Abstract
In recent years, integrating biological components in analytical instruments especially in biomedical research has become a prerequisite for early diagnosis of many diseases. It is well known that the material properties (electrical and physical) of CNTs is very sensitive to be affected by exposure to biomolecules and this led to the investigation by many researchers. Though the CNT-based biosensors has been widely used due their better performance, it still has many practical concerns in application. For the successful commercialization of the concept of CNT-based biosensors, many hurdles need to overcome. Modifications on CNT biosensors have experienced a dramatic change with outstanding developments. The present article provides an overview on the recent development in CNT biosensors and comprehensive analysis was given on various ways to improve the performance of CNT with new designs. In addition, some of the practical applications and concerns in the field are addressed. The scientific and technological challenges in the field are discussed in the conclusion.
Graphical Abstract
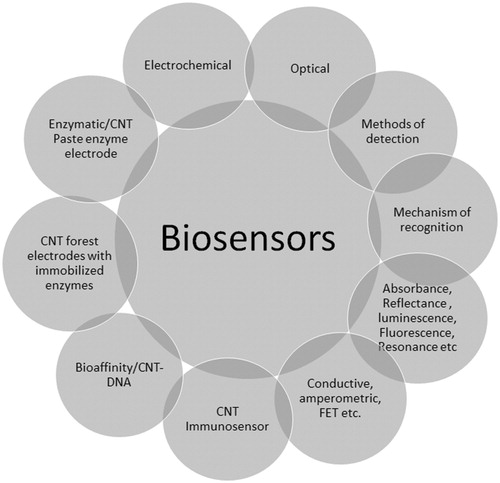
1. Introduction
The first biosensor [Citation1] was evolved about a century ago in 1906 but the true biosensor was developed in 1956 by Clark et al., and became father of biosensors [Citation2] subsequently 1962 he demonstrated an amperometric enzyme electrode for glucose detection. Guilbault and Montalvo discovered potentiometric biosensor in 1969 by using glass electrodes [Citation3]. Interestingly, Yellow Spring Instruments (YSI) was developed the first commercial biosensors in 1975. shows some events in the history of biosensors till date and the database ‘Web of Science’ has indexed over 4150 reports on the topic of ‘carbon nanotubes in biosenors’ from 2007 to 2018 (till 8 May 2018) is presented in . In addition, according to the list of publications, China occupies a prime position in developing such biosensors based on CNTs (see ). Though the CNTs-based biosensors was invented in early 2000s [Citation4–8], the real-time medical equipment is still in progressive. In general, the composition of CNT-based biosensor includes two main parts: biological sensitive element and transducer as shown in . The role of each component is stated in the legend of , transducer is to convert the concentration of analytes to other detectable physical signal, such as currents, absorbance, mass or acoustic variables for testing and detecting [Citation9]. CNT-based biosensors were categorized into two: (chemical and physical) based on the interactions between the analytes and the biological sensitive materials.
Figure 2. (a) Publications related to ‘carbon nanotubes in biosensors’ searched using ‘Web of Science’ this data includes regular articles, review articles, book chapters and conference proceedings and (b) Top 20 countries of research community working on CNTs-based biosensors.
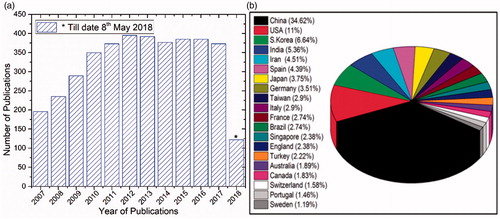
Figure 3. A schematic representation of a biosensor, the physical or chemical reaction is transformed into chemical signals after the target molecule was detected166. Where Analyte is a interest that needs to be detected (e.g. glucose is an ‘analyte’ in a biosensor designed to detect glucose); Bioreceptor is a that specifically recognizes the analyte (e.g. Enzymes, cells, aptamers, DNA and antibodies); The process of signal generation (in the form of light, heat, pH, charge or mass change, etc.) upon interaction of the bioreceptor with the analyte is termed bio-recognition; Transducer is an element that converts one form of energy into another; Electronics is the part of a biosensor that processes the transduced signal and prepares it for display; Display: The display consists of a user interpretation system such as the liquid crystal display of a computer or a direct printer that generates numbers or curves understandable by the user. This part often consists of a combination of hardware and software that generates results of the biosensor in a user-friendly manner.
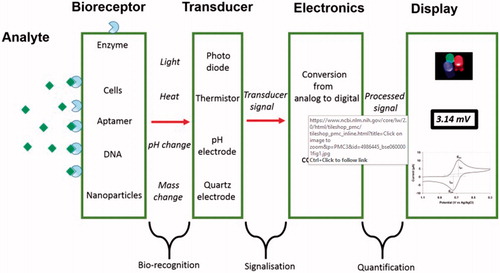
In description, carbon nanotubes (CNTs) are pseudo-one-dimensional allotropes of carbon, notably recognized as a network of carbon atoms assembled into seamless cylinders of one or more layers with either open or closed ends [Citation10–12]. During the past few years, CNTs have drew attention in healthcare system as one of the prospective contenders for medical devices owing to their several unique properties viz. excellent mechanical strength [Citation13], high surface area, excellent electrical conductivity [Citation14], electrochemically stable in aqueous and nonaqueous solutions and high thermal conductivity [Citation15]. In short, biosensor refers to a diagnostic device that transfigures a biological event into a measurable electrical signal. The remarkable sensitivity of CNTs toward their surface ensures that they serve as an ideal material for high sensitive nanoscale biosensor devices [Citation16–18]. But, the strong intermolecular π-π interactions, and hydrophobic nature of CNTs is a major obstacle for developing CNTs-based biosensors. In order to improve their solubility and stability, different functionalities of the CNTs have been improved by functionalizing through chemical adsorption [Citation19–21]. Many reports have emerged over the last decade showing that functionalized CNTs have an ability to address several concerns related to biocompatibility and toxicology [Citation22–24].
CNTs are often chosen as working electrodes for sensing devices owing to their extraordinary low detection limit [Citation24–26]. The hollow structure of CNTs is good for the adsorption of enzyme. Therefore, in amperometric CNTs-based biosensors, CNTs are always used to functionalize with enzyme to generate the enzyme CNT electrodes or to modify the surface of electrodes. For example, Eguílaz et al. reported that covalently functionalized SWCNT with polytyrosine electrode has an efficiency to generate surface Quinone groups to facilitate the electrooxidation of nicotinamide adenine dinucleotide based biosensors. The functionalization of SWCNT with polytyrosine has also show to be an efficient dispersion medium for these nanostructures [Citation27]. Burrs et al. demonstrated electrochemical biosensors which works with functionalized graphene (GR) paper for the detection of small molecules (glucose) or pathogen bacteria (Escherichia coli O157:H7) by using fractal platinum nanocauliflower [Citation28]. According to different analytes, different enzymes are selected, such as nicotinamide adenine dinucleotide (NADH) [Citation6,Citation8], glucose oxidase (GOD) [Citation29,Citation30], aflatoxin-oxidase [Citation7], cholesterol oxidase [Citation31], urease [Citation32], lactic acid oxidase [Citation33], horseradish peroxidase [Citation34,Citation35] and so on.
The recent and fast growth in CNTs has strongly influenced the medical sector because CNTs very sensitive to be affected by exposure to biomolecules as sensing elements for biosensors. A great deal of effort has been put over in the last decade worldwide in development of new biosensors with high sensitivity for the rapid detection of targeted substrates.
2. Functionalization of CNTs
Although, CNTs poses several attractive properties and advantages, dispersion activated by the high surface energy of the CNTs marks as an obstruction to progress any further. CNTs poses high hydrophobicity that makes them insoluble in water and other common solvents. Therefore, depending on application, CNTs needs to be functionalized to improve their solubility and other functional properties [Citation36,Citation37]. In fact, the functionalization of CNTs has become a prerequisite in order to enable facile fabrication of nanodevices. Among all the functionalization approaches, non-covalent and covalent surface modifications are two commonly used methods. The main advantage of non-covalent functionalization that their structure and their original properties remain the same, and becomes extremely difficult to modify further. Direct covalent functionalization can significantly alter the solubility and compatibility of CNTs [Citation38]. Mananghaya et al. reported that functionalized CNTs (f-CNTs) significantly improved the solubility [Citation39] and thereby performance and lowered their toxicity [Citation40]. But the disadvantage of covalent functionalization is that the structural homogeneity and other original properties of CNTs are changed thereby effecting mechanical properties [Citation41].
2.1. Non-covalent functionalization of CNTs
In order to improve CNTs biocompatibility, peptide immobilization for drug delivery, protein assembly and activity enhancement, non-covalent functionalization is considered as one of the important approaches as no significant changes in physical properties occurs [Citation42]. One major advantage with this approach is that the conjugated electronic structure of the CNTs is preserved while improving their solubility quite extraordinarily. Non-covalent functionalization of CNTs through polyaromatic adsorption π-π stacking, CH-π stacking, protein adoption, and lipid adsorption and sometimes even through vander Waals forces was observed by Mittal et al. and Bai et al. [Citation41,Citation43]. Polymer matrix with silicone rubber was used to functionalize MWCNTs with polymethyl phenylsiloxane. In addition, these approaches are generally straightforward and used a wide range of functionalization agents such as surfactants, polymer, composites and biomolecules. Sodium dodecyl sulfate (SDS) was used to obtain almost individual SWCNTs under ultra-sonication and ultracentrifugation conditions [Citation44]. Polymers have been used inplace instead of surfactants, but it has been noticed that they do not improve the dispersion effectively. Similarly, anionic, cationic and non-ionic surfactants are also used for dispersion of SWCNTs, but the amount of surfactants used are generally very low to about 5% [Citation45]. Cytotoxic groups also play a greater part innon-covalent functionalization, but those functional groups may limit biological application of such f-CNTs.
Non-covalent triple functionalized SWCNTs were used to develop biosensors by simultaneous immobilization of pyrene derivatives to nanotube sidewalls by dip coating method [Citation46]. Tu and Zheng reported that single-stranded-DNA complex molecule based biosensors with SWCNTs [Citation47] however, solubility of SWCNTs still remain challenge and these SWCNTs are unstable for use in gene delivery kind of applications [Citation48]. In other studies, highly water-soluble SWCNTs functionalized with PEGylated phospholipids were developed as a noninvasive diagnostic tool for cancer drug loading and delivery [Citation49,Citation50]. The same research group reported MWCNTs grafted with polyethylenimine to enhance binding properties of DNA which demonstrated high sensitivity and low toxic CNT-based DNA sensors for efficient gene delivery [Citation51]. Sanz et al. demonstrated RNA wrapping approach for the CNTs can have a better solubility and also demonstrated cytotoxic free [Citation52]. In addition, chemical modification of CNTs are reported and will be discussed in later sections.
2.2. Covalent functionalization of CNTs
As the perfect structure of CNTs is destroyed by covalent functionalization, Recently Setaro et al. reported an exceptional way to preserve the π-conjugated in MWCNT electronic structure to reconstruct the lost electronic properties by [2 + 1] cycloaddition, based on electron-poor aromatic azide [Citation53]. In another study, CNTs adsorbs proteins molecules and this can prevented by using polymer nonspecific bindings. Polyethylene glycol (PEG) is used to remove the proteins from the surface which are nonspecific in nature [Citation54,Citation55]. Triton-X surfactant containing an aliphatic chain and a short hydrophilic PEG unit was used to bind the nanotube surface by hydrophobic interactions. Connell et al. reported that the non-covalent functionalization of SWCNTs in the presence of surfactant (sodium dodecyl sulfate; SDS) and PVP shows the CNTs associate with a single layer of polymer for better dispersion [Citation44]. Liu et al. described that MWCNTs were functionalized using a non-covalent approach by using a grafted copolymer [Citation56]. Because of unavailability of carboxyl groups on the grafted surface, this particular approach can be applicable only for acid treated MWCNTs, but not for pristine MWCNTs. Kang et al. reported a hydrophobic polymer containing non-polar groups and a polymer with a polar hydrophilic side groups, a water-soluble polymer and two negatively charged side groups that can be used for the dispersion of SWCNTs for producing ribbon-type super structure. It should be noted that, the strong π-π covalent interactions between CNTs and polymers have imparted the wrapping of CNTs, and the solubility of CNTs in water has been improved by hydrophilic side groups present [Citation57]. In one of the study, it has been showed that ssDNA are made to bind with CNTs by π-stacking linear adsorption fashion or simply helical wrapping. Such structures facilitates CNT dispersion in electronic medium and can be a possible resolution for differentiating CNTs according to their electronic properties. The estimated properties can be made feasible since hydrophilic sugar-phosphate group provides the negative charge to the surface [Citation58]. By forming a precise directional π-π stacking with the CNTs, aromatic compounds with large number of chains and their derivatives were also made to disperse aqueous media more efficiently. Pyrene derivatives can also be used to anchor proteins or biomolecules on CNTs surface as the interactions between the polyaromatic backbone of pyrene and CNTs are permanent. There is an another class of polyaromatic molecules, viz. substituted anthracenes, heterocyclic polyaromatic porphyrins [Citation59] and phthalocyanines [Citation60] were used to disperse CNTs via the non-covalent method. Star et al. demonstrated that, water-soluble polyvinyl alcohol can be coated around the nanotubes to allow the dispersion of nanotubes in water. CNTs were also wrapped with starch to generate starched nanotubes [Citation61]. Long-chain polymers like Gum Arabic were also physically adsorbed to disperse the nanotubes individually in solvents [Citation62]. Aromatic polyimides were also used as functionalizing polymer to achieve non-covalent functionalization of CNTs [Citation63]. Different covalent functionalization methods on CNTs toward biological applications are presented in and .
There are numerous established techniques to functionalize CNTs, most of them are based on chemical methods [Citation64]. One advantage of chemical functionalization is that there is strong covalent bonding between CNT and coupling agents. The disadvantages include (1) harsh or sometimes expensive chemicals are involved in the functionalization; (2) non-expensive strong inorganic acids can be chosen, but due to the cutting and etching effects, their high probability of functionalized CNTs losing their partial mechanical strength; (3) usually long purification periods are preferred for most of the chemical functionalization. Functionalization with inorganic substances were also reported by Fu et al. [Citation65].
Figure 4. Different surface modifications of CNTs [Citation185]. (a) Aromatic molecules can be appended to nanotubes using certain non-covalent interactions (known as π–π interactions). Groups emanating from these molecules interact with the surrounding solvent or matrix. (b) Non-covalent interactions (including π–π interactions, van der Waals forces and charge-transfer interactions) can be used to wrap polymers around nanotubes and (c) Chemical groups can be covalently attached to nanotubes. In this case, lithium (Li) forms anions on the nanotube, which react with styrene monomers to form polystyrene chains covalently attached to the nanotube walls.
![Figure 4. Different surface modifications of CNTs [Citation185]. (a) Aromatic molecules can be appended to nanotubes using certain non-covalent interactions (known as π–π interactions). Groups emanating from these molecules interact with the surrounding solvent or matrix. (b) Non-covalent interactions (including π–π interactions, van der Waals forces and charge-transfer interactions) can be used to wrap polymers around nanotubes and (c) Chemical groups can be covalently attached to nanotubes. In this case, lithium (Li) forms anions on the nanotube, which react with styrene monomers to form polystyrene chains covalently attached to the nanotube walls.](/cms/asset/ae350bdf-a302-44d3-a08a-075fc4878f27/ynan_a_1478765_f0004_c.jpg)
Figure 5. Covalent and non-covalent interactions between CNTs and linear, dendritic and hyperbranched polymers and also biomacromolecules for producing CNT–polymer hybrid nanomaterials as anticancer drug delivery systems and Surface functionalization of the CNTs for biomedical applications [Citation186].
![Figure 5. Covalent and non-covalent interactions between CNTs and linear, dendritic and hyperbranched polymers and also biomacromolecules for producing CNT–polymer hybrid nanomaterials as anticancer drug delivery systems and Surface functionalization of the CNTs for biomedical applications [Citation186].](/cms/asset/5d072866-9627-450e-a8d3-265c3cb04910/ynan_a_1478765_f0005_c.jpg)
3. CNTs-based Biosensors
By virtue of the remarkable structural, optical, electronic and mechanical properties of CNTs offer diverse features of interest to engineer new generation probes in CNTs-based biosensors. As mentioned earlier, CNTs have a large specific surface area enables immobilization of a large number of functional units such as receptor moieties for biosensing and CNTs exhibits unique intrinsic optical properties such as photoluminescence in the near infrared (NIR) [Citation66,Citation67] and strong resonance Raman scattering [Citation68], making them as an excellent candidate for biological detection. Other interesting property is photothermal response that could reduce the tumor size or even completely removed by using NIR laser irradiation to generate heat. Different types of CNTs has different properties, for example, SWCNTs has unusual electronic property, based on the one-dimensional quantum effect. CNTs have multiplex functionalization capability through conjugation, thereby potentially enhancing recognition and signal transduction processes. The CNT could be able to conduct electricity ∼100 times greater than copper which support for transduction of electric signals generated upon recognition of a target [Citation69–73]. An ideal biosensor should have good physical and chemical as well as biological properties. CNTs could easily cross the biological membranes which makes them applicable in vivo with minimal invasiveness, and further implemented to photoacoustic. CNTs can be made biocompatible through various dispersion and functionalization methods. The more toxic CNTs the lesser density of surface functional groups, less toxic the more density of surface functional groups [Citation74]. By making the surface of pristine CNTs into hydrophilic f-CNTs the biocompatibility and biodegradability was increased [Citation75]. Numerous series of CNT biosensors have been established till date to examine an extensive range of cancer biomarkers by means of conjugation of DNA or aptamers, antibodies, peptides, proteins, or enzymes [Citation18,Citation76,Citation77]. Wang and Dai described an electrochemical biosensor for early stage discovery of cancer biomarkers [Citation78] and immunosensors based CNTs were also reported by Veetil et al. [Citation79]. However, it is well recognized that still there are quite a few challenges that need to be overcome for successful integration of immunosensors type of biosensors. Optical biosensors, on other hand provides a powerful substitute and offers a great advantage over a conventional analytical techniques by serving real-time and label-free detection just by analyzing the differences in the radiation of light (UV, visible or infrared). Sensitivity of the biosensors have been improved by increasing the interaction between the guided light and the sensor surface [Citation80]. shows the different types of CNT-based biosensor and a clear discussion is given in the following sections.
4. Electrochemical biosensors
With the intention to develop ultrahigh sensitive biosensors, various signal amplification strategies with CNTs have been developed. CNTs possess interesting electrochemical properties owing to the existence of reactive assemblies on the outer surface. CNTs have been accepted as a very favorable material for electrochemical biosensors as they have a capability of enhancing the electron transfer [Citation18,Citation73,Citation78,Citation81]. Electrochemical biosensors works on a principle where two or three electrode electrochemical cell (reference, working and counter electrode), converts/transfers a biological event into electrochemical signal. CNT-based electrochemical biosensors exhibits, high sensitivity, low production cost, faster response, ease of operation and promising portability makes them superior materials in biomedical applications. Based on the method of detection electrochemical biosensors are divided into two categories namely (i) bioenzymatic and (ii) bioaffinity sensors.
4.1. Bioenzymatic electrochemical biosensors
CNTs-based enzymatic electrochemical biosensors, utilizes bio-specificity of the enzymatic reaction to direct electrical transportation between biomolecules, particularly enzymes and CNT bulk electrode materials [Citation82]. There have been several reports based on various enzymes such as glucose oxidase (Gox) [Citation83], horse radish peroxidase (HRP) [Citation84], lactate oxide [Citation85] and malate dehydrogenase (MDH) [Citation86]. To analyze agribusiness, Zappi et al. developed glassy carbon printed electrodes by utilizing MWCNT, graphene, gold nanoparticles as nanomaterials for developing new disposable amperometric biosensors. Electrocatalyzed redox processes have been achieved in these biosensors by utilizing gold nanoparticle conducting property by providing useful interfaces in biochemical reactions [Citation87]. However, stability and bioactivity of the bioenzymatic electrochemical biosensors is always a challenging factor. Different strategies were reported in the literature which have addressed bioactivity, namely, (i) CNT paste enzyme electrodes, (ii) immobilization of enzymes on CNT-modified electrodes and (iii) CNT forest electrodes with immobilized enzymes. By means of physical absorption, DNA oligonucleotides can be trapped onto the chitosan wrapped CNTs for the detection of salmon sperm DNA [Citation88]. It was informed that immobilization of DNA onto the CNT electrode surface can boost the electroactive surface area by three folds. To augment the sequence-specific DNA detection sensitivity, Cai et al. reported the immobilization in CNT-based DNA covalently for electrochemical sensors by forming covalent amide bonds between the COOH/CNTs and the amine groups of the DNA oligonucleotides [Citation89].
4.1.1. CNT paste enzyme electrode
CNT paste was made with brumoform and constructed electrodes showed superior performance over other carbon electrodes [Citation90]. In another study, Rubianes et al. studied that CNTs paste mixed with mineral oil and their developed electrodes resulted in excellent electrocatalytic activity toward ascorbic acid, uric acid, dopamine, 3,4-dihydroxyphenylacetic acid (dopc) and hydrogen peroxide. The detection performance of such electrodes was up to 0.6 mM when GOx present [Citation91].
4.1.2. CNT modified electrodes with immobilized enzymes
As mentioned earlier, non-covalent and covalent functionalization are the two main methodologies for linking enzymes CNTs. Especially, when enzymes are linked with a non-covalent method, they can provide high surface loading, but, due to the weak interaction between enzyme and CNTs, further modification with polymer and nanoparticles is needed. Cai et al, has used nafion as a binding agent to grip the CNT composite mixture on to the electrode to enhance direct transfer of glucose oxidase (GOx). The slope (−53 mV/pH) obtained from plotting electron dependence versus pH suggested that the direct electron transfer of GOx is a two electron transfer pattern rather than the frequently occurring two proton transfer in a redox reaction process [Citation83]. In one of their work, Zhu et al. a nanocomposite film with bamboo shaped CNTs and chitosan for the immobilization of horseradish peroxidase enzyme. Chitosan offers to solubilize CNTs making the composite film hydrophilic and simplifies the whole immobilization of enzymes without using any severe reaction conditions and complicated procedures. The immobilized GOx and HRP showed an excellent bioelectrocatalytic activity toward H2O2 [Citation91]. In another example, Pt nanoparticles with a diameter of 2–3 nm deposited onto a nafion-containing GOx/CNTs film and enabled the composite structure to be used as an electrode material. The designed electrochemical glucose sensor showed a response time below 3 sec and a detection limit of upto 0.5 μM [Citation92].
Enzyme adsorption is another approach for enzyme immobilization in which a layer-by-layer method is involved. In one of the studies, through electrostatic adsorption, ionic liquids and glucose oxidase (GOx) are made to attach on-to the poly (sodium 4-styrenesulfonate (PSS) surface which is coated by CNTs, resulting in a high glucose oxidation rate [Citation93]. It is understood that CNTs which are vertically aligned, coupled with stimulant on their target tips can enable quick transfer of electrons compared to other architectures [Citation94]. In order to address the enzyme leakage, a covalent conjugation approach was followed to immobilize malate dehydrogenase (MDH) on MWCNT coated and screen-printed CNT electrodes [Citation87]. The detection limit upto 60–120 μM and short response time of 60 s was obtained. Yu et al. reported that the detection limit up to 70 nM and 50 nM for SWCNTs attached with myoglobin and HRP respectively [Citation95].
4.1.3. CNT forest electrodes with immobilized enzymes
CNT forest electrodes, commonly fabricated by chemical vapor deposition (CVD) technique that have a great control on structure and morphology of CNTs during the production also delivers more opportunities intended for the effective design of electrode. Wang et al. established a novel glucose biosensor of gold/CNTs/GOx by growing CNT forest on silicon substrate initially and then coating it by a thick e-beam coating of gold film. The newly fabricated glucose biosensor with electrode gold/CNTs-GOx exhibited rapid response time and a low detection limit with almost 0.01 mM with high sensitivity and stability values to glucose [Citation96]. In another study, for enzymatic assay, cholesterol biosensors were designed with vertically aligned CNT forests on Ti/Au electrode to monitor cholesterol in the blood. The large quantity of immobilized enzymes on the CNTs forest yielded high signal current and obtained a wider evident range of cholesterol in biological genetic samples [Citation97]. Even though nitric oxide is considered as a cell signal molecule, its molecular concentration, difficulty in detection and production effects has limited its application in vivo. To address this, Zheng et al. has used functionalized CNTs-based biosensors for the molecular detection of nitric oxide by means of DNA oligonucleotide adsorbed and wrapped around SWCNTs with a direct capability of quantifying nitric oxide concentrations selectively [Citation98]. Interference-free transducers of CNT-nanoelectrode glucose based biosensors were also developed for the selective detection of glucose and also to eliminate potential interference of hydrogen peroxide [Citation99] whereas structural configuration of GOx on SWCNT electrodes has also been developed elsewhere [Citation100]. As shown in , nonspecific proteins covalently immobilized on to the Au/CNTs surface [Citation101] and used as Raman tags. Non-enzymatic electrochemical biosensors have played a crucial role in oxidation of analyte catalyzed by electrocatalyst. Pt/MnO2/f-MWCNTs electrodes were engaged to identify catechin at a very low detection limit of 0.02 μM. Furthermore, enzyme free electrodes demonstrated to be able to detect red wine, black tea [Citation102] and green tea [Citation103].
Figure 6. Surface chemistry used to immobilize proteins on gold-coated glass slides for Raman detection of analytes by SWNT Raman tags. A self-assembled monolayer of cysteamine on gold was covalently linked to six-arm, branched poly (ethylene glycol)-carboxylate (6arm-PEG-COOH, right) to minimize nonspecific protein binding. Terminal carboxylate groups immobilize proteins. (b) Sandwich assay scheme. Immobilized proteins in a surface spot were used to capture an analyte (antibody) from a serum sample. Detection of the analyte by Raman scattering measurement was carried out after incubation of SWNTs conjugated to goat anti-mouse antibody (GaM-IgG-SWNTs), specific to the captured analyte. SWNTs were functionalized by (DSPE-3PEO) and (DSPE-PEG5000-NH2) (left). (c) Raman spectra of the SWNT G mode and radial breathing mode (RBM, inset) regions before and after SERS enhancement [Citation101].
![Figure 6. Surface chemistry used to immobilize proteins on gold-coated glass slides for Raman detection of analytes by SWNT Raman tags. A self-assembled monolayer of cysteamine on gold was covalently linked to six-arm, branched poly (ethylene glycol)-carboxylate (6arm-PEG-COOH, right) to minimize nonspecific protein binding. Terminal carboxylate groups immobilize proteins. (b) Sandwich assay scheme. Immobilized proteins in a surface spot were used to capture an analyte (antibody) from a serum sample. Detection of the analyte by Raman scattering measurement was carried out after incubation of SWNTs conjugated to goat anti-mouse antibody (GaM-IgG-SWNTs), specific to the captured analyte. SWNTs were functionalized by (DSPE-3PEO) and (DSPE-PEG5000-NH2) (left). (c) Raman spectra of the SWNT G mode and radial breathing mode (RBM, inset) regions before and after SERS enhancement [Citation101].](/cms/asset/5196d777-e712-4c4f-b122-9781d5a1dfed/ynan_a_1478765_f0006_c.jpg)
4.2. Bioaffinity electrochemical biosensors
Bioaffinity electrochemical biosensors are very stable complexes where biomolecules are strongly bounded to the sensor surface due to their bioaffinity. Bioaffinity electrochemical biosensors collect information from the target analyte by molecular recognition for example antibody, oligonucleotide, DNA, cells. It has been demonstrated that bioaffinity devices which make use of enzyme tags can significantly benefit from the superior response of the biocatalytic-reaction. Current electrochemical bioaffinity sensors, such as DNA biosensors and immunosensors have recently demonstrated an unlimited potential in carcinogenic related health care systems [Citation104]. CNT-DNA and CNT-Immunoelectrochemical sensors are being discussed in the following two sections.
4.2.1. CNTs-DNA electrochemical biosensors
To obtain a simple rapid inexpensive sensors, CNTs-DNA electrochemical biosensors were developed and advanced more rapidly based on nucleic acid recognition processes for testing/sensing molecular diagnosis, drug discovery genetic and infectious diseases [Citation105,Citation106]. In order to diagnose the genetic and infectious diseases, DNA hybridization is one of the most rapid, inexpensive and simple electrochemical biosensor [Citation107]. When a DNA is attached on to an electrode, the hybridization event is detected via a change in electrochemical parameters by the reactions caused by DNA hybridization. Nevertheless, when the electron transfer rate is substantially low and peak current is small, a mercury electrode is being used. To address this issue i.e. to increase electrochemical response rate without using mercury electrodes, electroactive indicators have been adapted into the CNT-DNA electrochemical biosensors to quicken the process. There were some studies which even reported the improvement of sensors without the indicators vaccination and gene therapy. Li et al. reported that the oligonucleotides immobilization onto CNT electrodes through physical absorption [Citation88]. Nevertheless, covalent attachment of CNTs and DNA electrochemical biosensors lead to fast electron transfer. For example, DNA based biosensors are functionalized with carboxylic acid groups and amino attached oligonucleotide probes at the end are made to attach COOH groups covalently to fasten electron transfer between electrode and the drug indicator with sensitivity of 1.0 × 10−10 mol/L [Citation89]. In another study, CNT-DNA based biosensors were fabricated by functionalizing them with MWCNTs–COOH and immobilization on-to an electrode surface using Mn(II) complex DNA intercalator and reported detection limit of 1.4 × 10−10 mol/L [Citation108]. After doing acetic acid-plasma treatment on Au coated CNTs, DNA with fixed sequences were attached on-to the tips and sidewalls of aligned CNTs for sensing DNA for highly selective electrochemical DNA sensors. By using amperiometric response of aligned CNTs, the sensitivity results showed 11.36 nm/mL and are much higher than conventional electrodes under similar conditions [Citation109]. Li et al. developed a CNT-nanoelectrode array for ultra-sensitive chemical DNA detection by entrenching it with SiO2. To strengthen the signal further for the detection, Ru(bpy)32+ mediators were engaged to target DNA. However, the authors did point out that the detection limit is considerably lower than the few oligonucleotide targets. It is found that the sensitivity has been much improved after optimization [Citation110]. Label free impedance spectral analysis has been used to observe indicator-free DNA immobilization with no hybridization markers. In this study as reported by Xu et al., ssDNA was covalently coupled with PPy/MWCNTs electrode and showed a significant lowered impedance with a good electronic transfer property. The limitations of double stranded dsDNA as a lower electronic transfer is mentioned and a detection limit of 5 × 10−12 mol L−1 was accomplished for the recognition of the DNA sequence without using a hybridization marker or intercalator [Citation111]. Chemically modified dimethylformamide SWCNTs electrode was used to as impedance DNA biosensor by using ssDNA probes and complementary DNA binding and was able to sense upto 1 × 10−9 mol/L [Citation112]. Guo et al. have reported an alternative class of DNA sensors based on the aptamers for sensitive detection of enzymes via mediated signal transduction without any covalent modification. Through aromatic exchanges between the nucleotide bases and SWCNTs sidewall, aptameric was made to shawl on the sidewalls of the CNTs [Citation113]. The detection was about 50 pM of thrombin was achieved through aptamer wrapping method ().
Table 1. Recent developments in biosensors based on SWCNTs/MWCNTs.
4.2.2. CNTs-Immunosensors
Immunosensors are condensed analytical biosensor devices which are based on ligand affinity, where immunochemical reaction interaction and molecular recognition between antigen and antibodies is coupled together to a transducer for selective detection of several kinds of proteins [Citation114]. The advancement of immunosensors technology is an achievement in the biomedicine and till today it continues to be an exciting area of research. Riberi et al. developed CNT-based electrochemical immunosensors to quantifyzearalenone (ZEA) by using carbon screen-printed electrodes modified with gold nanoparticles and MWCNT dispersions. The developed immunosensor displayed a limit of detection (0.15 pg/mL) and sensitivity (SC50 = 2 pg/mL) with an added advantage of minimum pre-treatment [Citation115]. In another study, Aziz et al. designed an amperometric immunosensor made up of Indium tin oxide (ITO) electrodes to detect the mouse IgG. An ITO electrode reformed completely with MWCNTs and PEG-silane to minimize nonspecific binding of proteins, is made to adsorb onto the unoccupied places of the MWCNTs-coated ITO electrode. Then an electrochemical immunosensor, avidin, was attached to the sidewalls of the CNTs to attach to antimouse IgG. When compared to the bare ITO electrode with PEG-silane modified electrode it showed no nonspecific binding of avidin. The detection limit was up to 10 pg/mL which is much smaller when equated to the other enzyme connected immunosorbent assays [Citation116]. In another study, conducting polymers were used as electroactive materials for increasing and decreasing the reaction rate and electrode response time, respectively. A polyethyleneimine polymer in branched form and carboxylic acid were used to bind CNTs to obtain a nanostructured surface in order to increase the immunoreactive electrode surface. After coating the electrode with Au, anti-cardiac troponin T was attached on the previously prepared polyethyleneimine and carboxylic acid CNTs electrode to generate a amperometric signal. A detection limit of 0.033 ng/mL was achieved with this kind of conductive polymer biosensor [Citation117].The electrochemical immunosensor demonstrated very low and elevated cancer-related detection of interleukin-6, a multifunctional cytokine by attaching SWCNT forests with enzymes and horseradish peroxidase. Interestingly, this developed biomarker detected serum levels above and below in the range ∼6 pg mL−1 [Citation118]. Wan et al. reported disposable screen-printed carbon electrodes based electrochemical immunosensors and demonstrated for the early detection of cancer with ultrahigh sensitivity [Citation119]. In addition, gold nanoparticles decorated MWCNTs based impedimetric immunosensors demonstrated successfully for the early detection of cancer cells in serum, especially for breast cancer patients. The blend of highly conductive ionic liquid and MWCNTs together with gold nanoparticles significantly enhanced the sensitivity of the proposed immunosensor and also had an optimistic effects on the biocompatibility and amplification power. These kind of immunosensors are able to detect very low concentrations of HER2 in serum samples of breast malignant patients and exhibit a constant increase in charge-transfer resistance with concentration of human epidermal growth factor receptor 2 [Citation120]. In addition, novel electrochemical immunosensor strategy with randomly oriented vertically aligned CNTs was developed to detect cancer biomarker proteins by Munge et al. Initially, matrix metalloproteinase-3 (MMP-3), a cancer biomarker and protein antibody (Ab1) was attached onto the tips of SWCNTs trailed by the immobilization of antigens MMP-3. A secondary anti-MMP 3 antibody (Ab2) coated with horseradish peroxidase labels on 500 nm polystyrene beads was applied to develop an enormous molecular tag filled with nearly 4200 enzyme labels. By this process, an ultralow detection limit of 4 pg/mL in 10 mL serum sample was achieved [Citation121]. Hafaiedh et al. developed a gold labeled SWCNT immunosensor for the selective detection of Anti-IGg immobilization [Citation122]. In another study, platinum/CNT electrodes were developed to study the electrochemical behavior of cysteine (CySH), an vital amino acid in biological systems [Citation123]. Biosensors based on CNT arrays have been applied to detect several types of biomarkers like prostate-specific antigen -mAb and human chorionic gonadotropin hCG antibodies [Citation124]. represents the immunobiosensor for the detection of CD4 T cells.
Figure 7. Schematic representation of the SWCNT immunosensor and its selective CD4 T cell capture [Citation26].
![Figure 7. Schematic representation of the SWCNT immunosensor and its selective CD4 T cell capture [Citation26].](/cms/asset/7c60e587-8598-430b-92cc-644fbe933f00/ynan_a_1478765_f0007_c.jpg)
Detection of prostate-specific antigen (PSA) response was expressively enhanced after adjustment of MWCNTs with bipolar electrode materials [Citation125]. Besides MWCNTs with microsomal cytochrome P450 was used to sense drugs and were used in breast malignant disease [Citation126]. MWCNTs/Au were used in developing double stranded-DNA based biosensors for the finding of berberine [Citation127]. Liu et al. reported tricosane (C23H48)/f-SWCNTs for volatile organic compounds of lung cancer causing detection [Citation128]. As shown in , folic acid functionalized polydopamine-coated CNTs were used for the recognition of HeLa and HL60 cancer cells [Citation129]. One report presented a simple preparation of poly-l-lysine (PLL) electro-polymerized on the surface of functionalized multiwall carbon nanotubes (f-MWCNT) for electrochemical detection of palmatine content in human serum and urine samples [Citation18].
5. Optical Biosensors
The basic principle of optical biosensors is to detect and measure the interaction between the target biomolecules with the analytes by measuring of photons (absorbance, reflectance or fluorescence emissions) alternatively than electrons (). The detection of optical sensors can be luminescence, fluorescence and color changes. The exceptional unique optical properties of CNTs have gained extensive research for developing optical biosensors for biomarker detection and imaging during the past few years. SWCNTs are indeed characterized as biocompatible fluorescent probes by their virtue of emission between 700 and 1400 nm. This range of wavelength in SWCNTs allows the rays to have a deep penetration through layers of skin and in vivo imaging of biological tissues and organs can be made possible with an almost 15 times lower dose limit [Citation130]. As we know, SWCNTs based sensors offers the added advantage of high photostability, besides low autofluorescence. Kim et al. was able to develop a SWCNTs based optical sensor by utilizing the fluorescence property of SWCNTs for selective detection of adenosine 5′-triphosphate (ATP) in living cells (). By conjugating luciferase enzyme to polymer-coated nanotubes, spatial and temporal information of NIR detection in ATP living cells was achieved with a detection limit of 240 nM [Citation131].
Figure 8. Electrochemical and electronic CNT biosensors for cancer detection. (A) Schematic illustration of the folic acid-targeted cytosensing strategy for an enhanced electrochemical detection of cancer cells using polydopamine-coated CNTs. (B) Schematic representation of an electrochemical DNA biosensor for cancer detection based on gold nanoparticles/aligned CNTs [Citation187].
![Figure 8. Electrochemical and electronic CNT biosensors for cancer detection. (A) Schematic illustration of the folic acid-targeted cytosensing strategy for an enhanced electrochemical detection of cancer cells using polydopamine-coated CNTs. (B) Schematic representation of an electrochemical DNA biosensor for cancer detection based on gold nanoparticles/aligned CNTs [Citation187].](/cms/asset/c21c7522-e073-4597-bb41-93ea3e9f70e4/ynan_a_1478765_f0008_c.jpg)
Figure 9. SWCNT/luciferase hybrid for cellular ATP detection: (a) SWCNT-based sensor that is able to selectively recognize ATP against other interfering molecules such as AMP, AMP, CTP and GTP, which allows to spatiotemporally detect ATP in living cells. The oxidized production of d-luciferin, oxyluciferin, quenches the NIR fluorescence of SWCNT. (b) NIR fluorescence images of HeLa cells containing SWCNTLuc sensor (2 μg/mL) with and without addition of Lrin. The NIR fluorescence in HeLa cells is quenched after addition of Lrin (240 μM), which is correlated with the ATP concentration. Reproduced with permission from [Citation131].
![Figure 9. SWCNT/luciferase hybrid for cellular ATP detection: (a) SWCNT-based sensor that is able to selectively recognize ATP against other interfering molecules such as AMP, AMP, CTP and GTP, which allows to spatiotemporally detect ATP in living cells. The oxidized production of d-luciferin, oxyluciferin, quenches the NIR fluorescence of SWCNT. (b) NIR fluorescence images of HeLa cells containing SWCNTLuc sensor (2 μg/mL) with and without addition of Lrin. The NIR fluorescence in HeLa cells is quenched after addition of Lrin (240 μM), which is correlated with the ATP concentration. Reproduced with permission from [Citation131].](/cms/asset/07219313-254a-4ffe-9816-aaf05d0e78fc/ynan_a_1478765_f0009_c.jpg)
An apparent advantage of SWCNTs is the mixture of different chiralities (n,m) present and surprisingly each of these chiral structures can be used as a different color for optical multiplexing. Heller et al. developed an exceptional multimodal optical sensor for distinct response pathways by developing the first label-free device to recognize and measure alkylating and ROS activity in as-it-happens within the living cells [Citation132]. SWCNTs also have been exploited as NIR fluorescent tags for careful probing and imaging of cells. Due to the presence of a slight electronic band gap between valence and conduction band, CNTs exhibit photoluminescence in near infrared (NIR, wavelength ∼0.8–2 µm) region and established a property related to semiconducting SWCNTs. Many research groups have studied and developed SWCNT-fluorescence biosensors with capability of no blinking, no bleaching and a large Stokes shift [Citation44,Citation133,Citation134]. Welsher et al. developed hydrophilic and water-soluble polyethyleneglycol (PEG)-modified SWCNTs for probing cell surface receptors. By detecting the intrinsic NIR photoluminescence of SWCNTs, these receptors are selectively conjugated bonded to rituxan antibody [Citation66].
6. CNTs as a field effect transistor biosensors
Field effect transistors (FET) are the three-channel devices consisting of source (S), drain (D) and gate (G) electrodes, commonly used for weak-signal amplification. Gate electrode is meant to control the voltage generated in the device. Since Silicon based transistors have already reached their scaling limit, transistors based on CNTs are now being considered to be among the most promising candidates to replace established silicon FETs [Citation135]. It is also understood that, within the next decade, the device transistor footprint containing all components (S, D, G) can be reduced to less than 40 nm with an increase in current density [Citation136]. As we know, performance of CNTs as a transistor is been degraded by the presence of large hysteresis and this is considered as a major drawback as an energy efficient material. Recently Park et al. modified CNTs by depositing TiO2 of thickness (5, 10, 20 and 30 nm) as a gate dielectric to fabricate CNT-based FETs with less than 0.5% hysteresis for VLSI [Citation137]. In one biomedical study, Besteman et al. constructed and demonstrated the first biosensor based SWNTs by binding it with enzyme GOx to decrease conductance. But, when dissolved in glucose, these semiconducting SWNTs are found to act as reversible pH sensors, suggesting its usage as enzymatic biosensor [Citation138]. Martinez et al. fabricated an electronic device based on CNT arrays for the detection of DNA hybridization. shows a CNT-FET- back gated device on a 1 cm2 chip by using palladium as the contact metal [Citation139].
Figure 10. (top) Scanning electron micrograph of the array of CNTFETs. S: source. D: drain. G: contact to back gate. 896 pairs of S/D electrodes are packed in 1 cm2. (bottom) Schematic.
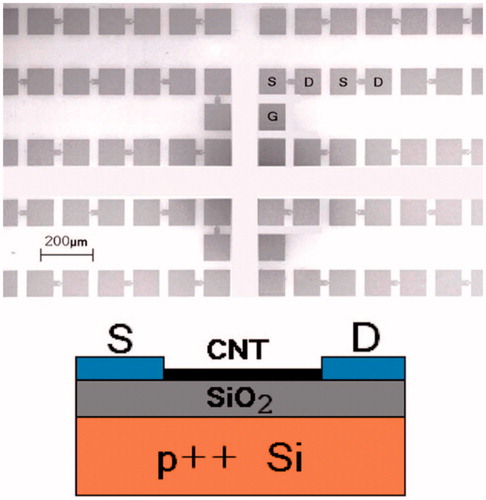
Authors observed significant changes in FET parameters after hybridization. In another study, CNT-FET was designed for the sensing of amyloid-β (Aβ) in human serum. Quantification of the concentration of antibodies immobilized on the CNT sensor surface was shown by using E. coli in the outer membrane (E. coli) which has high affinity towards antibodies. Therefore, antibodies at the low levels i.e. at 1 pg m/L in human serum could be measured as-it-happens without even labeling using CNTs-FET biosensors [Citation140]. Sudibya et al. demonstrated that the N-acetyl-d-glucosamine (GlcNAc)-fSWCNTs based FET biosensors regulated secretion (or exocytosis) of PC12 cell in real-time detection [Citation141]. Due to the high conductance of CNTs, higher sensitive to the electrochemical concentration at the surface can be induced by these networking molecules.
7. Toxicity and biocompatibility of CNTs
The biocompatibility of CNTs always remains a debate because there is a substantial evidence in the literature that debates about the cytotoxicity nature of CNTs. But, there are equal number of research articles that strongly supports the biocompatibility of CNTs and CNT-based materials. One of the major worries involving CNT-based materials is its indefinite effect on personnel involved in their fabrication and handling. CNTs are foreign particulate stable materials, characterized by its bio-persistence to the tissues which may possibly cause severe health damages to surrounding organs. Thus it has become vital to calculate or estimate the biocompatibility and toxicity of CNTs in vivo before the commercialization. As CNTs are characterized as non-biodegradable, these materials when leached out can mount up in tissue system and become health hazardous when not detected at their early stages. For example, due to their high reactiveness and size, sometimes normal phagocytic defenses misses out to recognize CNTs, eventually giving access to the blood or the nervous system. CNTs also have an ability to alter the function of proteins by modifying the structure and raising the potential for auto-immune effects and this can presumably be avoided when functionalized covalently and non-covalently [Citation142–145]. There are numerous intense research studies which proved that the CNTs and their composites based biomaterials are biocompatible. The exclusive electronic properties of CNT have invited numerous studies examining the biocompatible nature of CNT for neural applications especially concentrating on neurite extension. Mattson et al. revealed that neurite branching and growth were not detected on unmodified CNT surfaces suggesting a relatively weak adhesive nature of CNTs. In the same study, functionalized CNTs when enhanced with 4-hydroxynonenal antibody encouraged neurite extension and total neurite branching was significantly improved by twofold and threefold respectively [Citation146]. In another study, McKenzie et al. explored the effect of carbon nanofiber diameter (60–200 nm) and variable surface energy on neural implant applications. It has been found that astrocytes, a glial scar tissue-forming cells preferentially adhered and proliferated on larger diameter (greater than 100 nm) and higher surface energy (125–140 mJ m−2) carbon nanofibers. Astrocytes adhesion and proliferation has been limited drastically where these cells were seeded on the composites prepared from polycarbonate urethane and carbon nanofibers. It has been found that adhesion to a polymer matrix has been limited due to the nanometer roughness [Citation147]. Interestingly, carbon nanofibers found to be compatible with physiological condition. Elias et al. for the first time investigated the applications of carbon nanofibers for orthopedic applications by studying the adhesion and proliferation of osteoblast cells on carbon nanofibers with diameters varying from 60 to 200 nm. This study showed that unmodified carbon nanofibers without any functionalization with bioactive molecules demonstrated amplified osteoblast proliferation on the nanophase with a diameter <100 nm [Citation148]. Interested readers are suggested to go through the detailed review on CNTs biocompatibility and toxicity published elsewhere [Citation149,Citation150]. Star et al. conveyed that the catalytic biodegradation of chemically modified SWCNTs can be made possible through horseradish peroxidase enzyme in the presence of H2O2 of low concentration (∼40 µM) for a possible application in drug delivery, gene silencing and tumor imaging. It has been reported that within 10 days of inducement, all the CNTs were found to be degraded [Citation151,Citation152]. Like biocompatibility, biodurability of CNTs is also noticed to be influenced by the interaction of CNT surface functionalization. It has been found that, functionalized SWCNTs were revealed to degrade inside cells (termed as cellular degradation) such as neutrophils and macrophages when dissolved in phagolysosomal simulant fluid (PSF) by the action of myeloperoxidase [Citation153]. On the contrary, Poland et al. highlighted that the possible risk of pristine, non-functionalized SWCNTs remained unaffected (no morphological variations were observed) when exposed to the identical biological oxidative conditions (HRP or PSF) while it should be noted that MWCNTs which are >20 μm could lead to the amplified risk of carcinogenesis [Citation75,Citation154]. The same studies were expanded to MWCNTs and evidenced that they degraded much slower than SWCNTs because of their concentric graphite structure. The rate at which MWCNTs degrade is directly connected to the degree of carboxylation on the CNTs surface, ultimately delivering CO2 as a final byproduct.
Although there is concrete indication that suggests that oxidative conditions can prompt degradation of CNTs, the proof of degradation involving living organisms in CNTs is still missing. It was shown by Russier et al. that biodegradation of SWCNTs can be initiated by external and internal enzymes or by biofluids that mimics phagolysosome content. It should also be noted that the existence of COOH functional groups and defects on the CNTs surface offers interaction sites for oxidative agents and for the degradation process to initiate. Also, a comprehensive shortening of the CNTs by enzymatic action could improve the dispersibility of CNTs by giving away the defect sites generated during oxidative process. Under the same processing conditions, oxidized-SWCNTs are rapidly degraded in horseradish peroxidase compared to oxidized-MWCNTs of different sizes and diameter. When nitrogen (N) is used as a doping agent to replace some of the carbon atoms to form N-MWCNTs, more defective tubes existed not only on the surface walls but also in the graphite walls. Intrinsic nitrogen-functionalized MWCNTs with more defective sites presented complete enzymatic degradation within less than 3 months [Citation155,Citation156]. Later, the biodegradability of CNTs has been characterized in more advanced studies, and possible future advances in appropriate research is expected to take up it to next level. As per current analysis, CNTs tend to biodegrade at an extreme slow rate and may even take 3 months or more to trace out completely. Therefore, it can be safely concluded from current understanding that biodegradability of CNTs has no rapid impact on the safety of CNTs unless and otherwise where SCNT fiber is used [Citation157].
The demonstration of biodegradability for f-CNTs has significant implications for distant toxicological profiles. Many aspects occurring in the fluid might be responsible for the degradation of CNTs, such as kinetics, i.e. the rate at which degradation is happening and the kinetics of body excretion of these CNTs. This concern was partially analyzed by Kagan et al. who demonstrated, when compared to unbroken CNTs, degraded CNT byproduct does not cause any inflammatory responses to the body even after appreciable time. But, the author suggested that in-depth systematic studies are much needed to analyze the effect completely [Citation158]. CNTs were tested for cancer drug delivery systems by coating with doxorubicin (Dox) drug before functionalizing chemically with a biodegradable polymer chitosan. The studies proved to be biocompatible and nontoxic in nature when tested with human breast cancer cell line and also with fibroblasts [Citation159]. In vivo studies on mice to assess acute pulmonary inflammation and systemic immune responses have been carried on asbestos, SWCNTs, and carbon nanofibers (CNF) and were determined by Murray et al. It has been reported that inflammation of above said materials can be mainly be determined by the amount of agglomeration of particles/fibers. As this in vivo valuation is being done on mice, the extent at which lung effects co related to human beings is still not fully acknowledged. But on the positive side, many studies done on mice found similar lung effects and showed almost similar human health risk assessment [Citation160]. CNTs when mixed with other metal ions such nickel (26%) or iron (17.7% to 0.2%) to form metal composites may be more cytotoxic in vitro and in vivo with possible carcinogenic effects. However, in experimental animal studies, when comparing pure CNTs and CNT composite material with a low metal content, CNTs are more associated with positive effects whereas CNT composite materials may bring more thoughtful work-related health threats in chronic inhalation exposure and may even cause serious hostile lung effects [Citation161,Citation162].
By identifying creation exposure pathways of toxins, human exposure to toxic nanoparticles can be avoided [Citation163]. As of today, there is no standardized appropriate method to determine the physicochemical nature of nanoparticles in biological systems, and it may be one of the reasons why the exact nature of nanomaterial toxicity is undetermined till date. The determination of appropriate exposure protocols such as developing a computational simulation of the nanomaterial-cell interface that directs the dynamics of nanoparticles in complex environments including those within organisms.
8. Applications of CNT-based biosensors
Applications of the CNT-based biosensors is given in , among all the types, enzyme based biosensors are the most popular type of biosensors and many commercialized biosensing devices are based on it. Vicentini et al. reported an enhanced signal response of the biosensors based on a MWCNT-ionic liquids/tyrosinase [Citation164]. This biosensor also exhibits high stability and long lifetime. In addition, glucose detection is one of the important applications of enzyme biosensors because the concentration of blood glucose is one of the important indicators of human health [Citation165]. Non-enzyme CNT-based biosensors for glucose detection also evolved by dispersing nickel and copper nanoparticles instead of enzyme on the MWCNT electrode [Citation166] can effectively analyze glucose concentration in human serum. Gougis et al. reported another kind of non-enzymatic glucose biosensor by depositing gold nanostructures onto CNT electrode with remarkable performance [Citation167].
Immobilization of enzyme on CNTs is the key step for the preparation of CNT-based enzyme biosensors. Cang-Rong and Pastorin showed in their experiments that the enzyme was physically adsorbed onto CNTs has much higher activity [Citation168]. Chen et al. reported a physically adsorbed enzyme aflatoxin-oxidase onto a chitosan-SWCNTs modified gold electrode has high sensitive for the detection of sterigmatocystin [Citation169]. However, the adhesion strength between enzyme and electrode substrate surface is weak, for addressing this issue, Yola and Atar [Citation170] developed a gold nanoparticle/p-MWCNTs nanocomposites to detect quercetin and rutin simultaneously by a new square wave voltammetry method that exhibit very good biological activity and good stability, durability.
DNA biosensors are well known for medical diagnostics, forensic science, agriculture, or even environmental clean-up efforts just after there were developed. Tang et al. have fabricated completely electronic DNA sensors based on SWCNT-FET, which are readily amenable to integration with high density sensor arrays in ‘system-on-chip’ for microanalysis devices [Citation171]. The gold nanoparticles/MWCNTs film sensor interface significantly enhanced the performance of the electrochemical DNA biosensor. This novel sensing platform exhibits excellent sensitivity and selectivity and has been utilized in human serum samples for an assay of complementary target DNA analysis [Citation172].
Immune sensor mainly used in clinical medicine. For instance, Yu et al. developed amplified electrochemical immunosensors based on SWCNT forest platforms with multi-label secondary antibody-nanotube bioconjugates for highly sensitive detection of cancer biomarkers in serum and tissue lysates [Citation173]. These SWCNT immunoassay platforms show excellent accuracy for clinical screening and point-of-care diagnostics. Faridbod et al. have developed a novel ultra-sensitive immunosensor for the detection of pesticide such as atrazine [Citation174]. This biosensor has shown low response time, high sensitivity and long-term stability.
CNT-based electrochemical biosensing techniques can be used as clinical tools to detect protein cancer biomarkers [Citation175–177]. Biosensors can also be used as platforms for monitoring food traceability, quality, safety and nutritional value [Citation178,Citation179]. However, these applications are categorized into ‘single shot’ analysis tools (cost-effective and disposable) and “long-term” monitoring analysis tools. For example electrochemical biosensors used in drug discovery [Citation180]; for the detection of a number of chemical and biological agents that are considered to be toxic materials of defence interest [Citation181]; for use in artificial implantable devices such as pacemakers [Citation182] and other prosthetic devices [Citation183] and sewage epidemiology [Citation184].
9. Conclusions
CNTs consist of versatile features that combine the potential to serve for diagnostic and therapeutic applications. While they may serve as biosensors of cancer biomarkers, they can be loaded with anticancer drugs and used as therapeutic platforms in oncology. Although, CNTs-based biosensors are promising it still has many practical concerns in applications. For example, for the fabrication of biosensors usually needs specific size and helicity, but it is very hard to control size of CNTs while manufacturing. It is also very difficult to make cost-effective and high purity in mass production of CNTs, that is the reason why the current market prices of CNTs is too high for any realistic commercial applications. In CNT-based biosensors, enzyme always needs to immobilize onto surface of CNTs. However, immobilization may damage their biological activity, biocompatibility and structure stability and need to perform their cytotoxicity. As it stated that, the structural stabilization and surface characteristics of CNTs are need to be standardized for the cytotoxicity determination. The continuous CNT fibers avoid the leaching of CNT, implantable electrodes for in vivo testing. All the above-mentioned issues must be evaluated by a scientific and systematic methods. It is clear that much further advancement needed to be addressed before CNTs technology can be applied in carcinogenic treatments. The development of CNT-based biosensors have many different aspects which needs the co-operation between materials scientists, and engineers, who fabricates the new devices such as biosensors.
Acknowledgment
All the authors thank LRF (Lloyd's Register Foundation, United Kingdom) for the financial support.
Disclosure Statement
No potential conflict of interest was reported by the author(s).
References
- Cremer M. About the cause of the electromotive properties of the tissue, a contribution to the teaching of polyphasic electrolyte chains. Z Biol. 1906;47:562–608.
- Clark LC. Monitor and control of blood and tissue oxygenation. Trans Am Soc Artif Intern Organs. 1956;2:41–48.
- Guilbault GG, Montalvo JG Jr. A urea-specific enzyme electrode. J Am Chem Soc. 1969;91(8):2164–2165.
- Musameh M, Wang J, Merkoci A, et al. Low-potential stable NADH detection at carbon-nanotube-modified glassy carbon electrodes. Electrochem Commun. 2002:4(10):743–746.
- Allen BL, Kichambare PD, Star A. Carbon nanotube field-effect-transistor based biosensors. Adv Mater. 2007;19(11):1439–1451.
- Meng L, Wu P, Chen G, et al. Low potential detection of glutamate based on the electrocatalytic oxidation of NADH atthionine/singlewalled carbon nanotubes composite modified electrode. Biosens Bioelectron. 2009;24(6):1751–1756.
- Li SC, Chen JH, Cao H, et al. Amperometric biosensor for aflatoxin B1 based on aflatoxin-oxidase immobilized on multiwalled carbon nanotubes. Food Control. 2011;22(1):43–49.
- Liang B, Li L, Tang X, et al. Microbial surface display of glucose dehydrogenase for amperometric glucose biosensor. Biosens. Bioelectron. 2013;45:19–24.
- Ramon-Marquez T, Medina-Castillo AL, Nagiah N, et al. A multifunctional material based on co-electrospinning for developing biosensors with optical oxygen transduction. Anal Chim Acta. 2018;1015:66–73.
- Iijima S. Helical microtubules of graphitic carbon. Nature. 1991;354:56–58.
- Harris PJF. Carbon nanotube science-synthesis, properties and applications. Cambridge (UK): Cambridge University Press; 2009.
- De Volder MF, Tawfick SH, Baughman RH, et al. Carbon nanotubes: present and future commercial applications. Science. 2013;339:535–539.
- Peng B, Locascio M, Zapol P, et al. Measurements of near-ultimate strength for multiwalled carbon nanotubes and irradiation-induced crosslinking improvements. Nat Nanotechnol. 2008;3(10):626–631.
- Wei BQ, Vajtai R, Ajayan PM. Reliability and current carrying capacity of carbon nanotubes. Appl Phys Lett. 2001;79(8):1172–1174.
- Pop E, Mann D, Wang Q, et al. Thermal conductance of an individual single-wall carbon nanotube above room temperature. Nano Lett. 2006;6(1):96–100.
- Wang J. Carbon-nanotube based electrochemical biosensors: a review. Electroanalysis. 2005;17(1):7–14.
- Yun Y, Dong Z, Shanov V, et al. Nanotube electrodes and biosensors. Nano Today. 2007;2(6):30–37.
- Thirumalraj B, Kubendhiran S, Chen S-M, et al. Highly sensitive electrochemical detection of palmatine using a biocompatible multiwalled carbon nanotube/poly-l-lysine composite. J Colloid Interface Sci. 2017;498:144–152.
- Vardharajula S, Ali SZ, Tiwari PM, et al. Functionalized carbon nanotubes: biomedical applications. Int J Nanomed. 2012;7:5361–5374.
- Bianco A, Kostarelos K, Partidos CD, et al. Biomedical applications of functionalised carbon nanotubes. Chem Commun (Camb.). 2005;5:571–577.
- Kam NW, Liu Z, Dai H. Functionalization of carbon nanotubes via cleavable disulfide bonds for efficient intracellular delivery of siRNA and potent gene silencing. J Am Chem Soc. 2005;127(36):12492–12493.
- Colvin VL. The potential environmental impact of engineered nanomaterials. Nat Biotechnol. 2003;21(10):1166–1170.
- Erdely A, Dahm M, Chen BT, et al. Carbon nanotube dosimetry: from workplace exposure assessment to inhalation toxicology. Part Fibre Toxicol. 2013;10(1):53.
- Pastorin G, Kostarelos K, Prato M, et al. Functionalized carbon nanotubes: towards the delivery of therapeutic molecules. J Biomed Nanotechnol. 2005;1(2):133–142.
- Galano A. Carbon nanotubes: promising agents against free radicals. Nanoscale. 2010;2(3):373–380.
- Kim J, Park G, Lee S, et al. Single wall carbon nanotube electrode system capable of quantitative detection of CD4+ T cells. Biosens Bioelectron. 2017;90:238–244.
- Eguílaz M, Gutierrez F, González-Domínguez JM, et al. Single-walled carbon nanotubes covalently functionalized with polytyrosine: a new material for the development of NADH-based biosensors. Biosens Bioelectron. 2016;86:308–314.
- Burrsa SL, Bhargava M, Sidhu R, et al. A paper based graphene-nanocauliflower hybrid composite for point of care biosensing. Biosens Bioelectron. 2016;85:479–487.
- Magner E. Trends in electrochemical biosensors. Analyst. 1998;123(10):1967–1970.
- Boussema F, Gross AJ, Hmida F, et al. Dawson-type polyoxometalate nanoclusters confined in a carbon nanotube matrix as efficient redox mediators for enzymatic glucose biofuel cell anodes and glucose biosensors. Biosens Bioelectron. 2018;109:20–26.
- Zhu L, Xu L, Tan L, et al. Direct electrochemistry of cholesterol oxidase immobilized on gold nanoparticles-decorated multiwalled carbon nanotubes and cholesterol sensing. Talanta. 2013;106:192–199.
- Hu F, Chen S, Wang C, et al. Multi-wall carbon nanotube-polyaniline biosensor based on lectin--carbohydrate affinity for ultrasensitive detection of Con A. Biosens Bioelectron. 2012;34(1):202–207.
- Pavlidis IV, Tsoufis T, Enotiadis A, et al. Functionalized multi-wall carbon nanotubes for lipase immobilization. Adv Eng Mater. 2010;12(5):B179–B183.
- Moyo M, Okonkwo JO, Agyei NM. An amperometric biosensor based on horseradish peroxidase immobilized onto maize tassel-multi-walled carbon nanotubes modified glassy carbon electrode for determination of heavy metal ions in aqueous solution. Enzyme Microb Technol. 2014;56:28–34.
- Koskun Y, Şavk A, Şen B, et al. Highly sensitive glucose sensor based on monodisperse palladium nickel/activated carbon nanocomposites. Anal Chim Acta. 2018;1010:37–43.
- Punetha VD, Rana S, Yoo HJ, et al. Functionalization of carbon nanomaterials for advanced polymer nanocomposites: a comparison study between CNT and graphene. Prog Polym Sci. 2017;67:1–47.
- Zhu Z. An overview of carbon nanotubes and graphene for biosensing applications. Nano-Micro Lett. 2017:9(3):25.
- Di Crescenzo A, Ettorre V, Fontana A. . Non-covalent and reversible functionalization of carbon nanotubes. Beilstein J Nanotechnol. 2014;5:1675–1690.
- Mananghaya MR, Santos GN, Yu DN. Solubility of amide functionalized single wall carbon nanotubes: a quantum mechanical study. J Mol Liquids. 2017;242(Supplement C):1208–1214.
- Girardi FA, Bruch GE, Peixoto CS, et al. Toxicity of single-wall carbon nanotubes functionalized with polyethylene glycol in zebrafish (Danio rerio) embryos. J Appl Toxicol. 2017;37(2):214–221.
- Mittal V. Carbon nanotubes surface modifications: an overview. In: Mittal V, editor. Surface modification of nanotube fillers. Vol. 1. Wiley Interscience; 2011, p. 316.
- Antonucci A, Kupis-Rozmysłowicz J, Boghossian AA. Noncovalent protein and peptide functionalization of single-walled carbon nanotubes for biodelivery and optical sensing applications. ACS Appl Mater Interfaces. 2017;9(13):11321–11331.
- Bai L, Bai Y, Zheng J. Improving the filler dispersion and performance of silicone rubber/multi-walled carbon nanotube composites by noncovalent functionalization of polymethylphenylsiloxane. J Mater Sci. 2017;52(12):7516–7529.
- O'Connell MJ, Bachilo SM, Huffman CB, et al. Band gap fluorescence from individual single-walled carbon nanotubes. Science. 2002;297(5581):593–596.
- Moore VC, Strano MS, Haroz EH, et al. Individually suspended single-walled carbon nanotubes in various surfactants. Nano Lett. 2003;3(10):1379–1382.
- Holzinger M, Baur J, Haddad R, et al. Multiple functionalization of single-walled carbon nanotubes by dip coating. Chem Commun (Camb.). 2011;47(8):2450–2452.
- Tu X, Zheng M. A DNA-based approach to the carbon nanotube sorting problem. Nano Res. 2008;1:185–194.
- Kostarelos K. Cellular uptake of functionalized carbon nanotubes is in-dependent of functional group and cell type. Nat Nanotechnol. 2007;2(2):108–113.
- Liu Z, Sun X, Nakayama-Ratchford N, et al. Supramolecular chemistry on water-soluble carbon nanotubes for drug loading and delivery. ACS Nano. 2007;1(1):50–56.
- Liu Z, Robinson JT, Sun X, et al. PEGylated nanographene oxide for delivery of water-insoluble cancer drugs. J Am Chem Soc. 2008;130(33):10876–10877.
- Liu Y, Wu DC, Zhang WD, et al. Polyethylenimine-grafted multiwalled carbon nanotubes for secure noncovalent immobilization and efficient delivery of DNA. Angew Chem. 2005;44(30):4782–4785.
- Sanz V, Borowiak E, Lukanov P, et al. Optimising DNA binding to carbon nanotubes by non-covalent methods. Carbon. 2011;49(5):1775–1781.
- Setaro A, Adeli M, Glaeske M, et al. Preserving π-conjugation in covalently functionalized carbon nanotubes for optoelectronic applications. Nat Commun. 2017;8:14281.
- Harris JM, Zalipsky S. Poly(ethylene glycol) chemistry and biological applications. ACS Symposium Series, 213th National Meeting of the American Chemical Society, San Francisco, CA, April 13–17, 1997. Washington, DC: American Chemical Society.
- Ostuni E, Chapman RG, Holmlin RE, et al. A survey of structure–property relationships of surfaces that resist the adsorption of protein. Langmuir. 2001;17(18):5605–5620.
- Liu Y-T, Zhao W, Huang Z-Y, et al. Noncovalent surface modification of carbon nanotubes for solubility in organic solvents. Carbon. 2006;44:1613–1616.
- Kang YK, Lee O-S, Deria P, et al. Helical wrapping of single-walled carbon nanotubes by water soluble poly(p-phenylene ethynylene). Nano Lett. 2009;9(4):1414–1418.
- Zheng M, Jagota A, Semke ED, et al. DNA-assisted dispersion and separation of carbon nanotubes. Nat Mater. 2003;2(5):338–342.
- Zhong Q, Diev VV, Roberts ST, et al. Fused porphyrin-single-walled carbon nanotube hybrids: efficient formation and photophysical characterization. ACS Nano. 2013;7(4):3466–3475.
- Journet C, Master WK, Bernier P, et al. Large-scale production of single-walled carbon nanotubes by the electric-arc technique. Nature. 1997;388:756–758.
- Star A, Steuerman DW, Heath JR, et al. Starched carbon nanotubes. Angew Chem. 2002;41(14):2508–2512.
- Bandyopadhyaya R, Nativ-Roth E, Regev O, et al. Stabilization of individual carbon nanotubes in aqueous solutions. Nano Lett. 2002;2(1):25–28.
- Yang Z, Chen X, Chen C, et al. Noncovalent-wrapped sidewall functionalization of multiwalled carbon nanotubes with polyimide. Polym Compos. 2007;28(1):36–41.
- Sireesha M, Babu VJ, Ramakrishna S. Functionalized carbon nanotubes in bio-world: applications, limitations and future directions. Mater Sci Eng B-Adv. 2017;223:43–63.
- Fu Q, Lu C, Liu J. Selective coating of single wall carbon nanotubes with thin SiO2 layer. Nano Lett. 2002;2(4):329–332.
- Welsher K, Liu Z, Daranciang D, et al. Selective probing and imaging of cells with single walled carbon nanotubes as near-infrared fluorescent molecules. Nano Lett. 2008;8(2):586–590.
- Pan J, Li F, Choi JH. Single-walled carbon nanotubes as optical probes for bio-sensing and imaging. J Mater Chem B. 2017;5(32):6511–6522.
- Liu Z, Tabakman S, Sherlock S, et al., Multiplexed five-color molecular imaging of cancer cells and tumor tissues with carbon nanotube raman tags in the near-infrared. Nano Res. 2010;3(3):222–233.
- Mundra RV, Wu X, Sauer J, et al. Nanotubes in biological applications. Curr Opin Biotechnol. 2014;28:25–32.
- Peigney A, Laurent C, Flahaut E, et al. Specific surface area of carbon nanotubes and bundles of carbon nanotubes. Carbon. 2001;39(4):507–514.
- Kam NWS, O’connell M, Wisdom JA, et al. Carbon nanotubes as multifunctional biological transporters and near-infrared agents for selective cancer cell destruction. Proc Natl Acad Sci USA. 2005;102:11600–11605.
- Chakravarty P, Marches R, Zimmerman NS, et al. Thermal ablation of tumor cells with antibodyfunctionalized single-walled carbon nanotubes. Proc Natl Acad Sci USA. 2008;105:8697–8702.
- Holzinger M, Le Goff A, Cosnier S. Nanomaterials for biosensing applications: a review. Front Chem. 2014;2:63.
- Sayes CM, Liang F, Hudson JL, et al. Functionalization density dependence of single-walled carbon nanotubes cytotoxicity in vitro. Toxicol Lett. 2006;161:135–142.
- Bianco A, Kostarelos K, Prato M. Making carbon nanotubes biocompatible and biodegradable. Chem. Commun. 2011;47(37):10182–10188.
- Portney NG, Ozkan M. Nano-oncology: drug delivery, imaging, and sensing. Anal Bioanal Chem. 2006;384(3):620–630.
- Choi YE, Kwak JW, Park JW. Nanotechnology for early cancer detection. Sensors (Basel). 2010;10(1):428–455.
- Wang Z, Dai Z. Carbon nanomaterial-based electrochemical biosensors: an overview. Nanoscale. 2015;7(15):6420–6431.
- Veetil JV, Ye K. Development of immunosensors using carbon nanotubes. Biotechnol Prog. 2007;23:517–531.
- Jain A, Homayoun A, Bannister CW, et al. Single-walled carbon nanotubes as near-infrared optical biosensors for life sciences and biomedicine. Biotechnol J. 2015;10(3):447–459.
- Tothill IE. Biosensors for cancer markers diagnosis. Semin Cell Dev Biol. 2009;20:55–62.
- Kurbanoglu S, Ozkan SA, Merkoçi A. Nanomaterials-based enzyme electrochemical biosensors operating through inhibition for biosensing applications. Biosens Bioelectron. 2017;89(Part 2):886–898.
- Cai C, Chen J. Direct electron transfer of glucose oxidase promoted by carbon nanotubes. Anal Biochem. 2004;332(1):75–83.
- Lee YM, Kwon OY, Yoon YJ, et al. Immobilization of horseradish peroxidase on multi-wall carbon nanotubes and its electrochemical properties. Biotechnol Lett. 2006;28(1):39–43.
- Rubianes MD, Rivas GA. Enzymatic biosensors based on carbon nanotubes paste electrodes. Electroanalysis. 2005;17(1):73–78.
- Ruhal A, Rana JS, Kumar S, et al. Immobilization of malate dehydrogenase on carbon nanotubes for development of malate biosensor. Cell Mol Biol. 2012;58(1):15–20.
- Zappi D, Caminiti R, Ingo GM, et al. Biologically friendly room temperature ionic liquids and nanomaterials for the development of innovative enzymatic biosensors. Talanta. 2017;175:566–572.
- Li J, Liu Q, Liu Y, et al. DNA biosensor based on chitosan film doped with carbon nanotubes. Anal Biochem. 2005;346(1):107–114.
- Cai H, Cao X, Jiang Y, et al. Carbon nanotube-enhanced electrochemical DNA biosensor for DNA hybridization detection. Anal Bioanal Chem. 2003;375(2):287–293.
- Brrito PJ, Santhanam KSV, Ajayan PM. Carbon nanotube electrode for oxidation of dopamine. Biochem Bioenerg. 1996;41(1):121–125.
- Zhu Z, Wang J, Munir A, et al. Direct electrochemistry and electrocatalysis of horseradish peroxidase immobilized on bamboo shaped carbon nanotubes/chitosan matrix. Colloids Surf A. 2011;385(1–3):91–94.
- Hrapovic S, Liu Y, Male KB, et al. Electrochemical biosensing platforms using platinum nanoparticles and carbon nanotubes. Anal Chem. 2004;76(4):1083–1088.
- Wu X, Zhao B, Wu P, et al. Effects of ionic liquids on enzymatic catalysis of the glucose oxidase toward the oxidation of glucose. J Phys Chem B. 2009;113(40):13365–13373.
- Zhu Z, Garcia-Gancedo L, Flewitt AJ, et al. A critical review of glucose biosensors based on carbon nanomaterials: carbon nanotubes and graphene. Sensors (Basel). 2012;12(5):5996–6022.
- Yu X, Chattopadhyay D, Galeska I, et al. Peroxidase activity of enzymes bound to the ends of single-wall carbon nanotube forest electrodes. Electrochem Commun. 2003;5(5):408–411.
- Wang SG, Zhang Q, Wang R, et al. Multi-walled carbon nanotubes for the immobilization of enzyme in glucose biosensors. Electrochem Commun. 2003;5(9):800–803.
- Roy S, Vedala H, Choi W. Vertically aligned carbon nanotube probes for monitoring blood cholesterol. Nanotechnology. 2006;17(4):S14–S18.
- Zhang J, Boghossian AA, Barone PW, et al. Single molecule detection of nitric oxide enabled by d(AT)15 DNA adsorbed to near infrared fluorescent single-walled carbon nanotubes. J Am Chem Soc. 2011;133:567–581.
- Lin Y, Lu F, Tu Y, et al. Glucose biosensors based on carbon nanotube nanoelectrode ensembles. Nano Lett. 2004;4:191–195.
- Patolsky F, Weizmann Y, Willner I. Long-range electrical contacting of redox enzymes by SWCNTconnectors. Angew Chem Int Ed. 2004;43(16):2113–2117.
- Chen Z, Tabakman SM, Goodwin AP, et al. Protein microarrays with carbon nanotubes as multicolor Raman labels. Nat Biotechnol. 2008;26(11):1285–1292.
- Vilian ATE, Madhu R, Chen S-M, et al. Facile synthesis of MnO2/carbon nanotubes decorated with a nanocomposite of Pt nanoparticles as a new platform for the electrochemical detection of catechin in red wine and green tea samples. J Mater Chem B. 2015;3:6285–6292.
- Xiao-Ming Ma, Mi Sun, Yue Lin, et al. Progress of visual biosensor based on gold nanoparticles. J Anal Chem. 2018;46(1):1–10.
- Campuzano S, Yáñez-Sedeño P, Pingarrón JM. Diagnostics strategies with electrochemical affinity biosensors using carbon nanomaterials as electrode modifiers. Diagnostics. 2017;7(2):1–26.
- Zhu G, Sun H, Zou B, et al. Electrochemical sensing of 4-nitrochlorobenzene based on carbon nanohorns/graphene oxide nanohybrids. Biosens Bioelectron. 2018;106:136–141.
- Lawal AT. Progress in utilisation of graphene for electrochemical biosensors. Biosens Bioelectron. 2018;106:149–178.
- Sun H, Ren J, Qu X. Carbon nanomaterials and DNA: from molecular recognition to applications. Acc Chem Res. 2016;49(3):461–470.
- Niu S, Zhao M, Ren R, et al. Carbon nanotube-enhanced DNA biosensor for DNA hybridization detection using manganese(II)-Schiff base complex as hybridization indicator. J Inorg Biochem. 2009;103(1):43–49.
- He P, Dai L. Aligned carbon nanotube-DNA electrochemical sensors. Chem Commun (Camb.). 2004;3:348–349.
- Li J, Ng HT, Cassell A, et al. Carbon nanotube nanoelectrode array for ultrasensitive DNA detection. Nano Lett. 2003;3(5):597–602.
- Xu Y, Ye X, Yang L, et al. Impedance DNA biosensor using electropolymerized polypyrrole/multiwalled carbon nanotubes modified electrode. Electroanalysis. 2006;18(15):1471–1478.
- Weber JE, Pillai S, Ram MK, et al. Electrochemical impedance-based DNA sensor using a modified single walled carbon nanotube electrode. Mater Sci Eng C. 2011;31(5):821–825.
- Guo K, Wang Y, Chen H, et al. An aptamer–SWNT biosensor for sensitive detection of protein via mediated signal transduction. Electrochem Commun. 2011;13(7):707–710.
- Rezaei B, Shoushtari AM, Rabiee M, et al. An electrochemical immunosensor for cardiac Troponin I using electrospun carboxylated multi-walled carbon nanotube-whiskered nanofibres. Talanta. 2018;182:178–186.
- Riberi WI, Tarditto LV, Zon MA, et al. Development of an electrochemical immunosensor to determine zearalenone in maize using carbon screen printed electrodes modified with multi-walled carbon nanotubes/polyethyleneimine dispersions. Sens Actuators B. 2018;254:1271–1277.
- Aziz MA, Park S, Jon S, et al. Amperometric immunosensing using an indium tin oxide electrode modified with multi-walled carbon nanotube and poly(ethylene glycol)-silane copolymer. Chem Commun. 2007;25:2610–2612.
- Gomes-Filho SLR, Dias ACMS, Silva MMS, et al. A carbon nanotube-based electrochemical immunosensor for cardiac troponin. Microchem J. 2013;109:10–15.
- Malhotra R, Patel V, Vaqué JP, et al. Ultrasensitive electrochemical immunosensor for oral cancer biomarker IL-6 using carbon nanotube forest electrodes and multilabel amplification. Anal Chem. 2010;82(8):3118–3123.
- Wan Y, Deng W, Su Y, et al. Carbon nanotube-based ultrasensitive multiplexing electrochemical immunosensor for cancer biomarkers. Biosens Bioelectron. 2011;30(1):93–99.
- Arkan E, Saber R, Karimi Z, et al. A novel antibody-antigen based impedimetric immunosensor for low level detection of HER2 in serum samples of breast cancer patients via modification of a gold nanoparticles decorated multiwall carbon nanotube-ionic liquid electrode. Anal Chim Acta. 2015;874:66–74.
- Munge BS, Fisher J, Millord LN, et al. Sensitive electrochemical immunosensor for matrix metalloproteinase-3 based on single-wall carbon nanotubes. Analyst. 2010;135(6):1345–1350.
- Hafaiedh I, Ameur S, Abdelghani A. Impedance spectroscopy of supported multiwalled carbon nanotubes for immunosensor applications. Nanomed Nanotechnol. 2012;6(3):271–275.
- Fei S, Chen J, Yao S, et al. Electrochemical behavior of l-cysteine and its detection at carbon nanotube electrode modified with platinum. Anal Biochem. 2005;339:29–35.
- Yuichi T, Kenzo M, Kazuhiko M, et al. Microfluidic and label-free multi-immunosensors based on carbon nanotube microelectrodes. Jpn J Appl Phys. 2009;48:06FJ02.
- Feng QM, Pan JB, Zhang HR, et al. Disposable paper-based bipolar electrode for sensitive electrochemiluminescence detection of a cancer biomarker. Chem Commun (Camb.). 2004;50:10949–10951.
- Baj-Rossi C, De Micheli G, Carrara S. Electrochemical detection of anti-breast-cancer agents in human serum by cytochrome P450-coated carbon nanotubes. Sensors (Basel). 2012;12(5):6520–6537.
- Ovádeková R, Jantová S, Letasiová S, et al. Nanostructured electrochemical DNA biosensors for detection of the effect of berberine on DNA from cancer cells. Anal Bioanal Chem. 2006;386(7–8):2055–2062.
- Liu FL, Xiao P, Fang HL, et al. Singlewalled carbon nanotube-based biosensors for the detection of volatile organic compounds of lung cancer. Physica E Low Dimens Syst Nanostruct. 2011;44:367–372.
- Shobha BN, Muniraj NJR. Design, modeling and performace analysis of carbon nnaotube with DNS strans as biosensosrs for postate cancer. Microsyst Technol. 2015;21:791–800.
- Smith AM, Mancini MC, Nie S. Bioimaging: second window for in vivo imaging. Nat Nanotechnol. 2009;4(11):710–711.
- Kim JH, Ahn JH, Barone PW, et al. A luciferase/single-walled carbon nanotube conjugate for near-infrared fluorescent detection of cellular ATP. Angew Chem. 2010;49(8):1456–1459.
- Heller DA, Jin H, Martinez BM, et al. Multimodal optical sensing and analyte specificity using single-walled carbon nanotubes. Nat Nanotechnol. 2009;4(2):114–120.
- Heller DA, Baik S, Eurell TE, et al. Single-walled carbon nanotube spectroscopy in live cells: towards long-term labels and optical sensors. Adv Mater. 2005;17(23):2793–2799.
- Bachilo SM, Strano MS, Kittrell C, et al. Structure-assigned optical spectra of single-walled carbon nanotubes. Science. 2002;298(5602):2361–2366.
- Tran T-T, Mulchandani A. Carbon nanotubes and graphene nano field-effect transistor-based biosensors. Trends Anal Chem. 2016;79: 222–232.
- Cao Q, Tersoff J, Farmer DB, et al. Carbon nanotube transistors scaled to a 40-nanometer footprint. Science. 2017;356(6345):1369–1372.
- Park RS, Hills G, Sohn J, et al. Hysteresis-free carbon nanotube field-effect transistors. ACS Nano. 2017;11(5):4785–4791.
- Besteman K, Lee JO, Wiertz FGM, et al. Enzyme-coated carbon nanotubes as single-molecule biosensors. Nano Lett. 2003;3(6):727–730.
- Martinez MT, Tseng YC, Ormategui N, et al. Label-free DNA biosensors based on functionalized carbon nanotube field effect transistors. Nano Lett. 2009;9(2):530–536.
- Oh J, Yoo G, Chang YW, et al. A carbon nanotube metal semiconductor field effect transistor-based biosensor for detection of amyloid-beta in human serum. Biosens Bioelectron. 2013;50:345–350.
- Sudibya HG, Ma J, Dong X, et al. Interfacing glycosylated carbon-nanotube-network devices with living cells to detect dynamic secretion of biomolecules. Angew Chem. 2009;48(15):2723–2726.
- Firme CP III, Bandaru PR. Toxicity issues in the application of carbon nanotubes to biological systems. Nanomed: Nanotechnol Biol Med. 2009;6(2):245–256.
- Zhao X, Liu R. Recent progress and perspectives on the toxicity of carbon nanotubes at organism, organ, cell, and biomacromolecule levels. Environ Int. 2012;40:244–255.
- Qi W, Tian L, An W, et al. Curing the toxicity of multi-walled carbon nanotubes through native small-molecule drugs. Sci Rep. 2017;7(1):2815.
- Ahmadi H, Ramezani M, Yazdian-Robati R, et al. Acute toxicity of functionalized single wall carbon nanotubes: a biochemical, histopathologic and proteomics approach. Chem-Biol Interact. 2017;275:196–209.
- Mattson MP, Haddon RC, Rao AM. Molecular functionalization of carbon nanotubes and use as substrates for neuronal growth. J Mol Neurosci. 2000;14(3):175–182.
- McKenzie JL, Waid MC, Shi R, et al. Decreased functions of astrocytes on carbon nanofibre materials. Biomaterials. 2004;25(7–8):1309–1317.
- Elias KL, Price RL, Webster TJ. Enhanced functions of osteoblasts on nanometer diameter carbon fibers. Biomaterials. 2002;23(15):3279–3287.
- Smart SK, Cassady AI, Lu GQ, et al. The biocompatibility of carbon nanotubes. Carbon. 2006;44:1034–1047.
- Kumar S, Rani R, Dilbaghi N, et al. Carbon nanotubes: a novel material for multifaceted applications in human healthcare. Chem Soc Rev. 2017;46(1):158–196.
- Allen BL, Kotchey GP, Chen Y, et al. Mechanistic investigations of horseradish peroxidase-catalyzed degradation of single-walled carbon nanotubes. J Am Chem Soc. 2009;131(47):17194–17205.
- Allen BL, Kichambare PD, Gou P, et al. Biodegradation of single-walled carbon nanotubes through enzymatic catalysis. Nano Lett. 2008;8:3899–3903.
- Kagan VE, Konduru NV, Feng W, et al. Carbon nanotubes degraded by neutrophil myeloperoxidase induce less pulmonary inflammation. Nat Nano. 2010;5(5):354–359.
- Poland CA, Duffin R, Kinloch I, et al. Carbon nanotubes introduced into the abdominal cavity of mice show asbestos-like pathogenicity in a pilot study. Nat Nano. 2008;3(7):423–428.
- Russier J, Ménard-Moyon C, Venturelli E, et al. Oxidative biodegradation of single- and multi-walled carbon nanotubes. Nanoscale. 2011;3(3):893–896.
- Zhao Y, Allen BL, Star A. Enzymatic degradation of multiwalled carbon nanotubes. J Phys Chem A. 2011;115(34):9536–9544.
- Saito N, Haniu H, Usui Y, et al. Safe clinical use of carbon nanotubes as innovative biomaterials. Chem Rev. 2014;114:6040–6079.
- Kagan VE, Shi J, Feng W, et al. Fantastic voyage and opportunities of engineered nanomaterials: what are the potential risks of occupational exposures? J Occup Environ Med. 2010;52(9):943–946.
- Mezni A, Saber NB, Alhadhrami, AA, et al. Highly biocompatible carbon nanocapsules derived from plastic waste for advanced cancer therapy. J Drug Deliv Sci Technol. 2017;41:351–358.
- Murray AR, Kisin ER, Tkach AV, et al. Factoring-in agglomeration of carbon nanotubes and nanofibers for better prediction of their toxicity versus asbestos. Part Fibre Toxicol. 2012;9(10):1–19.
- Lam CW, James JT, McCluskey R, et al. Pulmonary toxicity of single-wall carbon nanotubes in mice 7 and 90 days after intratracheal instillation. Toxicol Sci. 2004;77(1):126–134.
- Shvedova AA, Castranova V, Kisin ER, et al. Exposure to carbon nanotube material: assessment of the biological effects of nanotube materials using human keratinocyte cells. J Toxicol Environ Health A. 2003;66(20):1901–1918.
- Buzea C, Blandino IIP, Robbie K. Nanomaterials and nanoparticles: sources and toxicity. Biointerphases. 2007;2(4):MR17–MR172.
- Vicentini FC, Janegitz BC, Brett CMA, et al. Tyrosinase biosensor based on a glassy carbon electrode modified with multi-walled carbon nanotubes and 1-butyl-3-methylimidazolium chloride within a dihexadecylphosphate film. Sens Actuators B. 2013;188:1101–1108.
- Pourasl AH, Ahmadi MT, Rahmani M, et al. Analytical modeling of glucose biosensors based on carbon nanotubes. Nanoscale Res Lett. 2014;9(1):1–7.
- Lin K-C, Lin Y-C, Chen S-M. A highly sensitive nonenzymatic glucose sensor based on multi-walled carbon nanotubes decorated with nickel and copper nanoparticles. Electrochim Acta. 2013;96:164–172.
- Gougis M, Tabet-Aoul A, Ma D, et al. Laser synthesis and tailor-design of nanosized gold onto carbon nanotubes for non-enzymatic electrochemical glucose sensor. Sens Actuators B. 2017;193:363–369.
- Jason Teng C-R, Giorgia P. The influence of carbon nanotubes on enzyme activity and structure: investigation of different immobilization procedures through enzyme kinetics and circular dichroism studies. Nanotechnology. 2009;20(25):255102.
- Chen J, Liu D, Li S, et al. Development of an amperometric enzyme electrode biosensor for sterigmatocystin detection. Enzyme Microb Technol. 2010;47(4):119–126.
- Yola ML, Atar N. A novel voltammetric sensor based on gold nanoparticles involved in p-aminothiophenol functionalized multi-walled carbon nanotubes: application to the simultaneous determination of quercetin and rutin. Electrochim Acta. 2014;119:24–31.
- Tang XW, Bansaruntip S, Nakayama N, et al. Carbon nanotube DNA sensor and sensing mechanism. Nano Lett. 2006;6(8):1632–1636.
- Dong X, Lu X, Zhang K, et al. Chronocoulometric DNA biosensor based on a glassy carbon electrode modified with gold nanoparticles poly(dopamine) and carbon nanotubes. Microchim Acta. 2012;180(1–2):101–108.
- Yu X, Munge B, Patel V, et al. Carbon nanotube amplification strategies for highly sensitive immunodetection of cancer biomarkers. J Am Chem Soc. 2006;128(34): 11199–11205.
- Norouzi P, Larijani B, Ganjali MR, et al. Int J Electrochem Sci. 2012;7(11)10414–10426.
- Jolly P, Formisano N, Estrela P. DNA aptamer-based detection of prostate cancer. Chem Pap. 2015;69:77–89.
- Bhalla N, Jolly P, Formisano N, et al. Introduction to biosensors. Essays Biochem. 2016;60(1):1–8.
- Formisano N, Jolly P, lBhalla N, et al. Optimisation of an electrochemical impedance spectroscopy aptasensor by exploiting quartz crystal microbalance with dissipation signals. Sens Actuators B. 2015;220:369–375.
- Sharma TK, Ramanathan R, Rakwal R, et al. Moving forward in plant food safety and security through nanobiosensors: adopt or adapt biomedical technologies? Proteomics. 2015;15:1680–1692.
- Dorst BV, Mehta J, Bekaert K, et al. Recent advances in recognition elements of food and environmental biosensors: a review. Biosens Bioelectron. 2010;26:1178–1194.
- Bhalla N, Lorenzo MD, Pula G, et al. Protein phosphorylation detection using dual-mode field-effect devices and nanoplasmonic sensors. Sci Rep. 2015;5:8687.
- Paddle BM. Biosensors for chemical and biological agents of defence interest. Biosens Bioelectron. 1996;11:1079–1113.
- Grayson ACR, Shawgo RS, Johnson AM, et al. A BioMEMS review: MEMS technology for physiologically integrated devices. Proc IEEE. 2004;92:6–21.
- Parker KK, O'Grady M. Porous electroactive hydrogels and uses thereof. 8,999,378 B2. U.S. Pat. 2015, 2015.
- Yang Z, k-Hordern BK, Frost CG, et al. Community sewage sensors for monitoring public health. Environ Sci Technol. 2015;49:5845–5846.
- Ajayan PM, Tour JM. Materials science: nanotube composites. Nature. 2007;447(7148):1066–1068.
- Adeli M, Soleyman R, Beiranvanda Z, et al. Carbon nanotubes in cancer therapy: a more precise look at the role of carbon nanotube-polymer interactions. Chem Soc Rev. 2013;42(12):5231–5256.
- Tîlmaciu C-M, Morris MC. Carbon nanotube biosensors. Front Chem. 2015;3:59.
- Li G, Liao JM, Hu GQ, et al. Study of carbon nanotube modified biosensor for monitoring total cholesterol in blood. Biosens Bioelectron. 2005;20:2140–2144.
- Santos RM, Rodrigues MS, Laranjinha J, et al. Biomimetic sensor based on hemin/carbon nanotubes/chitosan modified microelectrode for nitric oxide measurement in the brain. Biosens Bioelectron. 2013;44:152–159.
- Prasad BB, Prasad A, Tiwari MP, et al. Multiwalled carbon nanotubes bearing ‘terminal monomeric unit’ for the fabrication of epinephrine imprinted polymer-based electrochemical sensor. Biosens Bioelectron. 2013;45:114–122.
- Kress GJ, Shu HJ, Yu A, et al. Fast phasic release properties of dopamine studied with a channel biosensor. J Neurosci. 2014;34(35):11792–11802.
- Jin H, Heller DA, Kalbacova M, et al. Detection of single-molecule H2O2 signalling from epidermal growth factor receptor using fluorescent single-walled carbon nanotubes. Nat Nanotechnol. 2010;5(4):302–309.
- Park YK, Bold B, Lee WK, et al. D-(+)-galactose-conjugated single-walled carbon nanotubes as new chemical probes for electrochemical biosensors for the cancer marker galectin-3. Int J Mol Sci. 2011;12:2946–2957.
- Zheng TT, Zhang R, Zou L, et al. A label-free cytosensor for the enhanced electrochemical detection of cancer cells using polydopamine-coated carbon nanotubes. Analyst. 2012;137:1316–1318.
- Zhang Y, Gao G, Liu H, et al. Identification of volatile biomarkers of gastric cancer cells and ultrasensitive electrochemical detection based on sensing interface of Au-Ag alloy coated MWCNTs. Theranostics. 2014;4(2):154–162.