Abstract
In order to improve the antimicrobial activity of Ag nanomaterial and minimum their toxicity, cinnamic acid has been utilized as both reducing agent and protecting ligand to synthesis of silver (Ag) nanoparticles (NPs). The synthesized cinnamic acid (CA) functionalized Ag nanoparticles (CA-AgNPs) have been characterized by Ultraviolet-visible spectrophotometry (UV), Fourier transform infrared absorption spectra (FTIR), Atomic force microscope (AFM), Transmission electron microscope (TEM). UV absorption results indicated that a strong absorption band peaked at 452 nm which can be ascribed to the surface plasmon resonance (SPR) of AgNPs. FTIR results of CA-AgNPs indicated that cinnamic acid was indeed bound on the AgNPs. TEM measurements indicated that the products are round spherical NPs with the average particle size of 52.8 nm. Antimicrobial activity tests revealed that CA-AgNPs have significant inhibitory effects on E. coli and C. albicans. The CA-AgNPs prepared in this study are expected to be developed as efficient antimicrobial agents.
Graphical Abstract
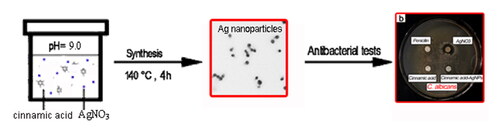
1. Introduction
Silver (Ag) nanomaterials have received considerable attention in recent years owning to their potential applications in wound dressing, orthopedic implants, food preservation, protective clothing and cosmetics as antimicrobial and biocidal agents [Citation1, Citation2]. One advantage of Ag nanomaterials as the potent antimicrobial agent is that they have strong biocidal efficiency and broad antimicrobial spectra. Furthermore, Ag nanomaterials have the potential to overcome the complication of bacterial resistance which devoted by commonly used antibiotics in combating bacterial infection for biomedical application [Citation3–5]. The effectiveness of Ag nanomaterials on a broad spectrum of pathogenic bacteria and fungi has been verified in recent studies [Citation6–8]. The formation and release of Ag+ and Ag2+ ions on Ag nanomaterials surface under an aqueous environment were considered as the main pathway to produce biocidal effect [Citation6]. Recent studies have also revealed that the antibacterial activity of AgNPs was significantly influenced by the size distribution, morphology, surface charge, surface chemistry, and capping agents and so on [Citation9, Citation10]. Various Ag nanomaterials with different kind of functionalized molecules have been synthesized and their antimicrobial activity varied with the initial synthesis approaches [Citation11–13]. Among the variety of synthesis methods, green synthesis strategy has attracted considerable attention due to their eco-friendly advantages over conventional methods involving chemical agents associated with environmental toxicity [Citation12]. Synthesis of nanometals using plant extracts has been considered as a fast and nontoxic methods for production of eco-friendly AgNPs [Citation10, Citation14, Citation15]. Hamed Barabadi et al. fabricated Zataria multiflora-derived AgNPs with strong antibacterial activity and biofilm inhibitory activity against S. aureus [Citation16]. Mentha pulegium extracts have been used as both reducing and stabilizing agents to prepare the plant extract modified AgNPs which have displayed promising antibacterial activity on Escherichia coli, Staphylococcus aureus, and Streptococcus pyogenes [Citation17]. M. Umadevi et al. utilized the aqueous beetroot extract as reducing agent to synthesize AgNPs. The prepared silver nanoparticles were effective in inhibiting the growth of both gram-positive and gram-negative bacteria [Citation18]. These studies indicated that green synthesis of AgNPs by using plant active components or plant extracts is promising strategy to obtain novel antimicrobial agent with high activity and broad spectrum.
Cinnamic acid is an aromatic carboxylic acid occurring naturally in plants with the formula C6H5CH = CHCOOH. It is a key intermediate in the shikimate and phenylpropanoid pathways leading to phenyl-propanoids, coumarins, flavonoids and the plant structural component lignin [Citation19]. Cinnamic acid has low toxicity and a broad spectrum of biological activities such as antimicrobial activities and anticancer effects derived from its ester derivatives [Citation20, Citation21]. It is also an important chemical intermediate such as its esters using as fragrances and pharmaceuticals, and its derivatives as antioxidants. It is also well known that cinnamic acid has weak antimicrobial activity [Citation22, Citation23]. Cinnamic acid derivatives are important and promising compounds with high potential for development into drugs since some of derivatives have been proved to be the most potent lipoxygenase inhibitor [Citation24].
In order to improve the antimicrobial activity of Ag nanomaterial and minimum their toxicity, cinnamic acid has been explored for the synthesis of AgNPs. It is expected that the combination of cinnamic acid and Ag nanomaterial can obtain more efficient antimicrobial agent. In this study, cinnamic acid, the main active component of medicinal plant cinnamon, was utilized to prepare novel Ag nanoparticles for the potential antimicrobial application.
2. Materials and methods
2.1. Reagent and instrument
Cinnamic acid and penicillin were obtained from Macklin reagent (Shanghai China). AgNO3, ethanol and agar were analytical grade. Nutrient broth, ammonia (40%) and hydrochloric acid (20%) were used without further purification. E. coli and Candida albicans were purchased from the Institute of Applied Microbiology, Heilongjiang Academy of Sciences.
2.2. Preparation of CA-AgNPs
Pure cinnamic acid (0.0282 g) was weighed and placed into a 50 mL round bottom flask. Sufficient pure water was added and then ultrasound for 15 min under 50 °C water bath to completely dissolve the cinnamic acid. The dissolved solution was transferred into a 50 mL volumetric flask and diluted to the mark with pure water to obtain 3.8 mM cinnamic acid aqueous solution. 1% AgNO3 solution was prepared by dissolving 0.1 g AgNO3 solid into 10 mL pure water under ultrasonic assistance. The 40 mL as-prepared cinnamic acid solution was placed in a beaker. The solution pH was adjusted to 9.0 by addition of appropriated volume of the concentrated ammonia. 1.5 mL of 1% AgNO3 solution was then slowly added into the solution. The final mixed solution was transferred into a Teflon-lined autoclave of 50 mL. The autoclave was put into the oven and maintained at 140 °C for 4 h. After the reaction, the obtained products were collected for UV, FTIR, TEM, AFM and XPS characterization.
In this study, AgNPs were also prepared by sodium borohydride (NaBH4) reduction method for comparison [Citation25], which can be considered as the unfunctionalized AgNPs. The chemical reaction is the sodium borohydride reduction of silver nitrate. A 10-mL volume of 1.0 mM silver nitrate was gradually added to 30 mL of 2.0 mM sodium borohydride solution (chilled in an ice bath) within 3 min under stirring condition.
UV spectra were measured by UV-5500 ultraviolet-visible (UV) spectrophotometer (Yuan Analytical Instruments Co., Ltd., Shanghai). The as-prepared colloidal solution added into a quartz cuvette for the UV absorption measurement. Fourier transform infrared (FTIR) spectra were obtained by IR Affinity-1 FTIR absorption spectrometer (Shimadzu, Japan) using KBr pellet method. The solid CA-AgNPs sample was collected from the centrifugation of the initial colloidal solution. The topography of the synthesized nanoparticles was observed by transmission electron microscope (TEM) JM-2100 (JEOL Ltd.). The as-synthesized colloidal solution (15 μL) was dried on a carbon coated copper grid at room temperature and stored in a desiccator prior for TEM imaging. The nanoparticle sizes were further characterized by atomic force microscope (AFM) (Molecular Imaging, USA). 30 μL of the as-synthesized colloidal solution were dropped onto a freshly cleaved mica surface and then rinsed by a copious of pure water. The AFM samples were dried under a flow of pure Ar gas and stored in a desiccator prior to AFM imaging. X-ray photoelectron spectroscopy (XPS) spectra was determined by Philip's XPS diffractometer (Netherlands). The solid CA-AgNPs sample (20 mg) was used for the measurement. Thermogravimetric analysis (TGA) was conduct on thermogravimetric analyzer (NETZSCH DSC 204F1, Germany). The solid CA-AgNPs sample (5 mg) collected by the centrifugation of the initial colloidal solution was used for the measurement.
2.3. Antimicrobial test
E. coli, a common foodborne pathogenic microorganism, and C. albicans, the most pathogenic yeast species have been utilized for the following test. 300 μL of the diluted bacterial solution (1 × 106 CFU/mL) was smeared onto the agar medium plate. 20 μL of the CA-AgNPs solution and control samples (penicillin solution, cinnamic acid solution, AgNO3 solution, AgNPs by NaBH4 reduction) were dropped onto a filter paper and dried in air, then placed the filter paper onto the agar medium plate. The plates were incubated at 37 °C (E. coli) and 28 °C (C. albicans) for 24 h. The inhibit growth effect around the filter paper was photographed with a digital camera.
Minimum inhibitory concentration (MIC) and minimum bactericidal concentration of CA-AgNPs (MBC) against E. coli and C. albicans were determined by double dilution method. The initial concentration of a solution of silver colloid solution is 188.4 μg/mL. It was successively diluted to 0.74 μg/mL with the nutrient solution, and transferred 100 μL solution to the 96 well microtiter plate. 100 μL of fresh bacteria suspension (1 × 106 CFU/mL) was transferred into the wells. The microtiter plate was incubated at 37 °C (E. coli) and 28 °C (C. albicans) for 24 h. After incubation, 10 μL of the suspensions were taken out and re-inoculated on the agar plate for 24 h to observe the bacterial growth.
3. Results and discussion
3.1. Characterization of the CA-AgNPs
shows the UV absorption spectra of CA-AgNPs solution (a) and pure cinnamic acid solution (b). The inset in is a photograph of CA-AgNPs colloidal solution. It is a homogeneous solution with yellow green color. There is a strong absorption band peaked at 452 nm in the UV spectrum in whereas no absorption band appeared at this range for the pure cinnamic acid solution. It can be ascribed to the surface plasmon resonance (SPR) of the formed AgNPs. It has been revealed that the SPR of AgNPs differed with their size and concentrations [Citation26]. In addition, a single surface plasmon resonance band indicates that the synthesized Ag nanomaterials are mainly spherical and not form aggregates according to the Mie theory [Citation27, Citation28].
shows FTIR spectrum of CA-AgNPs (line a) and pure cinnamic acid (line b). In the FTIR spectrum of pure cinnamic acid (line b), the broad absorption at 3445 cm−1 is ascribed to the stretching of the hydroxyl of carboxyl groups and their hydrogen effects [Citation29]. The absorption peaks higher than 3000 cm−1 (3065 and 3026 cm−1) and lower than 3000 cm−1 (2829 cm−1) are attributed to the unsaturated C-H vibration saturated C-H vibration of cinnamic acid, respectively. The strong absorption at 1680 cm−1 is attributed to the stretching of the carboxyl groups, and absorption band at 1626 cm−1, 1574 cm−1 and 1558 cm−1was ascribed to the stretching of C = C group of the aromatic ring. In the FTIR spectra of CA-AgNPs, the adsorption band corresponding to the stretching of the hydroxyl shift to 3523 cm−1with the increase of the absorption strength. In addition, the adsorption band corresponding to the carboxyl groups shift to 1649 cm−1. These results indicated that the carboxyl groups of the cinnamic acid existed on the surface of AgNPs and have a strong interaction with surface Ag atoms.
shows the representative TEM image of the obtained products. It can be seen that the most products are spherical AgNPs. The size of these AgNPs ranges from 45 to 55 nm. The average diameter of the observed AgNPs is 52.8 ± 8.0 nm. The morphology of the AgNPs was further verified by atomic force microscopy (AFM) imaging as shown in . The average size of the AgNPs by AFM measurement is 53.4 nm. This result is very close to that of TEM measurement.
Figure 3. (a) Representative TEM image of the CA-AgNPs. (b) Atomic force microscopy (AFM) image of the CA-AgNPs.
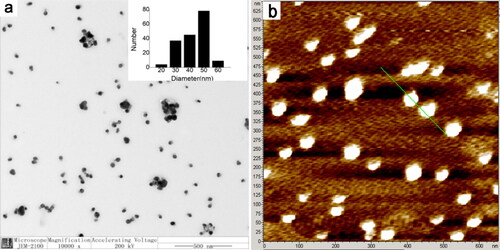
shows the X-ray photoelectron spectroscopy (XPS) of CA-AgNPs. The two peaks at the binding energies of 367 and 373 eV in XPS are attributed to the Ag 3d 5/2 and Ag 3d 5/2, respectively [Citation30]. This result indicates that the Ag exist in the metallic zero valent oxidation state on the surface of AgNPs.
shows the DSC curve of CA-AgNPs. It can be seen that the mass of the CA-AgNPs decreased at 157.51 °C, and there are three mass loss stages. The first mass loss stage at 127.51–257.51 °C can be ascribed to the dehydration process whereas the second mass loss stage at 257.51–462.51 °C is attributed to the pyrolysis process of the cinnamic acid capped on AgNPs [Citation31]. The third mass loss stage after 462.51 °C can be considered as the Ag vaporization from the AgNPs. The remained burning ash accounts for 64% of the total mass. This result further confirmed that the obtained nanomaterials are the cinnamic acid functionalized AgNPs in which the cinnamic acid was capped on the surface of AgNPs.
3.2. Antimicrobial activity of the CA-AgNPs
shows the zone of inhibition (ZOI) results of CA-AgNPs against E. coli and C. albicans. Cinnamic acid solution, AgNO3 solution, penicillin solution and AgNPs by NaBH4 reduction method were also tested for comparison. Penicillin, a commonly used antibiotic, is widely utilized as a positive control group to evaluate the antimicrobial ability [Citation32, Citation33]. It can be seen that the CA-AgNPs have high antimicrobial activity against E. coli and C. albicans. Especially for the C. albicans, the CA-AgNPs indicated more antimicrobial efficiency that of penicillin. It has been noted that cinnamic acid solution and AgNO3 solution also have antimicrobial activity. The antimicrobial activity of AgNO3 solution resulted from the Ag+, which has been verified in recent studies [Citation34, Citation35]. Cinnamic acid and its derivatives (esters, amides, aldehydes and alcohols) have been shown significant growth inhibition against one or several bacterial and fungal species [Citation20, Citation23]. However, the utilization of cinnamic acid as an antimicrobial is still not best optimal because of its low solubility in water. The antimicrobial activity of AgNPs by NaBH4 reduction method was less effective than those of CA-AgNPs as shown in .
The measured MIC and MBC of CA-AgNPs against E. coli and C. albicans was listed in . It can be seen that the MIC and MBC of CA-AgNPs are lower than those of AgNPs by NaBH4 reduction method. It is revealed that the antimicrobial efficiency of the CA-AgNPs is very close to that of penicillin according to MIC and MBC value in . Especially for the C. albicans, the MIC of the synthesized AgNPs is even lower than that of penicillin. The fungal pathogen C. albicans featured with a multilayered cell wall structure which is made up of an outer layer of proteins glycosylated with mannosyl residues and an inner skeletal layer of β-glucans and chitin [Citation36]. It should be noted that the MIC and MBC of CA-AgNPs against E. coli and C. albicans are lower those of the biogenic AgNPs in recent study [Citation37].
Table 1. The MIC and MBC of CA-AgNPs and control samples.
The possible antimicrobial mechanism of the CA-AgNPs was schematic illustrated in . One possible antimicrobial action of CA-AgNPs against C. albicans is derived from the continuously release of Ag+ ions from Ag nanoparticle surface by oxidation reaction with dissolved O2 in the surrounding environment. The released Ag ions can interact with proteins and glucans on outer and inner layer, and damage the cell wall structure of E. coli and C. albicans resulting in the loss of important components in cells to achieve the antimicrobial effect. Furthermore, the cinnamic acid molecules capped on AgNPs facilitates their attachment on the surface of the bacterial and fungal cell. Cinnamic acid has been shown a weak antimicrobial effect against bacteria (MIC values higher than 38 mM in this study) and a weak anti-fungal activity, which are in accordance with the previous report [Citation23]. Therefore, there may exist synergistic effects between cinnamic acid and Ag components accounted for the superior antimicrobial activity in this study. The detailed antimicrobial mechanism of CA = AgNPs and their further applications are underway.
4. Conclusion
In this study, cinnamic acid, an active component of plants, was used as reducing agent and stabilizer to synthesize CA-AgNPs. The synthesized CA-AgNPs were characterized by UV, FTIR, TEM, AFM and XPS. The results indicated that the obtained CA-AgNPs capped by cinnamic acid, the average particle size was 52.8 nm. Antimicrobial test revealed that CA-AgNPs had obvious inhibitory effect on E. coli and C. albicans. The CA-AgNPs prepared in this study are expected to be developed as the efficient antimicrobial agents.
Acknowledgments
The authors gratefully acknowledge the financial support by the Fundamental Research Funds for the Central Universities (2572020DR07), Natural Science Fund of Heilongjiang Province (LH2019B001), and the 111 Project (B20088) Heilongjiang Touyan Innovation Team Program (Tree Genetics and Breeding Innovation Team).
Disclosure statement
The authors declare that they have no conflict of interest.
Additional information
Notes on contributors
Congcong Sun
Congcong Sun obtained his B.S. degree in biotechnology from Jining normal university. Now, he is studying for master's degree at Northeast Forestry University. His current research interests include the extraction of plant polyphenols and green synthesis of metal nanomaterials.
Hongxin Zhi
Hongxin Zhi obtained her B.S. degree in biotechnology from Daqing normal university. Now, she is studying for master's degree at Northeast Forestry University. Her current research interests include photochemistry and green synthesis of metal nanomaterials.
Han Li
Han Li is an undergraduate student in College of Chemistry, Chemical Engineering and Resource Utilization, Northeast Forestry University. She participates a college Students’ innovation project about nanomaterial synthesis supervised by prof. Zhiguo Liu.
Jingchao Li
Jingchao Li obtained her B.S. degree in biotechnology from Jining normal university. She is studying for master’s degree at Northeast Forestry University. Her current research interests include synthesis and application of metal nanomaterials.
Kai Shao
Kai Shao obtained her B.S. degree in horticulture from North china institute of aerospace engineering. She is studying for master’s degree at Northeast Forestry University. His current research interests include synthesis and application of the inorganic nanomaterials.
Yamei Lin
Yamei Lin obtained her B.S. degree in biotechnology from FUJIAN agriculture and Forestry university. Now, she is studying for master’s degree at Northeast Forestry University. Her current research interests include the extraction of plant active components by new extraction methods.
Yujie Fu
Yujie Fu obtained her PhD degree in botany from Northeast Forestry University. She is a professor at Key Laboratory of Forest Plant Ecology, Ministry of Education, Northeast Forestry University. Her current research interests include photochemistry and Phytomedicine.
Zhiguo Liu
Zhiguo Liu obtained her PhD degree in analytical chemistry from State Key Laboratory of Electroanalytical Chemistry, Changchun Institute of Applied Chemistry, Chinese Academy of Sciences, China. Now, he is a professor at Key Laboratory of Forest Plant Ecology, Ministry of Education, Northeast Forestry University. His current research interests include photochemistry and nanotechnology.
References
- Moritz M, Geszke-Moritz M. The newest achievements in synthesis, immobilization and practical applications of antibacterial nanoparticles. Chem Eng J. 2013;228:596–613.
- Yun'An Q, Lin C, Li R, et al. Potential antibacterial mechanism of silver nanoparticles and the optimization of orthopedic implants by advanced modification technologies. Int J Nanomedicine. 2018;13:3311–3327.
- Zhang X, Shi X, Gautrot JE, et al. Nanoengineered electrospun fibers and their biomedical applications: a review. Nanocomposites. 2021;7(1):1–34.
- Aigbodion VS. Explicit microstructure and electrical conductivity of epoxy/carbon nanotube and green silver nanoparticle enhanced hybrid dielectric composites. Nanocomposites. 2021;7(1):35–39.
- Ito H, Sakata M, Hongo C, et al. Cellulose nanofiber nanocomposites with aligned silver nanoparticles. Nanocomposites. 2018;4(4):167–111.
- Saidin S, Jumat MA, Amin N, et al. Organic and inorganic antibacterial approaches in combating bacterial infection for biomedical application. Mater Sci Eng C Mater Biol Appl. 2021;118:111382.
- Korkmaz N, Ceylan Y, Taslimi P, et al. Biogenic nano silver: synthesis, characterization, antibacterial, antibiofilms, and enzymatic activity. Adv Powder Technol. 2020;31(7):2942–2950.
- Kaur A, Preet S, Kumar V, et al. Synergetic effect of vancomycin loaded silver nanoparticles for enhanced antibacterial activity. Colloids Surf B Biointerfaces. 2019;176:62–69.
- Wilkinson LJ, White RJ, Chipman JK. Silver and nanoparticles of silver in wound dressings: a review of efficacy and safety. J Wound Care. 2011;20(11):543–549.
- Saravanan M, Barabadi H, Vahidi H. Green nanotechnology: isolation of bioactive molecules and modified approach of biosynthesis. In: Patra C, Ahmad I, Ayaz M, Khalil AT, Mukherjee S, Ovais M, editors. Biogenic nanoparticles for cancer theranostics. Amsterdam, Netherlands: Elsevier; 2021. p. 101–122.
- Chernousova S, Epple M. Silver as antibacterial agent: ion, nanoparticle, and metal. Angew Chem Int Ed. 2013;44(24):1636–1653.
- Sharma VK, Yngard RA, Lin Y. Silver nanoparticles: green synthesis and their antimicrobial activities. Adv Colloid Interface Sci. 2009;145(1-2):83–96.
- Bonilla-Gameros L, Chevallier P, Sarkissian A, et al. Silver-based antibacterial strategies for healthcare-associated infections: processes, challenges, and regulations. An integrated review. Nanomedicine. 2020;24:102142.
- Iravani S. Green synthesis of metal nanoparticles using plants. Green Chem. 2011;13(10):2638–2650.
- Vahidi H, Kobarfard F, Alizadeh A, et al. Green nanotechnology-based tellurium nanoparticles: exploration of their antioxidant, antibacterial, antifungal and cytotoxic potentials against cancerous and normal cells compared to potassium tellurite. Inorg Chem Commun. 2021;124:108385.
- Barabadi H, Mojab F, Vahidi H, et al. Green synthesis, characterization, antibacterial and biofilm inhibitory activity of silver nanoparticles compared to commercial silver nanoparticles. Inorg Chem Commun. 2021;129:108647.
- Kelkawi A, Kajani AA, Bordbar A-K. Green synthesis of silver nanoparticles using mentha pulegium and investigation of their antibacterial, antifungal and anticancer activity. IET Nanobiotechnol. 2017;11(4):370–376.
- Bindhu MR, Umadevi M. Antibacterial and catalytic activities of green synthesized silver nanoparticles. Spectrochim Acta A Mol Biomol Spectrosc. 2015;135:373–378.
- Ruwizhi N, Aderibigbe BA. Cinnamic acid derivatives and their biological efficacy. Int J Mol Sci. 2020;21(16):5712.
- Sova M. Antioxidant and antimicrobial activities of cinnamic acid derivatives. Mini Rev Med Chem. 2012;12(8):749–767.
- Qian Y, Zhang HJ, Hao Z, et al. Synthesis, molecular modeling, and biological evaluation of cinnamic acid metronidazole ester derivatives as novel anticancer agents. Bioorg Med Chem. 2010;18(14):4991–4996.
- Amaliyah N, Sarjono PR, Ngadiwiyana N, et al. Antibacterial activity of cinnamic acid - chitosan encapsulation. J Kim Sains Apl. 2018;21(1):8–12.
- Juan G. Natural cinnamic acids, synthetic derivatives and hybrids with antimicrobial activity. Molecules. 2014;19:19292–19349.
- Pontiki E, Hadjipavlou-Litina D, Litinas K, et al. Novel cinnamic acid derivatives as antioxidant and anticancer agents: design, synthesis and modeling studies. Molecules. 2014;19(7):9655–9674.
- Mulfinger L, Solomon SD, Bahadory M, et al. Synthesis and study of silver nanoparticles. J Chem Educ. 2007;84(2):322–325.
- Thouti E, Chander N, Dutta V, et al. Optical properties of Ag nanoparticle layers deposited on silicon substrates. J Opt. 2013;15(3):035005.
- Ren KF, Gréhan G, Gouesbet G. Prediction of reverse radiation pressure by generalized Lorenz-Mie theory. Appl Opt. 1996;35(15):2702–2710.
- RL, CP, Scott M, Alexander. Intriguing branching of the maximum position of the absorption cross section in Mie theory explained. Opt Lett. 2020;45:4056–4059.
- Madhurambal G, Ravindran B, Mariappan M, et al. Growth and characterization of cinnamic acid–urea single crystal. J Therm Anal Calorim. 2011;104(3):875–878.
- Saikia M, Das T, Saikia BK. A novel rapid synthesis of highly stable silver nanoparticle/carbon quantum dot nanocomposites derived from low-grade coal feedstock. New J Chem. 2022;46(1):309–321.
- Fernandes FHA, Santana CP, Santos RL, et al. Santos, thermal characterization of dried extract of medicinal plant by DSC and analytical techniques. J Therm Anal Calorim. 2013;113(2):443–447.
- Biao L, Tan S, Wang Y, et al. Synthesis, characterization and antibacterial study on the chitosan-functionalized Ag nanoparticles. Mater Sci Eng C Mater Biol Appl. 2017;76:73–80.
- Kim YH, Kim GH, Yoon KS, et al. Comparative antibacterial and antifungal activities of sulfur nanoparticles capped with chitosan. Microb Pathog. 2020;144:104178.
- Liu J, Sonshine DA, Shervani S, et al. Controlled release of biologically active silver from nanosilver surfaces. ACS Nano. 2010;4(11):6903–6913.
- Biao L, Tan S, Zhang X, et al. Synthesis and characterization of proanthocyanidins-functionalized Ag nanoparticles. Colloids Surf B. 2018;169:438–443.
- Netea MG, Gow N, Munro CA, et al. Immune sensing of Candida albicans requires cooperative recognition of mannans and glucans by lectin and toll-like receptors. J Clin Invest. 2006;116(6):1642–1650.
- Wypij M, Czarnecka J, Świecimska M, et al. Synthesis, characterization and evaluation of antimicrobial and cytotoxic activities of biogenic silver nanoparticles synthesized from Streptomyces xinghaiensis OF1 strain. World J Microbiol Biotechnol. 2018;34(2):23.