortex and correlates with aberrant MECP2 promoter methylation. Epigenetics. 1(4):e1–11.
- Nelson PT, Wang W-X. 2010. MiR-107 is reduced in Alzheimer’s disease brain neocortex: validation study. J Alzheimer's Dis. 21(1):75–79.
- Nguyen A, Rauch TA, Pfeifer GP, Hu VW. 2010. Global methylation profiling of lymphoblastoid cell lines reveals epigenetic contributions to autism spectrum disorders and a novel autism candidate gene, RORA, whose protein product is reduced in autistic brain. FASEB J. 24(8):3036–3051.
- Nguyen LS, Fregeac J, Bole-Feysot C, Cagnard N, Iyer A, Anink J, Aronica E, Alibeu O, Nitschke P, Colleaux L. 2018. Role of miR-146a in neural stem cell differentiation and neural lineage determination: relevance for neurodevelopmental disorders. Mol Autism. 9:38–38. eng.
- Nguyen LS, Lepleux M, Makhlouf M, Martin C, Fregeac J, Siquier-Pernet K, Philippe A, Feron F, Gepner B, Rougeulle C, et al. 2016. Profiling olfactory stem cells from living patients identifies miRNAs relevant for autism pathophysiology. Mol Autism. 7:1.
- Nicholas B, Rudrasingham V, Nash S, Kirov G, Owen MJ, Wimpory DC. 2007. Association of Per1 and Npas2 with autistic disorder: support for the clock genes/social timing hypothesis. Mol Psychiatry. 12(6):581–592.
- O'Brien J, Hayder H, Zayed Y, Peng C. 2018. Overview of MicroRNA biogenesis, mechanisms of actions, and circulation. Front Endocrinol (Lausanne). 9:402.
- O'Connell RM, Rao DS, Chaudhuri AA, Boldin MP, Taganov KD, Nicoll J, Paquette RL, Baltimore D. 2008. Sustained expression of microRNA-155 in hematopoietic stem cells causes a myeloproliferative disorder. J Exp Med. 205(3):585–594.
- Ortega MA, Alvarez-Mon MA, Garcia-Montero C, Fraile-Martinez O, Lahera G, Monserrat J, Munoz-Merida L, Mora F, Rodriguez-Jimenez R, Fernandez-Rojo S, et al. 2021. MicroRNAs as critical biomarkers of major depressive disorder: a comprehensive perspective. Biomedicines. 9(11):1659.
- Oyama Y, Bartman CM, Gile J, Eckle T. 2017. Circadian MicroRNAs in cardioprotection. Curr Pharm Des. 23(25):3723–3730.
- Park HJ, Jung H. 2022. Neuro-immune interactions at single-cell resolution in neurodevelopmental, infectious, and neurodegenerative diseases. Anim Cells Syst (Seoul. 26(4):137–147.
- Park I, Kim D, Kim J, Jang S, Choi M, Choe HK, Choe Y, Kim K. 2020. microRNA-25 as a novel modulator of circadian Period2 gene oscillation. Exp Mol Med. 52(9):1614–1626.
- Patke A, Young MW, Axelrod S. 2020. Molecular mechanisms and physiological importance of circadian rhythms. Nat Rev Mol Cell Biol. 21(2):67–84.
- Qiu J, Zhang J, Zhou Y, Li X, Li H, Liu J, Gou K, Zhao J, Cui S. 2019. MicroRNA-7 inhibits melatonin synthesis by acting as a linking molecule between leptin and norepinephrine signaling pathways in pig pineal gland. J Pineal Res. 66(3):e12552.
- Raheja R, Regev K, Healy BC, Mazzola MA, Beynon V, Von Glehn F, Paul A, Diaz-Cruz C, Gholipour T, Glanz BI. 2018. Correlating serum micrornas and clinical parameters in amyotrophic lateral sclerosis. Muscle Nerve. 58(2):261–269.
- Rath MF, Coon SL, Amaral FG, Weller JL, Moller M, Klein DC. 2016. Melatonin synthesis: acetylserotonin O-methyltransferase (ASMT) is strongly expressed in a subpopulation of pinealocytes in the male rat pineal gland. Endocrinology. 157(5):2028–2040.
- Ren LR, Yao RB, Wang SY, Gong XD, Xu JT, Yang KS. 2021. MiR-27a-3p promotes the osteogenic differentiation by activating CRY2/ERK1/2 axis. Mol Med. 27(1):43.
- Ritvo ER, Ritvo R, Yuwiler A, Brothers A, Freeman BJ, Plotkin S. 1993. Elevated daytime melatonin concentrations in autism: a pilot study. Eur Child Adolesc Psychiatry. 2(2):75–78.
- Rossignol DA, Frye RE. 2011. Melatonin in autism spectrum disorders: a systematic review and meta-analysis. Dev Med Child Neurol. 53(9):783–792.
- Rylaarsdam L, Guemez-Gamboa A. 2019. Genetic causes and modifiers of autism spectrum disorder. Front Cell Neurosci. 13:385.
- Sabaie H, Dehghani H, Shiva S, Asadi MR, Rezaei O, Taheri M, Rezazadeh M. 2021. Mechanistic insight into the regulation of immune-related genes expression in autism spectrum disorder. Front Mol Biosci. 8:754296.
- Sarachana T, Zhou R, Chen G, Manji HK, Hu VW. 2010. Investigation of post-transcriptional gene regulatory networks associated with autism spectrum disorders by microRNA expression profiling of lymphoblastoid cell lines. Genome Med. 2(4):23. eng.
- Sare RM, Harkless L, Levine M, Torossian A, Sheeler CA, Smith CB. 2017. Deficient sleep in mouse models of fragile X syndrome. Front Mol Neurosci. 10:280.
- Sarowar T, Chhabra R, Vilella A, Boeckers TM, Zoli M, Grabrucker AM. 2016. Activity and circadian rhythm influence synaptic Shank3 protein levels in mice. J Neurochem. 138(6):887–895.
- Saus E, Soria V, Escaramis G, Vivarelli F, Crespo JM, Kagerbauer B, Menchon JM, Urretavizcaya M, Gratacos M, Estivill X. 2010. Genetic variants and abnormal processing of pre-miR-182, a circadian clock modulator, in major depression patients with late insomnia. Hum Mol Genet. 19(20):4017–4025.
- Saxton RA, Sabatini DM. 2017. mTOR signaling in growth, metabolism, and disease. Cell. 168(6):960–976.
- Schmeisser MJ, Ey E, Wegener S, Bockmann J, Stempel AV, Kuebler A, Janssen AL, Udvardi PT, Shiban E, Spilker C, et al. 2012. Autistic-like behaviours and hyperactivity in mice lacking ProSAP1/Shank2. Nature. 486(7402):256–260.
- Schroeder M, Jakovcevski M, Polacheck T, Drori Y, Luoni A, Röh S, Zaugg J, Ben-Dor S, Albrecht C, Chen A. 2018. Placental miR-340 mediates vulnerability to activity based anorexia in mice. Nat Commun. 9(1):1596.
- Seeburg DP, Sheng M. 2008. Activity-induced polo-like kinase 2 is required for homeostatic plasticity of hippocampal neurons during epileptiform activity. J Neurosci. 28(26):6583–6591.
- Serafini G, Pompili M, Hansen KF, Obrietan K, Dwivedi Y, Shomron N, Girardi P. 2014. The involvement of microRNAs in major depression, suicidal behavior, and related disorders: a focus on miR-185 and miR-491-3p. Cell Mol Neurobiol. 34(1):17–30.
- Shende VR, Kim S-M, Neuendorff N, Earnest DJ. 2014. MicroRNAs function as cis- and trans-acting modulators of peripheral circadian clocks. FEBS Lett. 588(17):3015–3022.
- Shin DW. 2020. Various biological effects of solar radiation on skin and their mechanisms: implications for phototherapy. Anim Cells Syst (Seoul. 24(4):181–188.
- Shu J, Silva B, Gao T, Xu Z, Cui J. 2017. Dynamic and modularized microRNA regulation and its implication in human cancers. Sci Rep. 7(1):13356.
- Singla R, Mishra A, Lin H, Lorsung E, Le N, Tin S, Jin VX, Cao R. 2022. Haploinsufficiency of a circadian clock gene Bmal1 (arntl or Mop3) causes brain-wide mTOR hyperactivation and autism-like behavioral phenotypes in mice. Int J Mol Sci. 23(11):6317.
- Spencer CM, Alekseyenko O, Hamilton SM, Thomas AM, Serysheva E, Yuva-Paylor LA, Paylor R. 2011. Modifying behavioral phenotypes in Fmr1KO mice: genetic background differences reveal autistic-like responses. Autism Res. 4(1):40–56.
- Su SC, Reiter RJ, Hsiao HY, Chung WH, Yang SF. 2018. Functional interaction between melatonin signaling and noncoding RNAs. Trends Endocrinol Metab. 29(6):435–445.
- Sulli G, Lam MTY, Panda S. 2019. Interplay between circadian clock and cancer: new frontiers for cancer treatment. Trends Cancer. 5(8):475–494.
- Sulli G, Manoogian ENC, Taub PR, Panda S. 2018. Training the circadian clock, clocking the drugs, and drugging the clock to prevent, manage, and treat chronic diseases. Trends Pharmacol Sci. 39(9):812–827. eng.
- Takahashi JS. 2017. Transcriptional architecture of the mammalian circadian clock. Nat Rev Genet. 18(3):164–179.
- Talebizadeh Z, Butler MG, Theodoro MF. 2008. Feasibility and relevance of examining lymphoblastoid cell lines to study role of microRNAs in autism. Autism Res. 1(4):240–250.
- Tamura H, Nakamura Y, Terron MP, Flores LJ, Manchester LC, Tan DX, Sugino N, Reiter RJ. 2008. Melatonin and pregnancy in the human. Reprod Toxicol. 25(3):291–303.
- Tang Z, Xu T, Li Y, Fei W, Yang G, Hong Y. 2020. Inhibition of CRY2 by STAT3/miRNA-7-5p promotes osteoblast differentiation through upregulation of CLOCK/BMAL1/P300 expression. Mol Ther Nucleic Acids. 19:865–876.
- Testa U, Pelosi E, Castelli G, Labbaye C. 2017. miR-146 and miR-155: two key modulators of immune response and tumor development. Noncoding RNA. 3(3):22.
- Toma C, Torrico B, Hervas A, Salgado M, Rueda I, Valdes-Mas R, Buitelaar JK, Rommelse N, Franke B, Freitag C, et al. 2015. Common and rare variants of microRNA genes in autism spectrum disorders. World J Biol Psychiatry. 16(6):376–386.
- Tonacci A, Bagnato G, Pandolfo G, Billeci L, Sansone F, Conte R, Gangemi S. 2019. MicroRNA cross-involvement in autism spectrum disorders and atopic dermatitis: a literature review. J Clin Med. 8(1):88.
- Tordjman S, Anderson GM, Bellissant E, Botbol M, Charbuy H, Camus F, Graignic R, Kermarrec S, Fougerou C, Cohen D, et al. 2012. Day and nighttime excretion of 6-sulphatoxymelatonin in adolescents and young adults with autistic disorder. Psychoneuroendocrinology. 37(12):1990–1997.
- Tordjman S, Anderson GM, Pichard N, Charbuy H, Touitou Y. 2005. Nocturnal excretion of 6-sulphatoxymelatonin in children and adolescents with autistic disorder. Biol Psychiatry. 57(2):134–138.
- Tordjman S, Najjar I, Bellissant E, Anderson GM, Barburoth M, Cohen D, Jaafari N, Schischmanoff O, Fagard R, Lagdas E, et al. 2013. Advances in the research of melatonin in autism spectrum disorders: literature review and new perspectives. Int J Mol Sci. 14(10):20508–20542.
- Trajkovski M, Hausser J, Soutschek J, Bhat B, Akin A, Zavolan M, Heim MH, Stoffel M. 2011. MicroRNAs 103 and 107 regulate insulin sensitivity. Nature. 474(7353):649–653.
- Treiber T, Treiber N, Meister G. 2019. Regulation of microRNA biogenesis and its crosstalk with other cellular pathways. Nat Rev Mol Cell Biol. 20(1):5–20.
- Trickett J, Heald M, Oliver C, Richards C. 2018. A cross-syndrome cohort comparison of sleep disturbance in children with Smith-Magenis syndrome, Angelman syndrome, autism spectrum disorder and tuberous sclerosis complex. J Neurodev Disord. 10(1):9.
- Uwatoko H, Hama Y, Iwata IT, Shirai S, Matsushima M, Yabe I, Utsumi J, Sasaki H. 2019. Identification of plasma microRNA expression changes in multiple system atrophy and Parkinson’s disease. Mol Brain. 12(1):49.
- Vasu MM, Sumitha PS, Rahna P, Thanseem I, Anitha A. 2019. microRNAs in autism spectrum disorders. Curr Pharm Des. 25(41):4368–4378.
- Voiculescu SE, Zygouropoulos N, Zahiu CD, Zagrean AM. 2014. Role of melatonin in embryo fetal development. J Med Life. 7(4):488–492.
- Wang Q, Bozack SN, Yan Y, Boulton ME, Grant MB, Busik JV. 2014. Regulation of retinal inflammation by rhythmic expression of MiR-146a in diabetic retina. Invest Ophthalmol Vis Sci. 55(6):3986–3994.
- Williams AE, Perry MM, Moschos SA, Larner-Svensson HM, Lindsay MA. 2008. Role of miRNA-146a in the regulation of the innate immune response and cancer. Biochem Soc Trans. 36(Pt 6):1211–1215.
- Williams SM, An JY, Edson J, Watts M, Murigneux V, Whitehouse AJO, Jackson CJ, Bellgrove MA, Cristino AS, Claudianos C. 2019. An integrative analysis of non-coding regulatory DNA variations associated with autism spectrum disorder. Mol Psychiatry. 24(11):1707–1719.
- Woodbury ME, Freilich RW, Cheng CJ, Asai H, Ikezu S, Boucher JD, Slack F, Ikezu T. 2015. miR-155 is essential for inflammation-induced hippocampal neurogenic dysfunction. J Neurosci. 35(26):9764–9781. eng.
- Wrigley S, Arafa D, Tropea D. 2017. Insulin-like growth factor 1: at the crossroads of brain development and aging. Front Cell Neurosci. 11:14.
- Wu X, Li W, Zheng Y. 2020. Recent progress on relevant microRNAs in autism spectrum disorders. Int J Mol Sci. 21:16.
- Wu YE, Parikshak NN, Belgard TG, Geschwind DH. 2016. Genome-wide, integrative analysis implicates microRNA dysregulation in autism spectrum disorder. Nat Neurosci. 19(11):1463–1476.
- Wu ZY, Huang SD, Zou JJ, Wang QX, Naveed M, Bao HN, Wang W, Fukunaga K, Han F. 2020. Autism spectrum disorder (ASD): disturbance of the melatonin system and its implications. Biomed Pharmacother. 130:110496.
- Yang Y, Sun B, Huang J, Xu L, Pan J, Fang C, Li M, Li G, Tao Y, Yang X, et al. 2017. Up-regulation of miR-325-3p suppresses pineal aralkylamine N-acetyltransferase (Aanat) after neonatal hypoxia-ischemia brain injury in rats. Brain Res. 1668:28–35.
- Yang Z, Matsumoto A, Nakayama K, Jimbo EF, Kojima K, Nagata K, Iwamoto S, Yamagata T. 2016. Circadian-relevant genes are highly polymorphic in autism spectrum disorder patients. Brain Dev. 38(1):91–99.
- Yenen AS, Çak HT. 2020. Melatonin and circadian rhythm in autism spectrum disorders. Turk Psikiyatri Derg. 31(3):201–211. eng.
- Yoo SH, Kojima S, Shimomura K, Koike N, Buhr ED, Furukawa T, Ko CH, Gloston G, Ayoub C, Nohara K, et al. 2017. Period2 3'-UTR and microRNA-24 regulate circadian rhythms by repressing PERIOD2 protein accumulation. Proc Natl Acad Sci U S A. 114(42):E8855–E8864.
- You YH, Qin ZQ, Zhang HL, Yuan ZH, Yu X. 2019. MicroRNA-153 promotes brain-derived neurotrophic factor and hippocampal neuron proliferation to alleviate autism symptoms through inhibition of JAK-STAT pathway by LEPR. Biosci Rep. 39(6):BSR20181904.
- Yu D, Jiao X, Cao T, Huang F. 2018. Serum miRNA expression profiling reveals miR-486-3p may play a significant role in the development of autism by targeting ARID1B. Neuroreport. 29(17):1431–1436.
- Yuan P, Yang T, Mu J, Zhao J, Yang Y, Yan Z, Hou Y, Chen C, Xing J, Zhang H. et al. 2020. Circadian clock gene NPAS2 promotes reprogramming of glucose metabolism in hepatocellular carcinoma cells. Cancer Lett. 469:498–509.
- Zadehbagheri F, Hosseini E, Bagheri-Hosseinabadi Z, Rekabdarkolaee HM, Sadeghi I. 2019. Profiling of miRNAs in serum of children with attention-deficit hyperactivity disorder shows significant alterations. J Psychiatr Res. 109:185–192.
- Zhang J, Fang Z, Jud C, Vansteensel MJ, Kaasik K, Lee CC, Albrecht U, Tamanini F, Meijer JH, Oostra BA, et al. 2008. Fragile X-related proteins regulate mammalian circadian behavioral rhythms. Am J Hum Genet. 83(1):43–52.
- Zhang PJ, Zheng DX, Sun DY, Pan DY. 2019. High throughput sequencing reveals circadian rhythm gene rorα is cooperatively suppressed by multiple micrornas in oral squamous cell carcinoma. Oral Surg Oral Med Oral Pathol Oral Radiol. 128(1):e26.
- Zhang W, Wang P, Chen S, Zhang Z, Liang T, Liu C. 2016. Rhythmic expression of miR-27b-3p targets the clock gene Bmal1 at the posttranscriptional level in the mouse liver. FASEB J. 30(6):2151–2160.
- Zhao X, Zhu X, Cheng S, Xie Y, Wang Z, Liu Y, Jiang Z, Xiao J, Guo H, Wang Y. 2014. MiR-29a/b/c regulate human circadian gene hPER1 expression by targeting its 3'UTR. Acta Biochim Biophys Sin (Shanghai). 46(4):313–317.
- Zheng X, Wu K, Liao S, Pan Y, Sun Y, Chen X, Zhang Y, Xia S, Hu Y, Zhang J. 2018. MicroRNA-transcription factor network analysis reveals miRNAs cooperatively suppress RORA in oral squamous cell carcinoma. Oncogenesis. 7(10):1–18.
- Zheng Y, Wang HQ, Guo HX, Xie HL, Zhang WD, Han DX, Jiang H, Yuan B, Zhang JB. 2022. CircRNA-WNK2 acts as a ceRNA for miR-328a-3p to promote AANAT expression in the male Rat pineal gland. Endocrinology. 163(2):bqab255.
- Zhou J, Park CY, Theesfeld CL, Wong AK, Yuan Y, Scheckel C, Fak JJ, Funk J, Yao K, Tajima Y, et al. 2019. Whole-genome deep-learning analysis identifies contribution of noncoding mutations to autism risk. Nat Genet. 51(6):973–980. eng.
- Zhou L, Miller C, Miraglia LJ, Romero A, Mure LS, Panda S, Kay SA. 2021. A genome-wide microRNA screen identifies the microRNA-183/96/182 cluster as a modulator of circadian rhythms. Proc Natl Acad Sci USA. 118(1):e2020454118.