ABSTRACT
Probiotics offer various health benefits. Lactobacillus plantarum has been used for decades to enhance human intestinal mucosal immunity and improve skin barrier integrity. Extracellular vesicles (EVs) derived from eukaryotic or prokaryotic cells have been recognized as efficient carriers for delivery of biomolecules to recipient cells, and to efficiently regulate human pathophysiology. However, the mechanism underlying the beneficial effects of probiotic bacteria-derived EVs on human skin is unclear. Herein, we investigated how L. plantarum-derived EVs (LEVs) exert beneficial effects on human skin by examining the effect of LEVs on cutaneous immunity, particularly on macrophage polarization. LEVs promoted differentiation of human monocytic THP1 cells towards an anti-inflammatory M2 phenotype, especially M2b, by inducing biased expression of cell-surface markers and cytokines associated with M2 macrophages. Pre- or post-treatment with LEVs under inflammatory M1 macrophage-favouring conditions, induced by LPS and interferon-γ, inhibited M1-associated surface marker, HLA-DRα expression. Moreover, LEV treatment significantly induced expression of macrophage-characteristic cytokines, IL-1β, GM-CSF and the representative anti-inflammatory cytokine, IL-10, in human skin organ cultures. Hence, LEVs can trigger M2 macrophage polarization in vitro, and induce an anti-inflammatory phenomenon in the human skin, and may be a potent anti-inflammatory strategy to alleviate hyperinflammatory skin conditions.
Introduction
Like mammalian cells, most bacteria constitutively release lipid bilayer-enclosed, nanosized extracellular vesicles (EVs) termed outer membrane vesicles in gram-negative bacteria, or membrane vesicles in gram-positive bacteria [Citation1–Citation3]. These EVs contain bioactive molecules, including nucleic acids, proteins, lipids, toxins and various virulence factors, and have diverse functions in cell-to-cell communication between bacteria and in inter-kingdom signalling. Bacterial EVs are more effective than bacterial extracts or purified toxins in evoking cellular responses in the recipient cells [Citation1,Citation4,Citation5]. Moreover, pathogenic bacterial EVs can directly and potently affect the human immune system and cause disease even in the absence of live cells, as exemplified in sepsis [Citation6–Citation9]. Recently, gram-positive bacteria-derived EVs have been drawing increasing attention due to their effects on human health [Citation10]. In particular, EVs derived from Staphylococcus aureus and Propionibacterium acnes, representative gram-positive bacteria on the human skin, are strongly associated with the pathogenesis of atopic dermatitis and acne vulgaris, respectively [Citation4,Citation11].
In contrast to pathogen-derived EVs, probiotic bacteria-derived EVs have been suggested to exert beneficial immunomodulatory properties, as indicated by findings in disease models of inflammatory colitis and in intestinal immune cells [Citation12]. Probiotics were originally defined as live microorganisms that exhibit beneficial effects on gut function upon administration; therefore, studies of probiotic bacteria, represented primarily by Lactobacillus and Bifidobacterium, and their EVs have mostly focused on their effects on the intestinal immune system or intestinal epithelial barrier function [Citation10,Citation13–Citation16]. Given that the skin is another major habitat for microorganisms and is exposed daily to various environmental cues affecting the composition of commensal and pathogenic bacteria, it is important to investigate the effects of probiotics and their EVs on the skin to provide a means to beneficially regulate the skin microflora. A recent report suggested that Lactobacillus plantarum-derived EVs (LEVs) can prevent skin inflammation in atopic dermatitis [Citation17]; however, the underlying mechanism was not elucidated.
Macrophages, a component of the mononuclear phagocyte system, are part of the innate immune system and play major roles not only in inflammation and host defence, but also in protective functions, including anti-inflammation, wound healing and tissue remodelling [Citation18,Citation19]. Owing to their diversity and plasticity, mature but inactive macrophages (often referred to as naïve M0), which are directly differentiated from monocytes that have migrated into tissues, can be subsequently polarized towards two distinct active states depending on microenvironmental signals. Classically activated (M1) macrophages are induced by toll-like receptor (TLR) and interferon (IFN) signals (lipopolysaccharides [LPS], IFN-γ), while alternatively activated (M2) macrophages are further divided into four subtypes in response to various activating factors: M2a, which are induced by IL-4 and IL-13, M2b induced by immune complexes (antigen–antibody) in combination with IL-1β or LPS, M2 c induced by IL-10, transforming growth factor (TGF)-β or glucocorticoids, and M2d/TAM induced by costimulation with TLR ligands and A2 adenosine receptor agonists, or by IL-6 [Citation19–Citation24]. While M1 macrophages predominate during the early stage of inflammation and mediate pathogenic clearance and the recruitment of other effector cells, M2 macrophages are involved in anti-inflammation, wound healing, tissue remodelling and vasculogenesis at the end of inflammation. Failure to switch from M1 to M2 predominance, or an imbalance in their ratio, causes chronic inflammation and tissue damage and is associated with various human diseases, including allergic asthma, atherosclerosis, obesity, type 2 diabetes, autoimmune diseases and chronic skin diseases [Citation21,Citation25,Citation26], implying that a balanced M1/M2 ratio is important for tissue homoeostasis. Among the various factors regulating M1 or M2 polarization, such as cytokines, growth factors, drugs and natural products, (mesenchymal) stem cell- or pathogen-derived EVs preferentially induce polarization towards M2 or M1, respectively [Citation27,Citation28], suggesting that EVs play a role in macrophage polarization.
In this study, therefore, we investigated the role of LEVs from the probiotic strain L. plantarum APsulloc 331261 in regulating cutaneous immunity, especially macrophage polarization. To this end, human monocytic THP1 cells and human skin organ cultures were treated with LEVs, after which the expressions of various cellular markers and cytokines associated with macrophage states were analysed and the released cytokines were determined. We further validated the effect of LEV treatment before or after M1 macrophage induction on the expression of M1-specific polarization markers.
Materials and methods
Purification of bacterial EVs
For separation/isolation, characterization and functional studies of bacterial EVs, we followed the guidelines of minimal information for studies of EVs 2018 (MISEV2018) [Citation29]. L. plantarum APsulloc 331261 (deposit number: KCCM11179P) was isolated from green tea leaves collected in the Dosun Green Tea Garden in Jeju, South Korea by Amorepacific R&D Center (Yongin, South Korea). L. plantarum APsulloc was grown in MRS broth at 37°C in an anaerobic chamber (Bactron; Sheldon Manufacturing, Cornelius, OR) connected to a gas cylinder containing 5% H2, 5% CO2 and 90% N2 for 24 h, and was then subcultured in the same condition following 1 to 100 dilution. S. aureus (ATCC 6538) was grown in BDTM Tryptic Soy Broth medium (Franklin Lakes, NJ) for 18–24 h at 32°C and then subcultured in the same condition after 1 to 100 dilution. When the culture reached an optical density of 1.0–1.5 at 600 nm, or reached stationary phase, the cells (≥ 2.7 × 109 colony-forming units (cfu)/mL of L. plantarum APsulloc; ≥ 8.6 × 108 cfu/mL of S. aureus) were pelleted by sequential centrifugation at 1800× g at 4°C for 20 min and 10,000× g at 4°C for 20 min. The culture supernatants (≤10 L) of L. plantarum APsulloc were filtered through a 0.45-μm membrane (Merck Millipore, Burlington, MA) and concentrated 10-fold using a tangential flow filtration (TFF) system with Pellicon 2 mini filters (Merck Millipore). LEVs or S. aureus-derived extracellular vesicles (SEVs) were purified from 10-fold culture concentrates or approximately 1 L-culture supernatants, respectively, according to a previously reported purification method for gram-positive bacterial EVs [Citation30]. In brief, the supernatants were filtered through a 0.45-μm filter membrane and subjected to ultracentrifugation (UC) at 150,000× g at 4°C for 3 h (Type 45 Ti rotor, Beckman Coulter, Brea, CA). The pellet was diluted in HEPES-buffered saline (HBS) and the total protein concentration was determined using a Bradford assay (Bio-Rad Laboratories, Hercules, CA). The distribution, average diameter and particle numbers of EVs were measured using qNano Gold equipped NP80, NP150 or NP200 nanopores based on the principle of tunable resistive pulse sensing (TRPS) (Izon Science, Christchurch, New Zealand), or using Zetasizer Nano ZS based on dynamic light scattering (DLS) (Malvern Instruments, Worcestershire, UK). LEVs or SEVs purified by an UC method were investigated for their biological effects on human THP1 cells (10 μg/mL protein concentration) and skin organ cultures (50 μg/mL protein concentration). Medium concentrates from complete MRS medium that had not been conditioned by bacteria, yet was processed in the same way as conditioned medium, were prepared using the same procedure for LEV purification by UC and used as negative control for the LEV effect. To prepare L. plantarum ferment lysates (LFLs), cells and culture supernatants were ruptured under high pressure (>1000 bars). The fractions containing nanosized particles were then enriched by a continuous process of filtration through a 0.22 μm membrane (Merck Millipore) and ultrafiltration using a membrane module rated at a 10 kDa-nominal molecular weight limit (Merck Millipore).
OptiPrep density gradient UC
Density gradient UC using OptiPrepTM [60% (w/v) solution of iodixanol in water; Axis-Shield PoC AS, Oslo, Norway] was performed according to the manufacturer’s protocol with specific modifications. A discontinuous gradient of 50%, 35% and 20% (w/v) iodixanol was made by mixing 50% (w/v) iodixanol working solution with appropriate amounts of a homogenization medium (HM; 0.25 M sucrose, 150 mM NaCl, 20 mM HEPES pH 7.4). Iodixanol working solution was prepared by mixing 5 volumes of OptiPrep with 1 volume of dilution medium (DM; 0.25 M Sucrose, 0.9 M NaCl, 120 mM HEPES pH 7.4). The LEV pellets after UC were dissolved in 0.417 mL of DM and mixed with 5 volumes (2.085 mL) of OptiPrep solution. Next, 2.5 mL of control (50% iodixanol alone), or sample in 50% iodixanol was overlaid with 2.5 mL each of 35%, 20% and 0% solutions and centrifuged at 200,000× g at 4°C for 2 h (SW 41 Ti rotor, Beckman Coulter). The 1 mL fractions were then collected from the top of the gradient in the control tube, and the density of each fraction was determined via a standard curve created with absorbance values at 340 nm. The EV fractions at densities between 1.17~1.24 (between fractions 5 and 6; F5-6) were collected using a 1 mL-syringe from the sample tube. To remove iodixanol, the fraction was diluted in 60 mL of HBS, ultracentrifuged at 150,000× g at 4°C for 3 h, and resuspended with 200 μL of HBS. LEVs purified by OptiPrep density gradient UC (density-purified LEVs) were analysed for total protein concentration using a Bradford assay, and their distribution, average diameter and particle numbers were measured using DLS with Zetasizer Nano ZS or TRPS with qNano Gold. Density-purified LEVs were then used for validating the biological effects of UC-purified LEVs and for high resolution image analysis using bio-transmission electron microscopy (TEM) and cryo-electron microscopy (EM).
Bio-TEM and cryo-EM analyses
Bio-TEM images were obtained as previously described [Citation4]. In brief, density-purified LEVs were applied to 400-mesh copper grids and negatively stained with 2% uranyl acetate. Electron micrographs were recorded with a Tecnai G2 20 microscope (FEI, Hillsboro, OR) at an acceleration voltage of 200 kV. For cryo-EM analysis, 3 µL of LEVs was added to both sides of a Quantifoil TEM grid with a hole diameter of 1.2 μm and an inter-hole distance of 1.3 μm. The TEM grid was blotted for 1.5 s and plunged into liquid ethane using a Cryoplunge 2 system (Gatan, Pleasanton, CA). The cryo-TEM samples were stored in liquid nitrogen before TEM observation. The samples were examined under a Tecnai F20 electron microscope (FEI) operated at 120 kV.
Human skin organ culture
Written informed consent was obtained, and the study protocol was approved by the Institutional Review Board of the Severance Hospital at Yonsei University College of Medicine in Seoul, Republic of Korea (IRB no. 7-2017-1041). Postsurgical remnants of human abdominal skin from two different donors were obtained and prepared as previously described [Citation31]. In each experiment, the tissue sample was divided into 1 cm × 1 cm pieces as needed for material treatments including a vehicle for a negative control, and cultured in Dulbecco’s modified Eagle’s medium supplemented with 2% foetal bovine serum and 1% penicillin/streptomycin at 37°C in the presence of 5% CO2 for six days. On days 2 and 4, vehicle or bacterial EVs were added in 2-mL culture medium of the tissue samples to a final protein concentration of 50 μg/mL. For cytokine array analysis, culture supernatants were harvested at day 6 and centrifuged at 13,000 rpm at 4°C for 10 min. The supernatants were then applied to the membranes of human cytokine antibody array C3 (RayBiotech, Peachtree Corners, GA) and immune blotting was performed following the manufacture’s protocol. Dot intensity for each cytokine on the membrane was determined by densitometry using ImageJ (https://imagej.nih.gov/ij/). The tissues at day 6 were embedded in Tissue-Tek® O.C.T. compound (Sakura Finetek USA, Torrance, CA) and frozen on dryice. Frozen tissue sections were stained with haematoxylin and eosin (Abcam, Cambridge, UK).
Cell culture
Human THP1 cells were maintained in RPMI-1640 medium supplemented with 10% FBS, 1 unit of penicillin and 50 μg/mL of streptomycin (Thermo Fisher Scientific, Waltham, MA, USA) at 37°C in the presence of 5% CO2. To induce differentiation of monocytes to macrophages, THP1 cells were treated with 10 nM phorbol-12-myristate-13-acetate (PMA; Sigma-Aldrich) or 10 μg/mL LEVs for 48 h. To induce M1 macrophages, THP1 cells were treated with 20 ng/mL of IFN-γ and 10 pg/mL of LPS (R&D Systems, Minneapolis, MN, USA). Cytotoxicity was measured using the Pierce™ LDH Cytotoxicity Assay Kit according to the manufacturer’s instruction (Thermo Fisher Scientific).
Quantitative reverse transcription (RT-q)PCR
Total RNA was extracted from cells using TRIzol® reagent (Thermo Fisher Scientific), following the manufacturer’s instructions, and was quantified using a NanoDrop One spectrophotometer (Thermo Fisher Scientific). RNA (100 ng) was reverse-transcribed using the iScript™ cDNA synthesis kit (Bio-Rad, Hercules, CA, USA), following the manufacturer’s instructions. qPCR was performed using a QuantStudio 3 real-time PCR system (Thermo Fisher Scientific) using TaqMan™ Gene Expression Master Mix (Thermo Fisher Scientific) per the manufacturer’s instructions. Thermal cycles were as follows: 5 min at 95°C; followed by 40 cycles of 15 s at 95°C and 60 s at 60°C. Primers and hydrolysis probe sets (TaqMan Gene Expression Assay) for CD14, CCR2, ICAM-1 and all TLRs 1–9 were purchased from Thermo Fisher Scientific. For other genes, gene-specific primers were designed using ProbeFinder software, and a matching probe was obtained from Universal ProbeLibrary (Roche, Basel, Switzerland; Supplementary Table S1). Gene expression was determined using the 2–ΔΔCq method, and all data were normalized to glucose-6-phosphate dehydrogenase (G6PD) as a reference gene. Along with G6PD, additional reference genes, including β-actin (ACTB), TATA-box binding protein (TBP), ribosomal protein L32 (RPL32) and glyceraldehyde 3-phosphate dehydrogenase (GAPDH) were used to validate their consistent expression during culture under different cellular conditions and to verify the qPCR data normalized by G6PD following MIQE guidelines [Citation32].
Droplet digital (dd)PCR
Total RNA (100 ng) was reverse-transcribed using the iScript™ cDNA synthesis kit with MS2 RNA spike-in (Roche, Indianapolis, IN). The cDNA (diluted 1:10) was subjected to ddPCR on a QX200 Droplet Digital PCR System (Bio-Rad) using ddPCR Supermix for Probes (Bio-Rad), following the manufacturer’s instructions. Data acquisition and analysis were performed using the in-built software (Bio-Rad) [Citation4]. Primers and probes for TLRs were purchased from Thermo Fisher Scientific.
Statistical analysis
The preparation of LEVs using UC or density gradient UC methods were performed a minimum of three times. The aliquots of LEVs at high protein concentration purified from 10 L-culture by UC method were used to investigate biological effects. All experiments for RT-qPCR analyses in THP-1 cells were conducted a minimum of three times (three independent treatments). The experiments involving human skin cultures were performed twice using two independent tissue samples. Data are expressed as the mean ± standard error of the mean (SEM). Statistical significance was determined by one-way ANOVA for three or more groups or Student’s t-test for two groups. p < 0.05 was considered significant.
Results
L. plantarum APsulloc spontaneously releases EVs
We previously identified L. plantarum APsulloc 331261 (Accession No. given by the international depositary authority: KCCM11179P; hereafter, referred to as L. plantarum) from fermented green tea leaves. LEVs were isolated from culture supernatants using conventional UC, or density UC methods according to the purpose (biological effect versus high resolution image, respectively) (Supplementary Figure S1a). The size and distribution of LEVs purified by both methods was similar based on DLS analysis (Supplementary Figure S1b). TRPS analysis revealed that UC- and density-purified LEVs had a diameter of approximately 100 nm, with an average value of 104 ± 42.4 nm (mode diameter, 77 nm) and 83 ± 20.3 nm (mode diameter, 73 nm), respectively, implying a wide size distribution in both purification methods ()). The mean number of particles per milligram of protein was 2.13 × 109 for UC-purified LEVs, and 3.83 × 1010 for density-purified LEVs ()), suggesting that EVs purified by a density gradient UC show higher purity than those purified via conventional UC methods. Given the total culture volume and number of cells, the yield of UC-purified LEVs was approximately 2.45 × 1010 per 1 L or 2.7 × 1012 cfu/L. According to bio-TEM and cryo-EM analyses for density-purified LEVs, LEVs had a closed spherical membrane structure ()), similar to previously described gram-positive bacteria-derived EVs [Citation4,Citation30]. Meanwhile, UC-purified LEVs (hereafter, referred to as LEVs) showed less cytotoxicity than whole cell lysates of L. plantarum when used at high protein concentrations of 10 μg/mL (Supplementary Figure S2), at which LEVs were used in subsequent in vitro assays.
Figure 1. Purification of EVs from L. plantarum APsulloc. (a) TRPS analysis of ultracentrifugation (UC)- or OptiPrep density UC (Density)-purified LEVs. The purification of LEVs using two UC methods was performed a minimum of three times and representative TRPS results are shown for each method. (b) Protein concentration and particle numbers of purified LEVs. Conc., concentration; No., number. (c) Bio-TEM and cryo-EM image analyses of density-purified LEVs. The outlined LEV images are enlarged and the lipid bilayer indicated by black and white arrows. Black and white arrowheads indicate the potential double membrane of the vesicles. Scale bars, 200 nm.
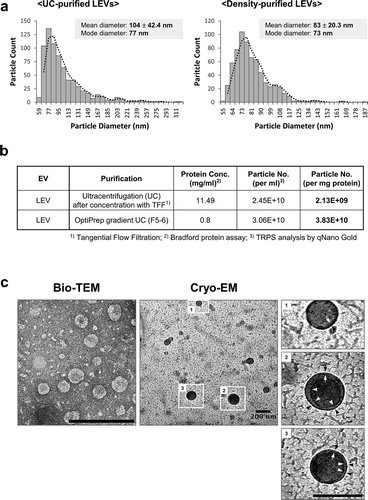
LEV treatment enhances the secretion of anti-inflammatory cytokine IL-10 in human skin organ cultures
Given that probiotic bacteria, including Lactobacillus, modulate host immune responses, we investigated whether LEVs could influence immune reactions in a human skin organ culture system. LEVs or SEVs, which are strongly pathogenic EVs associated with atopic dermatitis [Citation11,Citation33,Citation34], were applied twice to human skin specimens during a 6-day culture period, and the tissues and culture supernatants were harvested for immunohistological and cytokine array analyses, respectively (); Supplementary Figure S3). Skin samples in the SEV-treated group showed an aberrant epidermal structure, with multiple vacuoles, which was quantified by measuring the ratio of the number of vacuoles (empty space due to cytoplasmic loss) to the total number of cells in the epidermis, whereas those in the LEV-treated group did not show any damage when compared to vehicle-treated control skin samples (); Supplementary Figure S3a). These findings suggest that the cultured skin tissues properly and differentially respond to external cues. Moreover, SEV treatment caused a relatively high increase in the secretion of multiple cytokines, including GM-CSF, IL-13, IL-1β, IL-10, CXCL9, chemokine C-C motif ligand (CCL)8, IL-2 and IL-1α (in descending order), by more than 2-fold differences when compared to vehicle treatment ()). In contrast, LEV treatment did not increase, but rather suppressed the secretion of most inflammatory cytokines when compared to vehicle or SEV treatment, however, it did significantly increase the secretion of anti-inflammatory IL-10 or that of IL-1β and granulocyte-macrophage colony-stimulating factor (GM-CSF) (); Supplementary Figure S3b). Taken together, these results suggest that without causing tissue damage, LEV treatment suppresses the secretion of major inflammatory cytokines and increases at least the secretion of anti-inflammatory cytokine IL-10 in cultured human skin tissues.
Figure 2. LEV treatment enhances the secretion of anti-inflammatory cytokine IL-10 in human skin organ cultures. Human cultured skin tissues were treated twice with vehicle (HBS), LEVs (50 μg/mL), or SEV (50 μg/mL) during a 6-day culture period. Tissues and supernatants were harvested on day six for H&E staining and cytokine array analysis (n = 2; two independent tissue samples). (a) Scheme of bacterial EV treatment of human skin cultures and H&E staining. The outlined area is enlarged. Scale bars, 100 μm. (b) Abnormality of the epidermis was quantified by measuring the ratio of the number of vacuoles (empty space due to cytoplasmic loss) to the total number of cells in the epidermis. Data are expressed as the mean ratio ± SEM of three different images and statistical significance was analysed by one-way ANOVA. ****p < 0.0001; ns, non-significant. (c) Representative immunoblot images of cytokine array analysis. Markedly increased cytokines by LEV and SEV treatments are indicated by red-coloured boxes and arrows. Inflammatory cytokines that are preferentially, and significantly, increased by SEV treatment are indicated by blue-coloured boxes and arrows. Cytokines that are more than 2-fold increased or decreased in LEV- or SEV-treated compared to vehicle-treated groups are shown left or right, respectively, on the basis of NC/PC in the graph (red line for 2-fold increase; green line for 2-fold decrease). The average fold change in LEV- or SEV- treated groups relative to vehicle control was determined by densitometry using ImageJ (https://imagej.nih.gov/ij/). NC/PC, negative control/positive control of the spots in cytokine array.
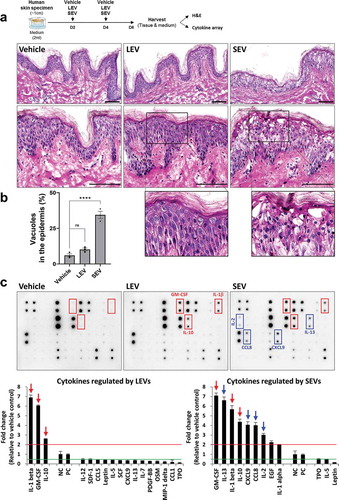
LEV treatment induces the differentiation of human monocytic THP1 cells towards the macrophage lineage
Considering that LEVs induced cytokines including IL-1β, GM-CSF and IL-10, which are primarily secreted by macrophages [Citation35,Citation36], in cultured human skin tissues (); Supplementary Figure S3b), we postulated that they may influence monocyte differentiation and macrophage activation. The mature yet inactive state of macrophages (naïve M0) are differentiated from monocytes that have migrated into tissues and are subsequently polarized into pro-inflammatory M1, or anti-inflammatory and tissue-repairing M2 macrophages, all of which secrete IL-10 [Citation21,Citation35,Citation37]. Once monocytes become differentiated into the macrophage lineage, they reportedly express several markers including cluster of differentiation 14 (CD14), intercellular adhesion molecule 1 (ICAM-1) and C-C chemokine receptor type 2 (CCR2) in different patterns. CD14, as a co-receptor of TLR4, detects bacterial LPS and is highly expressed in monocytes and most tissue macrophages [Citation38]. ICAM1, one of the specific markers of activated macrophages, participates in the binding of immune cells to endothelial cells for extravasation [Citation39,Citation40]. Meanwhile, CCR2, a receptor for CCL2 (monocyte chemoattractant protein-1), has a unique role in monocyte chemotaxis and is lost upon monocyte differentiation [Citation41]. Thus, as a first step, we investigated whether LEV treatment can induce the differentiation of human THP1 monocytic cells towards the macrophage lineage by examining the expression patterns of these genes.
In general, monocytes differentiate into the macrophage lineage upon exposure to PMA [Citation42,Citation43]. When THP1 cells in suspension culture were treated with PMA for 48 h, they significantly adhered to the surface ()), indicating that they had transitioned to activated macrophages [Citation42,Citation43]. Moreover, in PMA-treated cells, gene expression of CD14 and ICAM-1 was significantly increased, whereas that of CCR2 was decreased ()). Thus, THP1 cells properly responded to PMA by differentiating into macrophages. In the same way, LEV treatment induced the adherence of THP1 cells to the surface ()), and significantly upregulated the expression of CD14 and ICAM-1 and downregulated that of CCR2 compared to treatments with the vehicle or medium concentrates, to a greater extent than that observed upon PMA treatment ()). Furthermore, density-purified LEVs showed much stronger activity in regulating the expression of these genes ()), confirming the biological effects driven by UC-purified LEVs. The expression patterns of CD14 in different treatments, which were normalized by G6PD, were further verified using two other reference genes, ACTB and RPL32 (Supplementary Figure S4). These results suggest that LEV treatment induces the differentiation of monocytic cells into the macrophage lineage even in the absence of PMA, possibly controlling the fate of monocytes.
Figure 3. LEV treatment induces the differentiation of human monocytic THP1 cells towards the macrophage lineage. (a) Bright-field microscopic images of THP1 cells in culture. THP1 cells in suspension were treated with vehicle (HBS), PMA (10 nM), medium conc. (medium concentrates, 10 μg/mL), or LEVs (10 μg/mL) for 48 h. Magnification, 100 ×. (b) THP1 cells were treated with medium conc. (10 μg/mL), LEVs (10 μg/mL), PMA (10 nM), or density-purified LEVs (density-LEVs, 10 μg/mL) for 48 h. The mRNA expression of activated macrophage-specific genes was analysed by RT-qPCR. Glucose-6-phosphate dehydrogenase (G6PD) was used for normalization. Data are expressed as the mean fold change ± SEM of triplicate measurements and statistical significance was analysed by one-way ANOVA.
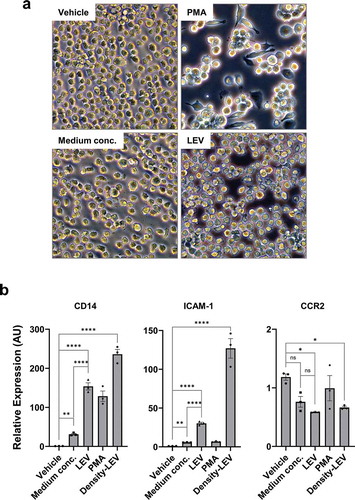
LEV treatment enhances the expression of M2-polarized cell markers
Based on the fact that LEVs can induce monocyte differentiation to the macrophage lineage, which are subsequently polarized to M1 or M2 macrophage states, as a next step, we extensively analysed the mRNA expression of a series of cell markers related to either M1 or M2 macrophages, which have been described elsewhere [Citation18–Citation22,Citation35], using RT-qPCR in response to LEV treatment. The M1 or M2-polarized genes showing significantly increased mRNA levels in LEV-treated compared to vehicle-treated cells were highlighted ()). Among M1-polarized cell markers, LEV treatment upregulated the expression of IL-15, suppressor of cytokine signalling 3 (SOCS3), and CD80 [Citation21,Citation44,Citation45]. However, it had no significant effect on the expression of other M1 macrophage markers, such as CD68 [Citation21], TLR2/TLR4 [Citation46], which are highly expressed in THP1 cells (Supplementary Figure S5), and human leukocyte antigen-DR alpha (HLA-DRα) [Citation18,Citation21] (). In contrast, the majority of M2-related genes, including CD209 [Citation47], C-type lectin domain family 5-member A (CLEC5A) [Citation48], CD200 R [Citation49], sphingosine kinase 1 (SPHK1), class A scavenger receptor 1 (SRA1) [Citation50], IL1 receptor, type II (IL1R2) [Citation49], and CD163 [Citation51], were significantly increased in LEV-treated, compared to vehicle-treated, cells (). The functional activity of LEVs in regulating the expression of macrophage-polarized genes was further verified using density-purified LEVs, which did not significantly increase the expression of M1-related genes (CD68, TLR2, HLA-DRα), however did increase M2-related genes (CLEC5A, SRA, CD163), and was specific when considering the effects driven by medium concentrates (Supplementary Figure S6). Moreover, among M2-related cell markers, not all of which were increased by M2-polarizing factors (IL-4/IL-13), the gene expression patterns of SPHK1 and CD163 after LEV treatment were similar to those induced by IL-4/IL-13 treatment (Supplementary Figure S7a,c). Taken together, although LEV treatment elicited pleiotropic responses in THP1 cells, the overall mRNA expression pattern of macrophage markers in LEV-treated THP1 cells showed a significant bias towards M2 polarization.
Figure 4. LEV treatment enhances the expression of M2-polarized cell markers. THP1 cells were treated with LEVs (10 μg/mL) for 48 h. (a) mRNA expression of markers specific for M1 or M2 macrophages was analysed by RT-qPCR. G6PD was used for normalization. Statistically significant fold changes (FC) are indicated in different colours according to the increased degree (light grey, 1.5 < FC < 5; medium grey, 5 < FC < 10; dark grey, 10 < FC). -, non-significant. (b) mRNA expression of representative M1 macrophage-specific surface markers. ns, non-significant. (c) mRNA expression of M2 macrophage-specific markers. Data in (a-c) are expressed as the mean fold change ± SEM of triplicate treatments and statistical significance was analysed by Student’s t-tests.
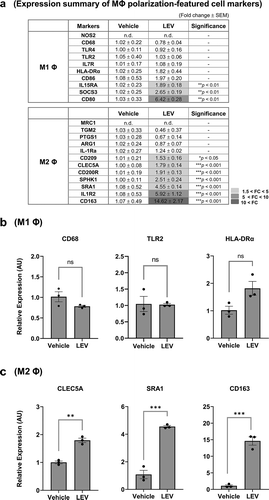
Gene expression profiling of secretory proteins after LEV treatment reveals a preferential differentiation of THP1 cells to M2b macrophages
We further examined the mRNA expression profile of chemokines after LEV treatment in the presence of PMA to activate THP1 cells. Chemokines associated with different subtypes of macrophages have been described elsewhere [Citation21,Citation35,Citation52]. The mRNA expression of chemokine genes that are supposedly stimulated by M1 macrophages was not significantly different between LEV-treated and vehicle-treated groups, although some chemokines were slightly upregulated by LEV treatment (). Among the chemokines related to three subtypes of M2 macrophages, only M2b macrophage-related chemokines were significantly increased by LEV treatment (), meanwhile, CCL23 and CCL24, which are characteristic of M2a macrophages, were significantly downregulated ()). The expression of CXCL13 and CCL18, which are specific for M2c macrophages [Citation52], was hardly affected ()). Of M2b-related chemokines increased by LEV treatment, the expression of CXCL1 and CCL20, and not CCL1, CXCL2 or CXCL3, were increased in M2-inducing conditions by IL-4/IL-13 treatment (Supplementary Figure S7b,c), suggesting that chemokine expression after LEV treatment is at least in part similar to that in M2-inducing conditions although different stimulating factors are required to induce specific subtypes of M2 macrophage. Among various cytokines, IL-10, IL-1β, and IL-6, which are M2 macrophage-characteristic cytokines [Citation24,Citation53–Citation55], were barely expressed in PMA-treated THP1 cells; however, their expression was significantly upregulated upon LEV treatment ()). This result was in line with the fact that in human skin organ cultures, the protein levels of IL-1β and IL-10 were increased by more than 2-fold upon LEV treatment ()). Taken together, these findings indicate that LEV treatment upregulates the expression of secretory proteins specific for M2 macrophages, especially, M2b macrophages, implying that LEVs can regulate macrophage polarization during monocyte/macrophage transition towards the anti-inflammatory or tissue-repairing alternative M2 state in the skin.
Figure 5. Gene expression profiling of secretory proteins after LEV treatment reveals a preferential differentiation of THP1 cells to M2b macrophages. THP1 cells were pre-incubated with PMA (10 nM) for 48 h and treated with vehicle (HBS) or LEVs (10 μg/mL) for an additional 48 h. (a) mRNA expression of M1- and M2-specific chemokines was analysed by RT-qPCR. G6PD was used for normalization. Statistically significant fold changes (FC) are indicated in different colours according to the increased degree (light grey, 1.5 < FC < 5; medium grey, 5 < FC < 10). -, non-significant. (b, c) mRNA expression of representative M1 (b) and M2b (c) macrophage-specific chemokines. ns, non-significant. (d) mRNA expression of M2 macrophage-specific cytokines as determined by RT-qPCR. Data in (a-d) are expressed as the mean fold change ± SEM of triplicate treatments and statistical significance was analysed by Student’s t-tests.
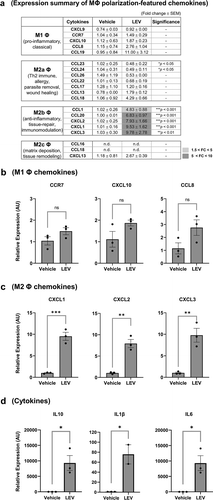
LEV treatment downregulates inflammation-induced expression of M1 macrophage cell-surface markers
Next, we investigated whether LEVs could alleviate inflammation-related phenomena. IFN-γ and LPS are used to evoke inflammatory responses and to drive monocytes into M1 macrophages [Citation43]. Thus, THP1 cells were treated with LEVs and/or IFN-γ and LPS in the presence or absence of PMA to examine whether LEVs interfere with M1 induction. The expression of HLA-DRA, which is upregulated in M1 macrophages upon exposure to pro-inflammatory stimuli [Citation21,Citation56], was not significantly changed by LEVs per se (), but was markedly upregulated under M1-inducing conditions ()). However, this upregulation was significantly inhibited by LEV treatment before or after M1 induction by IFN-γ and LPS. The inhibitory effect of LEVs under M1-inducing conditions was also observed in PMA-free conditions ()). These results suggest that LEVs could reduce the inflammation-related phenomena associated with M1 macrophages, possibly by promoting the differentiation of naïve macrophages towards the M2 state or by increasing the transition of M1 to M2 macrophages.
Figure 6. LEV treatment downregulates inflammation-induced expression of the M1 macrophage cell-surface marker, HLA-DRα. Human THP1 monocytes at day 1 post plating were pre-incubated with 10 nM PMA. PMA was maintained during the treatment period (A) or washed out after 48 h (b). On days 3 and 5, the cells were treated with LEVs (10 μg/ml) or 20 ng/ml of IFN-γ and 10 pg/ml of LPS for 48 h. On day 7, the cells were harvested and HLA-DRα mRNA expression was analysed by RT-qPCR. G6PD was used for normalization. Data are expressed as the mean fold change ± SEM of triplicate measurements and statistical significance was analysed by one-way ANOVA.
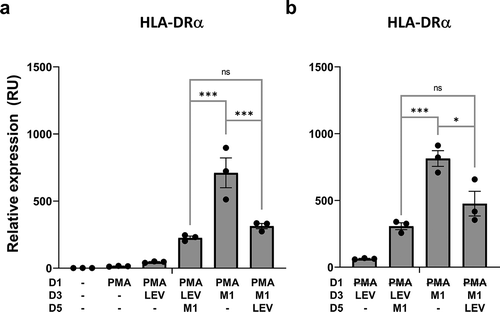
Bacterial fractions prepared under high pressure contain LEV-like nanovesicles and show similar effects as LEVs in human skin organ cultures
When EVs are considered as powerful bioactive materials to promote changes in their targets, one significant limitation is the low yield and complicated purification procedure of EVs. To overcome this in mammalian cells, EV-mimetic nanovesicles have been produced from stem cells or immune cells via multiple serial extrusions [Citation57,Citation58]. Based on a similar concept, we manufactured LEV-like vesicles using high pressure and successive filtration and ultrafiltration procedures to efficiently collect the nanosized materials. According to bio-TEM and TRPS analyses, bacterial fractions after this process (termed Lactobacillus ferment lysates, LFLs) included nanosized vesicles with an average diameter of 173 ± 59.6 nm, yielding approximately 1.97 × 108 particles per mg proteins ()). In human skin organ cultures, LFL-treated tissues showed no damage to the epidermis at the same protein concentration as LEVs and had increased expression of IL-1β, GM-CSF, and IL-10, similar to LEV-treated tissues ()). Although LFLs were less effective than LEVs in down-regulating inflammatory cytokines, save for PDGF-BB, TPO and leptin, which were all shown to be decreased by 0.5-fold or less in LEV-treated tissues ()), their increase in LFL-treated tissues was less than 2-fold compared to vehicle-treated tissues, and thus, appeared to be of no significance (). These results suggest that bacteria can be processed using high pressure and successive filtration and ultrafiltration procedures to produce bioactive materials comprising EV-like, small-sized vesicles that can be used as an alternative for the treatment of human skin, without inducing damage.
Figure 7. Bacterial fractions prepared under high pressure contain LEV-like nanovesicles and show similar effects as LEVs in human skin organ cultures. (a) Bio-TEM image of LFLs (left) and TRPS analysis for mean diameter and particle number of LFLs (right). The outlined LFL images (1–4) are enlarged and additional large vesicles (5, 6) are shown. Scale bars, 200 nm. (b) Images of H&E staining of human skin cultures after LFL treatment. The cultured tissues were treated with vehicle, LEVs, or LFLs (each 50 μg/ml) twice over a 6-day culture period as indicated in Figure 2a. (c) Abnormality of the epidermis was quantified by measuring the ratio of the number of vacuoles (empty space due to cytoplasmic loss) to the total number of cells in the epidermis. Data are expressed as the mean ratio ± SEM of three different images and statistical significance was analysed by one-way ANOVA. Ns, non-significant. (d, e) Cytokine array analysis of culture supernatants and representative blot image. Markedly increased cytokines by LFL treatment are indicated by red-coloured arrows and boxes. Cytokines that were increased by more than 2-fold in LFL-treated compared to vehicle-treated groups or decreased by 0.5-fold or less in LEV-treated but increased in LFL-treated compared to vehicle-treated groups were shown left or right, respectively, on the basis of NC/PC in the graph (red line for 2-fold increase; green line for 2-fold decrease). The average fold change in LFL-treated groups relative to vehicle control was determined by densitometry using ImageJ. NC/PC, negative control/positive control of the spots in cytokine array.
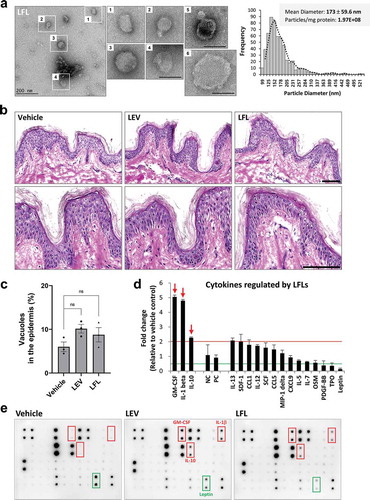
Discussion
Probiotics are administered for their health benefits, primarily for immune modulation. As a representative probiotic species, L. plantarum acts not only on the mucosal immune system, but also on the human skin [Citation59,Citation60]. However, the detailed mechanism underlying its beneficial effects on the skin has not been elucidated. Here, we report for the first time that EVs derived from L. plantarum preferentially induce monocyte differentiation into M2 macrophages in human THP1 cells.
Macrophages play a key role in innate immune surveillance, and proper activation of these cells upon exposure to microenvironmental cues is vital for tissue homoeostasis. In particular, macrophages are pleiotropic and functionally diverse, and are capable of changing their activation state (M1 or M2) in response to growth factors and external cues, such as cytokines, chemokines, microbes and microbial products. In case of failure to switch from the initial inflammatory M1 to the anti-inflammatory and tissue-repairing M2 state, the increased M1 macrophages reinforce a pro-inflammatory environment and generate chronic inflammation associated with the phenotypes of various inflammatory skin disorders such, as atopic dermatitis and psoriasis [Citation26,Citation61–Citation63]. Based on extensive analyses of macrophage-associated cellular marker, chemokine and cytokine gene expression, LEV-treated THP1 cells were found to be primarily differentiated into M2 macrophages, especially, the M2b subtype, which is known to release pro-inflammatory cytokines (e.g., IL-1β, IL-6 and TNF-α) as well as the anti-inflammatory cytokine IL-10 and low levels of IL-12 [Citation24]. Most of these cytokines were observed in LEV-treated human skin organ cultures and in LEV-treated THP1 cells () and ); Supplementary Figure S3b). Given that M2b macrophages exert strong immune-regulatory and anti-inflammatory effects, thereby regulating the breadth and depth of the immune response [Citation24], LEVs may relieve hyperinflammatory conditions by regulating macrophage polarization. Or, LEVs may reduce inflammatory conditions by stimulating M2-associated signalling in M1 macrophages. Considering these capabilities, LEVs could be used to improve hyperinflammatory skin conditions and disorders. Furthermore, LEVs were less cytotoxic than bacterial cell lysates at the same protein concentrations (Supplementary Figure S2), which supports the utilization of LEVs as efficient anti-inflammatory materials across various protein concentrations. However, taking into account the differential cytotoxic effect between LEVs and bacterial cell lysates as well as the limited supply of tissue samples available for the current study, which resulted in the use of only one high dose of LEVs for in vitro (10 μg/mL) and ex vivo (50 μg/mL) treatments, there may be more efficient doses or concentrations of LEVs to sufficiently induce or verify their biological effects. Therefore, it is recommended to test LEV-driven effects at various protein concentrations to validate the effects and to establish the potential for industrial applications. In addition, LFLs prepared by repetitive bacterial cell rupture under high pressure followed by ultrafiltration may be used as LEV-like vesicles that can be produced at a low cost, although these may be less effective at down-regulating inflammatory cytokines compared to LEVs, perhaps due to their larger size, or their potential of containing partially ruptured bacterial cell lysates.
Which bacterial EV factors regulate monocyte differentiation and M2 macrophage polarization remains to be investigated. It has been reported that lipoteichoic acid (LTA) can regulate macrophages via TLRs, among which TLR2 is responsible for immune responses, followed by NF-κB signalling-mediated cytokine production [Citation64,Citation65]. Unlike S. aureus LTA, LTA from L. plantarum has various beneficial effects in humans [Citation66]. Given that EVs, which include LTA or LPS for gram-positive or gram-negative bacteria, respectively, are more effective in evoking cellular responses than bacterial cell extracts and purified toxins [Citation1,Citation4,Citation5], we speculated that LEVs could elicit multifaceted and strong immunomodulatory activities through signalling between vesicular LTA and TLRs, especially TLRs 2 and 4, on THP1 cells (Supplementary Figure S5). However, we do not exclude the possibility that other bioactive molecules, including proteins, lipids, nucleic acids and toxins trapped in LEVs, may also be involved in this regulation. Indeed, the genetic loci in L. plantarum involved in the modulation of the secretion of anti-inflammatory IL-10 and pro-inflammatory IL-12 in human mononuclear cells were identified through an analysis of 42 strains: these loci included genes encoding the phosphotransferase system, quorum-sensing system and bacteriocin biosynthesis and transport [Citation67], implying the involvement of a complex of bacterial factors in the cytokine response in innate immune cells. In this regard, it will be interesting to investigate the protein or lipid components specifically, or preferentially, enriched in L. plantarum APsulloc-derived LEVs and LEV-like vesicles through proteomic or lipidomic analyses and to compare their profiles to those of the parent cells, or EVs derived from other L. plantarum strains or gram-positive bacteria. Moreover, EVs are transported into recipient cells via several mechanisms, including receptor-mediated endocytosis, direct fusion, phagocytosis and caveolae- or clathrin-mediated endocytosis [Citation68]. Hence, to determine which uptake pathways and signalling molecules are involved in LEV-evoked cellular responses would be helpful to interpret bacterial EV-mediated phenomena in human cells and to develop alternative methods using bacterial EVs as immunomodulatory materials.
In human skin organ cultures, pathogenic SEVs increased the levels of multiple inflammatory cytokines, including IL-13, CXCL9, CCL8, IL-2 and IL-1α, as well as the anti-inflammatory cytokine IL-10. Given that IL-13, which is dominant in atopic dermatitis pathological conditions [Citation69], CXCL9, which predominantly mediates lymphocytic infiltration to the inflammatory sites [Citation70], CCL8, which is a chemotactic factor for attracting monocytes and lymphocytes [Citation71], and IL-2, which is primarily secreted by T cells in response to microbial infection [Citation72], are highly associated with inflammatory responses, SEVs are indeed representative pathogenic materials that have a strong impact on skin pathophysiology, as indicated in previous studies where SEVs induced and exacerbated atopic dermatitis‐like skin inflammation in part by recruiting immune cells to activated microvascular endothelial cells [Citation11,Citation33,Citation34]. Nonetheless, the increased IL-10 in SEV-treated skin tissues might be a natural response driven by M2 macrophages or regulatory T (Treg and Tr1) cells to induce immune tolerance under prolonged inflammatory conditions [Citation73], as the culture was maintained for six days ()). In contrast to SEVs, LEVs did not induce these inflammatory cytokines, but rather increased the expression of certain cytokines, including IL-10, IL-1β and GM-CSF. Although IL-1β and GM-CSF have been defined as pro-inflammatory cytokines, they can polarize macrophages into M2-like functional phenotypes or act as an anti-inflammatory/regulatory cytokine, respectively, in the presence of relevant factors [Citation20,Citation36], suggesting the presence of pleiotropic dual activities for these cytokines in dose- and context-dependent manners. Therefore, LEVs can serve as anti-inflammatory and immunomodulatory substances by inducing secretion of these cytokines without inducing inflammatory cytokines.
In summary, our results suggest that without causing tissue damage, LEVs induce secretion of the anti-inflammatory cytokine IL-10, along with immunomodulatory cytokines IL-1β and GM-CSF in human skin organ cultures, and induce monocyte-to-macrophage transition, and macrophage polarization towards the M2b state in vitro. Therefore, LEVs could be used as anti-inflammatory and immunomodulatory substances for correcting the imbalance between M1 and M2 macrophages, thereby improving hyperinflammatory skin conditions and the phenotypes of inflammatory skin disorders.
Disclosure of interest
I-HB, KM, PJP, JK and E-GC are employees of Amorepacific Corporation. The other authors declare that they have no conflicts of interest regarding the contents of this article.
Supplemental Material
Download MS Word (1.5 MB)Supplementary material
Supplemental data for this article can be accessed here.
Additional information
Funding
References
- Brown L, Wolf JM, Prados-Rosales R, et al. Through the wall: extracellular vesicles in Gram-positive bacteria, mycobacteria and fungi. Nat Rev Microbiol. 2015;13(10):620–15.
- Toyofuku M, Nomura N, Eberl L. Types and origins of bacterial membrane vesicles. Nat Rev Microbiol. 2019;17(1):13–24.
- Kim JH, Lee J, Park J, et al. Gram-negative and Gram-positive bacterial extracellular vesicles. Semin Cell Dev Biol. 2015;40:97–104.
- Choi E-J, Lee HG, Bae I-H, et al. Propionibacterium acnes-derived extracellular vesicles promote acne-like phenotypes in human epidermis. J Invest Dermatol. 2018;138(6):1371–1379.
- Rivera J, Cordero RJ, Nakouzi AS, et al. Bacillus anthracis produces membrane-derived vesicles containing biologically active toxins. Proc Natl Acad Sci U S A. 2010;107(44):19002–19007.
- Kulp A, Kuehn MJ. Biological functions and biogenesis of secreted bacterial outer membrane vesicles. Annu Rev Microbiol. 2010;64:163–184.
- Park K-S, Choi K-H, Kim Y-S, et al. Outer membrane vesicles derived from Escherichia coli induce systemic inflammatory response syndrome. PLoS One. 2010;5(6):e11334.
- Raeven P, Zipperle J, Drechsler S. Extracellular Vesicles as Markers and Mediators in Sepsis. Theranostics. 2018;8(12):3348–3365.
- Park KS, Lee J, Lee C, et al. Sepsis-like systemic inflammation induced by nano-sized extracellular vesicles from feces. Front Microbiol. 2018;9:1735.
- Liu Y, Defourny KAY, Smid EJ, et al. Gram-positive bacterial extracellular vesicles and their impact on health and disease. Front Microbiol. 2018;9:1502.
- Kim J, Bin B-H, Choi E-J, et al. Staphylococcus aureus-derived extracellular vesicles induce monocyte recruitment by activating human dermal microvascular endothelial cells in vitro. Clin Exp Allergy. 2019;49(1):68–81.
- Molina-Tijeras JA, Galvez J, Rodriguez-Cabezas ME. The immunomodulatory properties of extracellular vesicles derived from probiotics: a novel approach for the management of gastrointestinal diseases. Nutrients. 2019;11(5):1038.
- Grande R, Celia C, Mincione G, et al. Detection and physicochemical characterization of membrane vesicles (MVs) of lactobacillus reuteri DSM 17938. Front Microbiol. 2017;8:1040.
- Li M, Lee K, Hsu M, et al. Lactobacillus-derived extracellular vesicles enhance host immune responses against vancomycin-resistant enterococci. BMC Microbiol. 2017;17(1):66.
- van Baarlen P, Wells JM, Kleerebezem M. Regulation of intestinal homeostasis and immunity with probiotic lactobacilli. Trends Immunol. 2013;34(5):208–215.
- Karczewski J, Troost FJ, Konings I, et al. Regulation of human epithelial tight junction proteins by Lactobacillus plantarum in vivo and protective effects on the epithelial barrier. Am J Physiol Gastrointest Liver Physiol. 2010;298(6):G851–9.
- Kim MH, Choi SJ, Choi H-I, et al. Lactobacillus plantarum-derived extracellular vesicles protect atopic dermatitis induced by staphylococcus aureus-derived extracellular vesicles. Allergy Asthma Immunol Res. 2018;10(5):516–532.
- Mantovani A, Biswas SK, Galdiero MR, et al. Macrophage plasticity and polarization in tissue repair and remodelling. J Pathol. 2013;229(2):176–185.
- Murray PJ, Wynn TA. Protective and pathogenic functions of macrophage subsets. Nat Rev Immunol. 2011;11(11):723–737.
- Biswas SK, Mantovani A. Macrophage plasticity and interaction with lymphocyte subsets: cancer as a paradigm. Nat Immunol. 2010;11(10):889–896.
- Shapouri-Moghaddam A, Mohammadian S, Vazini H, et al. Macrophage plasticity, polarization, and function in health and disease. J Cell Physiol. 2018;233(9):6425–6440.
- Martinez FO, Gordon S. The M1 and M2 paradigm of macrophage activation: time for reassessment. F1000Prime Rep. 2014;6:13.
- Anderson CF, Mosser DM. A novel phenotype for an activated macrophage: the type 2 activated macrophage. J Leukoc Biol. 2002;72(1):101–106.
- Wang L-X, Zhang S-X, Wu H-J, et al. M2b macrophage polarization and its roles in diseases. J Leukoc Biol. 2019;106(2):345–358.
- Hristodorov D, Mladenov R, von Felbert V, et al. Targeting CD64 mediates elimination of M1 but not M2 macrophages in vitro and in cutaneous inflammation in mice and patient biopsies. MAbs. 2015;7(5):853–862.
- Kasraie S, Werfel T. Role of macrophages in the pathogenesis of atopic dermatitis. Mediators Inflamm. 2013;2013:942375.
- Park K-S, Svennerholm K, Shelke GV, et al. Mesenchymal stromal cell-derived nanovesicles ameliorate bacterial outer membrane vesicle-induced sepsis via IL-10. Stem Cell Res Ther. 2019;10(1):231.
- da Silva TA, Roque-Barreira MC, Casadevall A, et al. Extracellular vesicles from Paracoccidioides brasiliensis induced M1 polarization in vitro. Sci Rep. 2016;6(1):35867.
- Thery C, Witwer KW, Aikawa E, et al. Minimal information for studies of extracellular vesicles 2018 (MISEV2018): a position statement of the international society for extracellular vesicles and update of the MISEV2014 guidelines. J Extracell Vesicles. 2018;7(1):1535750.
- Lee E-Y, Choi D-Y, Kim D-K, et al. Gram-positive bacteria produce membrane vesicles: proteomics-based characterization of staphylococcus aureus-derived membrane vesicles. Proteomics. 2009;9(24):5425–5436.
- Lee EJ, Kim JY, Ahn Y, et al. Critical Role of ATP-P2X7 Axis in UV-Induced Melanogenesis. J Invest Dermatol. 2019;139(7):1554–1563 e6.
- Bustin SA, Benes V, Garson JA. et al. The MIQE guidelines: minimum information for publication of quantitative real-time PCR experiments. Clin Chem. 2009;55(4):611–622.
- Jun SH, Lee JH, Kim SI, et al. Staphylococcus aureus-derived membrane vesicles exacerbate skin inflammation in atopic dermatitis. Clin Exp Allergy. 2017;47(1):85–96.
- Hong S-W, Kim M-R, Lee E-Y, et al. Extracellular vesicles derived from Staphylococcus aureus induce atopic dermatitis-like skin inflammation. Allergy. 2011;66(3):351–359.
- Arango Duque G, Descoteaux A. Macrophage cytokines: involvement in immunity and infectious diseases. Front Immunol. 2014;5:491.
- Bhattacharya P, Budnick I, Singh M, et al. Dual role of GM-CSF as a pro-inflammatory and a regulatory cytokine: implications for immune therapy. J Interferon Cytokine Res. 2015;35(8):585–599.
- Shi C, Pamer EG. Monocyte recruitment during infection and inflammation. Nat Rev Immunol. 2011;11(11):762–774.
- Dobrovolskaia MA, Vogel SN. Toll receptors, CD14, and macrophage activation and deactivation by LPS. Microbes Infect. 2002;4(9):903–914.
- Yang L, Froio RM, Sciuto TE, et al. ICAM-1 regulates neutrophil adhesion and transcellular migration of TNF-alpha-activated vascular endothelium under flow. Blood. 2005;106(2):584–592.
- Bernatchez SF, Atkinson MR, Parks PJ. Expression of intercellular adhesion molecule-1 on macrophages in vitro as a marker of activation. Biomaterials. 1997;18(20):1371–1378.
- Deshmane SL, Kremlev S, Amini S, et al. Monocyte chemoattractant protein-1 (MCP-1): an overview. J Interferon Cytokine Res. 2009;29(6):313–326.
- Starr T, Bauler TJ, Malik-Kale P, et al. The phorbol 12-myristate-13-acetate differentiation protocol is critical to the interaction of THP-1 macrophages with Salmonella Typhimurium. PLoS One. 2018;13(3):e0193601.
- Genin M, Clement F, Fattaccioli A, et al. M1 and M2 macrophages derived from THP-1 cells differentially modulate the response of cancer cells to etoposide. BMC Cancer. 2015;15(1):577.
- Arnold CE, Whyte CS, Gordon P, et al. A critical role for suppressor of cytokine signalling 3 in promoting M1 macrophage activation and function in vitro and in vivo. Immunology. 2014;141(1):96–110.
- Ohteki T. Critical role for IL-15 in innate immunity. Curr Mol Med. 2002;2(4):371–380.
- Quero L, Hanser E, Manigold T, et al. TLR2 stimulation impairs anti-inflammatory activity of M2-like macrophages, generating a chimeric M1/M2 phenotype. Arthritis Res Ther. 2017;19(1):245.
- Relloso M, Puig-Kröger A, Pello OM, et al. DC-SIGN (CD209) expression is IL-4 dependent and is negatively regulated by IFN, TGF-beta, and anti-inflammatory agents. J Immunol. 2002;168(6):2634–2643.
- Tong L, Li J, Choi J, et al. CLEC5A expressed on myeloid cells as a M2 biomarker relates to immunosuppression and decreased survival in patients with glioma. Cancer Gene Ther. 2019. DOI:10.1038/s41417-019-0140-8
- Roszer T. Understanding the mysterious M2 macrophage through activation markers and effector mechanisms. Mediators Inflamm. 2015;2015:816460.
- Bi Y, Chen J, Hu F, et al. M2 macrophages as a potential target for antiatherosclerosis treatment. Neural Plast. 2019;2019:21.
- Kowal K, Silver R, Sławińska E, et al. CD163 and its role in inflammation. Folia Histochem Cytobiol. 2011;49(3):365–374.
- Mantovani A, Sica A, Sozzani S, et al. The chemokine system in diverse forms of macrophage activation and polarization. Trends Immunol. 2004;25(12):677–686.
- Sanmarco LM, Ponce NE, Visconti LM, et al. IL-6 promotes M2 macrophage polarization by modulating purinergic signaling and regulates the lethal release of nitric oxide during Trypanosoma cruzi infection. Biochim Biophys Acta Mol Basis Dis. 2017;1863(4):857–869.
- Braune J, Weyer U, Hobusch C, et al. IL-6 regulates M2 polarization and local proliferation of adipose tissue macrophages in obesity. J Immunol. 2017;198(7):2927–2934.
- Suzuki K, Meguro K, Nakagomi D, et al. Roles of alternatively activated M2 macrophages in allergic contact dermatitis. Allergol Int. 2017;66(3):392–397.
- Conway EM, Pikor LA, Kung SHY, et al. Macrophages, inflammation, and lung cancer. Am J Respir Crit Care Med. 2016;193(2):116–130.
- Jang SC, Kim OY, Yoon CM, et al. Bioinspired exosome-mimetic nanovesicles for targeted delivery of chemotherapeutics to malignant tumors. ACS Nano. 2013;7(9):7698–7710.
- Kim Y-S, Kim J-Y, Cho R, et al. Adipose stem cell-derived nanovesicles inhibit emphysema primarily via an FGF2-dependent pathway. Exp Mol Med. 2017;49(1):e284.
- Muizzuddin N, Maher W, Sullivan M, et al. Physiological effect of a probiotic on skin. J Cosmet Sci. 2012;63(6):385–395.
- van Baarlen P, Troost FJ, van Hemert S, et al. Differential NF-kappaB pathways induction by Lactobacillus plantarum in the duodenum of healthy humans correlating with immune tolerance. Proc Natl Acad Sci U S A. 2009;106(7):2371–2376.
- Lu C-H, Lai C-Y, Yeh D-W, et al. Involvement of M1 macrophage polarization in endosomal toll-like receptors activated psoriatic inflammation. Mediators Inflamm. 2018;2018:3523642.
- McLaughlin T, Ackerman SE, Shen L, et al. Role of innate and adaptive immunity in obesity-associated metabolic disease. J Clin Invest. 2017;127(1):5–13.
- Abraham C, Dulai PS, Vermeire S, et al. Lessons learned from trials targeting cytokine pathways in patients with inflammatory bowel diseases. Gastroenterology. 2017;152(2):374–388 e4.
- Grangette C, Nutten S, Palumbo E, et al. Enhanced antiinflammatory capacity of a Lactobacillus plantarum mutant synthesizing modified teichoic acids. Proc Natl Acad Sci U S A. 2005;102(29):10321–10326.
- Cox KH, Cox ME, Woo-Rasberry V, et al. Pathways involved in the synergistic activation of macrophages by lipoteichoic acid and hemoglobin. PLoS One. 2012;7(10):e47333.
- Kim HG, Lee SY, Kim NR, et al. Inhibitory effects of Lactobacillus plantarum lipoteichoic acid (LTA) on Staphylococcus aureus LTA-induced tumor necrosis factor-alpha production. J Microbiol Biotechnol. 2008;18(6):1191–1196.
- van Hemert S, Meijerink M, Molenaar D, et al. Identification of Lactobacillus plantarum genes modulating the cytokine response of human peripheral blood mononuclear cells. BMC Microbiol. 2010;10:293.
- Huang-Doran I, Zhang C-Y, Vidal-Puig A. Extracellular vesicles: novel mediators of cell communication in metabolic disease. Trends Endocrinol Metab. 2017;28(1):3–18.
- Tsoi LC, Rodriguez E, Degenhardt F, et al. Atopic dermatitis is an IL-13-dominant disease with greater molecular heterogeneity compared to psoriasis. J Invest Dermatol. 2019;139(7):1480–1489.
- Tokunaga R, Zhang W, Naseem M, et al. CXCL9, CXCL10, CXCL11/CXCR3 axis for immune activation - – A target for novel cancer therapy. Cancer Treat Rev. 2018;63:40–47.
- Ge B, Li J, Wei Z, et al. Functional expression of CCL8 and its interaction with chemokine receptor CCR3. BMC Immunol. 2017;18(1):54.
- Liao W, Lin JX, Leonard WJ. IL-2 family cytokines: new insights into the complex roles of IL-2 as a broad regulator of T helper cell differentiation. Curr Opin Immunol. 2011;23(5):598–604.
- Couper KN, Blount DG, Riley EM. IL-10: the master regulator of immunity to infection. J Immunol. 2008;180(9):5771–5777.