ABSTRACT
Volatile compounds (VCs) produced by diverse microbes seem to affect plant growth, development and/or stress tolerance. We investigated how VCs released by soilborne fungi Fusarium oxysporum and Verticillium dahliae affect Arabidopsis thaliana responses to abiotic and biotic stresses. Under salt stress, VCs from both fungi helped its growth and increased chlorophyll content. However, in contrast to wild-type A. thaliana (Col-0), V. dahliae VCs failed to increase leaf surface area in auxin signalling mutants aux1-7, tir1-1 and axr1-3. Compared to wild-type Col-0, the degree of lateral root density enhanced by V. dahliae VCs in these mutants was also reduced. Consistent with the involvement of auxin signalling in fungal VC-mediated salt torelance, A. thaliana line carrying DR5::GUS displayed increased auxin accumulation in root apex upon exposure to V. dahliae VCs, and 1-naphthylphthalamic acid, an auxin transport inhibitor, adversely affected V. dahliae VC-mediated salt tolerance. F. oxysporum VCs induced the expression of PR1 but not PDF1.2 in A. thaliana lines containing PR1::GUS and PFD1.2::GUS. When challenged with Pseudomonas syringae after the exposure to F. oxysporum VCs, A. thaliana showed reduced disease symptoms. However, the number of bacterial cells in F. oxysporum VC-treated plants was not significantly different from that in control plants.
Introduction
Even though plants have evolved multiple mechanisms to mitigate the effect of various biotic and abiotic stresses (Fujita et al. Citation2006; Harrison Citation2012; Huang et al. Citation2012), high levels of stress adversely affect plant growth and development, resulting in reduced crop productivity and health. Increasing salinity in certain arable lands, due to irrigation, draught or a combination of both, is one of the most serious abiotic threats to global crop production, because most crops are sensitive to high levels of NaCl (Xiong and Zhu Citation2002). Almost one billion hectares, representing about 7% of world’s land, are affected by high levels of salt (Metternicht and Zinck Citation2003). High soil salinity causes ion toxicity, osmotic and oxidative stress, and nutrient deficiency and also limits plant’s ability to uptake water from the soil (Bano and Fatima Citation2009). Multiple morphological, physiological and biochemical processes have been shown to be affected by salt (Munns and Tester Citation2008; Deinlein et al. Citation2014).
To ensure that the production of food, fibre and feed meets growing global demand, novel strategies for ensuring crop health in the presence of this and other types of stress are urgently needed. Deployment of evolutionarily fine-tuned beneficial plant–microbe partnerships and some of the processes underpinning their interactions has been promoted as one such strategy. Certain rhizosphere microbes are known to alleviate plant stress caused by high salinity via various mechanisms, including manipulation of plant hormone signalling (Yang et al. Citation2009; Shrivastava and Kumar Citation2015; Lenoir et al. Citation2016). Achromobacter piechaudii strain ARV8 that expresses ACC deaminase increased tomato tolerance to salt stress, presumably by reducing the production of ethylene (Mayak et al. Citation2004). Trichoderma virens and Trichoderma atroviride helped the growth of Arabidopsis thaliana under high NaCl conditions by restoring auxin homeostasis (Contreras-Cornejo et al. Citation2014). Volatile compounds (VCs) produced by some plant growth promoting rhizobacteria (PGPR) have been shown to alleviate plant salt stress (Zhang et al. Citation2008, Citation2010; Vaishnav et al. Citation2015, Citation2016; Ledger et al. Citation2016). VCs produced by Bacillus amyloliquefaciens GB03 conferred increased salt tolerance in A. thaliana presumably via tissue-specific regulation of HKT1, a high-affinity K+ transporter, and induced accumulation of osmoprotectants (Zhang et al. Citation2008, Citation2010). Pseudomonas simiae AU produced VCs that enhanced the growth of soybean seedlings under salt stress, and the underlying mechanism appears to include up-regulation of several salt stress-related proteins (Vaishnav et al. Citation2015). Similar to increased salt stress tolerance caused by VCs from PGPRs, application of individual VCs (e.g. 4-nitroguaiacol and quinoline) or a mix of VCs (e.g. 2-undecanone, 7-hexanol and 3-methylbutanol) also caused better growth under salt stress (Ledger et al. Citation2016; Vaishnav et al. Citation2016).
Besides increased salt tolerance, VCs produced by some bacteria and fungi also have been shown to elicit induced systemic resistance (ISR), a defence mechanism that protects plants from diverse pathogens (Bitas et al. Citation2013; Farag et al. Citation2013; Song and Ryu Citation2013; Chung et al. Citation2016; Li et al. Citation2016). For example, 1-octen-3-ol, a VC commonly produced by fungi, induced the expression of several defence-related genes in A. thaliana, and upon inoculation with Botrytis cinerea disease, symptom was less severe in 1-octen-3-ol-treated plants than control plants (Kishimoto et al. Citation2007). More examples of microbial VCs as potential elicitors for ISR were reviewed by Bitas et al. (Citation2013) and Li et al. (Citation2016).
Here, we investigated how fungal VCs affect salt tolerance and pathogen defence in plants using A. thaliana and soilborne fungi Fusarium oxysporum and Verticillium dahliae, the causative agents of vascular wilt diseases in diverse plants. VCs produced by both fungi have been shown to affect plant growth and development, and the underlying mechanism involves components of auxin signalling (Bitas et al. Citation2015; Li et al. Citation2018). However, how their VCs affect plant responses to (a)biotic stresses has not been studied.
Materials and methods
Fungal cultures and plant materials
F. oxysporum strains NRRL26379 and NRRL38335 were obtained from the USDA ARS Culture Collection (Peoria, IL). V. dahliae strains PD322 and PD413 were provided by Dr. Krishna Subbarao at University of California-Davis. These strains, preserved as conidial suspension in 20% glycerol, were revitalised by culturing on half-strength Potato Dextrose Agar (PDA; Becton, Spark, MD) at room temperature. Seeds of A. thaliana ecotype Col-0 were purchased from Lehle Seed Co. (Round Rock, TX). Two transgenic Col-0 lines containing PR1::GUS and PDF1.2::GUS, respectively, and auxin signalling mutants, including CS3074 (aux1-7), CS3075 (axr1-3) and CS3798 (tir1-1), were obtained from the Arabidopsis Biological Resource Center at the Ohio State University. Transgenic Col-0 line containing DR5::GUS was provided by Dr. Darrell Desveaux at University of Toronto.
Assessment of plant salt tolerance upon fungal VC exposure
Bi-partite Petri plate (=I plate), which has been widely used for studying VC-mediated interaction between fungi and plants (Zhang et al. Citation2008; Paul and Park Citation2013; Bitas et al. Citation2015), was employed for this assessment. One compartment of I plate contained 12 ml half-strength PDA for culturing fungus, and the other compartment had 12 ml Murashige and Skoog (MS) medium (Sigma-Aldrich, St. Louis, MO) supplemented with no NaCl or 100 mM NaCl. Seed treatment was conducted as described in Bitas et al. (Citation2015). Arabidopsis is a glycophytic plant and is sensitive to high salt throughout its development stages, especially during seed germination and seedling (Xiong and Zhu Citation2002), Accordingly, prior to exposing A. thaliana seedlings to 100 mM NaCl, they were germinated and grown on MS medium supplemented with 0.8% (w/v) agar and 0.25% (w/v) sucrose for 7 days. One plug of fungal culture (5 mm in diameter) was inoculated on the PDA side, and five Col-0 seedlings were transplanted to the MS side to initiate co-cultivation (Bitas et al. Citation2015). The control treatment for all experiments consisted of PDA alone (no fungus). Growth chamber conditions for co-cultivation were 22°C, 12 h light (4500 lx, 60 μmol photons/m2 s) and 60% relative humidity.
Quantification of foliar and root growth
After co-cultivation for 14 days, roots were weighed immediately. Chlorophyll content was measured spectrophotometrically as previously described (Hiscox and Israelstam Citation1979). Leaf surface area was quantified as reported by Zhang et al. (Citation2008). Seedlings were photographed using a digital camera (Nikon D80). Resulting images were imported into Adobe Photoshop CS4 (Adobe Systems, San Jose, CA) for measuring leaf surface area using a histogram function. Primary root length was measured by analysing photographed roots using ImageJ (http://imagej.nih.gov/ij/). The number of lateral roots was counted under stereomicroscope. Lateral root density was calculated by dividing the total number of lateral roots by primary root length.
NPA treatment
MS medium was amended with 1-naphthylphthalamic acid (NPA, Sigma-Aldrich) to determine whether polar auxin transport is critical for mediating the effect of V. dahliae VCs on plant growth in the presence of 100 mM NaCl. NPA was dissolved in DMSO, and the corresponding control treatment was done on MS medium amended with the same volume of DMSO only. Both leaf surface area and lateral root density were determined.
Assessment of GUS activity
Histochemical staining to assess GUS activity in three transgenic A. thaliana lines that contain DR5::GUS, PR1::GUS and PDF1.2::GUS, respectively, was performed as previously described (Bitas et al. Citation2015). Vacuum was applied for 5 min to seedlings in 50 mM sodium phosphate (pH 7.0), 0.1% (v/v) Triton X-100, 2 mM K4Fe(CN)6, 2 mM K3Fe(CN)6 and 2 mM X-Gluc (5-bromo-4-chloro-3-indolyl-beta-d-glucuronic acid, cyclohexylammonium salt, Gold Biotechnology, Olivette, MO). Subsequently, they were incubated for 15 h at 37°C in the dark with gentle agitation (75 rpm). Stained seedlings were cleared and dehydrated by washing with 70% ethanol solution for 30 min. After repeated washing to remove all chlorophylls, they were stored in 90% ethanol at 4°C until observation using a stereomicroscope (Olympus SZ60) and a compound microscope (Nikon 104). Images of plants were taken using Olympus DP26 camera.
Infection of A. thaliana with P. syringae
Bacterial inoculum was prepared as previously described (Katagiri et al. Citation2002). P. syringae pathovar tomato (Pst) strain, DC3000, was cultured in King’s B (KB) medium containing kanamycin (50 mg/L) for 15 h at 200 rpm at 28°C. After washing bacterial cells collected by centrifugation twice with sterile water, they were resuspended in sterile water containing 0.02% Silwet L77 and 10 mM MgCl2 to the final concentration of 2 × 108 colony-forming units (CFU)/mL. Seedlings of A. thaliana were co-cultivated with individual fungal strains for 12 days in I plate as described above. On the day of bacterial inoculation, fungal culture along with PDA was removed. Four basal leaves were inoculated with 5 μL each of the bacterial suspension. After inoculation, I plates were sealed with Parafilm and placed in the plant growth chamber for 5 days.
Disease severity was scored as the percentage of total leaf surface displaying chlorotic or necrotic symptoms, ranging from 0 (no symptom) to 100 (whole leaf affected), as previously described (Hossain et al. Citation2007). To determine the density of Pst DC3000 cells in inoculated leaves, CFU per gram of leaves was determined. Harvested leaves were weighed, surface sterilised (soaked in 70% ethanol for 15 s), rinsed thoroughly in sterile water and then homogenised in sterile water. Diluted leaf suspensions were plated on KB agar supplemented with kanamycin (50 mg/L). After 2 days of incubation at 28°C, bacterial colonies were counted.
Statistical analysis
The experimental design was completely randomised, consisting of three replications for each treatment. Each experiment was repeated at least twice. One-way analysis of variation (ANOVA) to assess the effects of fungal VCs on growth was done using Minitab 17.3 (Minitab Inc., State College, PA). The significance of treatment was determined using the F value. When a significant F test was obtained for treatment, separation of the means was preformed using Fisher’s least significant difference test. Significance of the statistical tests was evaluated at P < 0.05.
Results and discussion
VCs emitted by both F. oxysporum and V. dahliae increased salt tolerance in A. thaliana
To investigate how VCs produced by these fungi affect plant growth under salt stress, we employed two strains each of F. oxysporum (NRRL26379 and NRRL38335) and V. dahliae (PD322 and PD413). These strains were chosen based on the ability of their VCs to enhance plant growth and development (Bitas et al. Citation2015; Li et al., under revision). In agreement with our previous results, their VCs significantly enhanced the growth of A. thaliana in the absence of NaCl (). In the presence of 100 mM NaCl, A. thaliana seedlings exposed to fungal VCs displayed much more robust foliar growth () than control seedlings. In addition, VC-treated plants had increased leaf chlorophyll content ()) and lateral root density ()). Similarly, VCs produced by certain PGPR strains also improved plant growth under salt stress (Zhang et al. Citation2008, Citation2010; Vaishnav et al. Citation2015, Citation2016; Ledger et al. Citation2016). Decreased leaf surface area and chlorophyll content are common plant responses to salt stress, resulting in reduced photosynthesis and growth (Netondo et al. Citation2004; Hasanuzzaman et al. Citation2013; Negrão et al. Citation2017). The ability of F. oxysporum and V. dahliae VCs to maintain leaf surface area and to prevent chlorophyll degradation seems to mitigate the adverse effect of salt stress on photosynthesis, thus helping sustain growth. Moreover, increased lateral root density in response to VC exposure would certainly help nutrient acquisition under unfavourable environmental conditions. Increased salt tolerance would benefit the growth of F. oxysporum and V. dahliae by ensuring the supply of root-derived nutrients such as root exudates and dead root cells and soil nutrient mobilisation by plants.
Figure 1. Effect of fungal VCs on A. thaliana in the presence of NaCl. Col-0 seedlings on medium containing no NaCl and 100 mM NaCl were co-cultivated with PDA only (control), two F. oxysporum strains (NRRL26379 and NRRL38335) and two V. dahliae strains (PD322 and PD413) for 14 days. Representative images after treatment (a), leaf surface area (b), leaf chlorophyll content (c) and lateral root density (d) are shown. Values shown correspond to the mean ± SE of data from three replicates (n = 15). Different letters indicate significant difference among treatments, according to Fisher’s least significant difference (LSD) test at P = 0.05.
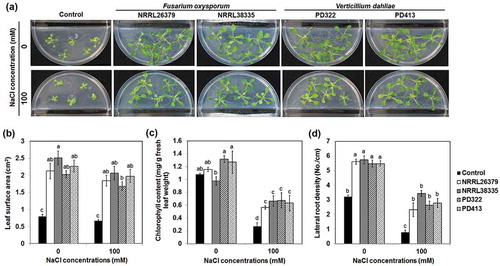
Auxin signalling is involved in increased salt tolerance caused by V. dahliae VCs
Maintenance of plant hormone homeostasis protects plants from salt-induced damages (Hasanuzzaman et al. Citation2013; Deinlein et al. Citation2014). The involvement of auxin in plant stress tolerance has been well established (Fu and Wang Citation2011; Pieterse et al. Citation2012; Naseem et al. Citation2015; Forni et al. Citation2017). Based on previous studies showing the critical role of auxin signalling in controlling plant responses to VCs produced by various microbes (Zhang et al. Citation2007; Splivallo et al. Citation2009; Bailly et al. Citation2014; Bitas et al. Citation2015; Garnica-Vergara et al. Citation2016), we investigated how VCs produced by two V. dahliae strains affect the growth of A. thaliana mutants defective in auxin influx (aux1-7) or response (tir1-1 and axr1-3) in the presence of 100 mM NaCl. These mutants were chosen because their growth responses to VCs produced by F. oxysporum and V. dahliae were defective (Bitas et al. Citation2015; Li et al. under revision). Upon exposure to V. dahliae VCs, the leaf surface area and lateral root density of all mutants did not increase as much as that of the wild type (). In particular, both the leaf surface area and lateral root density of aux1-7 did not look significantly different from those observed in control treatment. However, the tir1-1 and axr1-3 mutants displayed significantly increased lateral root densities when exposed to PD322 VCs compared to PD413 VCs and control treatment ()). These results indicated the involvement of AUX1, TIR1 and AXR1 in mediating increased salt tolerance conferred by V. dahliae VCs, with AUX1 playing a key role. It was reported that under high salt, the inhibition of lateral root formation is caused by suppression of basipetal auxin transport, resulting in over-accumulation of auxin in root epidermis of elongation zone while depleting auxin in root apex (Wang et al. Citation2009). AUX1 encodes a non-redundant auxin influx carrier (Marchant Citation1999), which facilitates auxin distribution in lateral root cap and elongation of epidermal cells (Marchant et al. Citation2002; Band et al. Citation2014). The aux1-7 mutant exhibited higher susceptibility to salt stress than the wild type, suggesting that auxin transport is required for alleviating salt stress (Wang et al. Citation2009; Galvan-Ampudia and Testerink Citation2011).
Figure 2. Growth responses of A. thaliana auxin signalling mutants to V. dahliae VCs in the presence of NaCl. Col-0 wild type (WT) and mutants of Col-0 defective in polar auxin transport (aux1-7) or auxin response (tir1-1 and axr1-3) on medium containing 100 mM NaCl were co-cultivated with PDA only (control) and V. dahliae strains PD322 and PD413 for 14 days. Leaf surface area (a) and lateral root density (b) after treatment are shown. Values shown correspond to the mean ± SE of data from three replicates (n = 15). Different letters indicate significant difference among treatments, according to Fisher’s LSD test at P = 0.05.
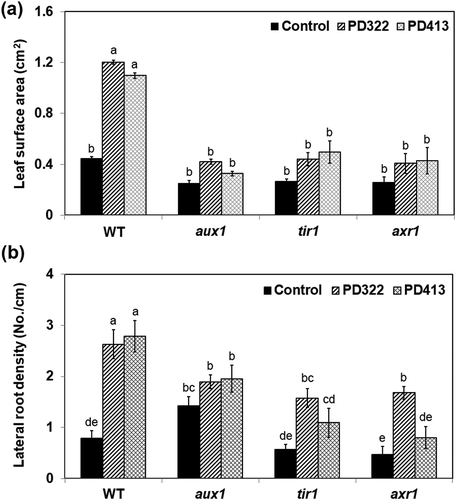
We hypothesise that V. dahliae VCs increase salt tolerance by facilitating auxin recycling mediated by polar auxin transport. To test this hypothesis, we first determined whether increased auxin accumulation in root apex could be observed after VC treatment using a transgenic A. thaliana line that contains the auxin-responsive reporter DR5::GUS. This reporter consists of the GUS (β-glucuronidase) gene under the control of DR5, a highly active auxin-responsive synthetic promoter (Ulmasov et al. Citation1997). After 7 days of co-cultivation with V. dahliae in the presence of 100 mM NaCl, a greater number of root apex displayed pronounced GUS staining compared to control plants (), indicating that increased auxin accumulation is associated with reduced inhibition of root growth in the presence of NaCl. A previous study showed that application of exogenous auxin led to increased length and cell number of root meristem in the presence of 100 mM NaCl (Liu et al. Citation2015).
Figure 3. Expression of GUS in root tips of transgenic A. thaliana Col-0 containing DR5::GUS. Plants were stained for GUS activity after 7 days of co-cultivation with PDA only (control) and V. dahliae strains PD322 and PD413. Plants were cultured on medium containing no NaCl or 100 mM NaCl. The number of root tips expressing GUS for each treatment is shown. Values shown correspond to the mean ± SE of data from three replicates (n = 15). Different letters indicate significant difference among treatments, according to Fisher’s LSD test at P = 0.05.
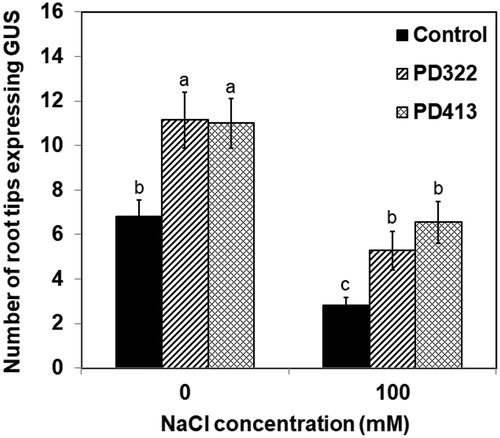
To further investigate whether polar auxin transport plays an important role in robust root growth under salt stress caused by V. dahliae VCs, plants grown on medium containing NPA, which disrupts auxin recycling in A. thaliana, were exposed to V. dahliae VCs. The NPA treatment reduced the growth enhancement (). The growth enhancement effect of PD322 VCs was completely blocked by NPA. However, PD413 VCs still increased plant growth in the presence of NPA. Analysis of VCs produced by PD322 and PD413 showed the production of both common and strain-specific compounds (Li et al. under revision). Six VCs, including 2-methyl-1-propanol, 3-octanone, 3-octanol, 1-octen-3-ol, himachala-2,4-diene and phenylethyl alcohol, were produced by both strains. PD322 emits two specific VCs (2-methyl-1-butanol and 4,11-selinadiene), while five VCs, including 3-methyl-1-butanol, 3-pentenol, 4-methyl-5-hexen-2-ol, 4-methyl-6-hepten-3-ol and 3,5,5-trimetyl-2-hexene, were uniquely produced by PD413. This finding suggested that the difference between the two V. dahliae strains may be caused by different VCs they produce.
Figure 4. Growth response of A. thaliana to V. dahliae VCs in the presence of an inhibitor of polar auxin transport, 100 mM NaCl, or both. Leaf surface area (a) and lateral root density (b) of Col-0 seedlings co-cultivated with PDA only (control) and V. dahliae strains PD322 and PD413 for 14 days under three conditions, including no NaCl, 100 mM NaCl (Na100) and a combination of 100 mM NaCl and 10 μM NPA (Na100 + NPA10), are shown. The same volume of DMSO was used for all treatments. Values shown correspond to the mean ± SE of data from three replicates (n = 15). Different letters indicate significant difference among treatments, according to Fisher’s LSD test at P = 0.05.
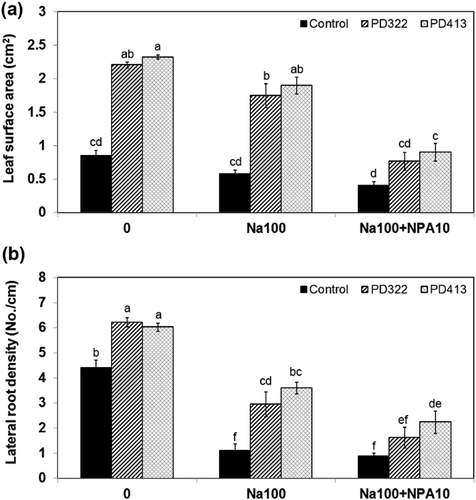
F. oxysporum VCs induced PR1 expression, but their effect on disease suppression varied depending on the strains used
Besides affecting growth and development, VCs produced by some microbes seem to function as elicitors for ISR (Bitas et al. Citation2013; Farag et al. Citation2013; Song and Ryu Citation2013; Chung et al. Citation2016; Li et al. Citation2016). To evaluate if VCs produced by F. oxysporum and V. dahliae also affect plant defence signalling, we employed two A. thaliana transgenic lines containing PR1::GUS and PDF 1.2::GUS, respectively. These reporters have been commonly used for monitoring the salicylic acid (SA)- and jasmonic acid/ethylene (JA/ET)-mediated defence signalling pathways, respectively. Compared to control plants and those exposed to V. dahliae PD322 VCs, PR1::GUS expression in plants exposed to VCs from NRRL26379 and NRRL38335 was noticeably higher after 7 days of co-cultivation ()), suggesting the induction of the SA-dependent signalling pathway by F. oxysporum VCs. Similar to our finding, the induced expression of PR1 was observed by VCs emitted by Paenibacillus polymyxa E681 and T. asperellum IsmT5 and by specific compounds (e.g. tridecane, m-cresol and methyl benzoate) (Lee et al. Citation2012; Naznin et al. Citation2014; Kottb et al. Citation2015). However, our analysis of VCs produced by these F. oxysporum strains did not reveal the production of these compounds (Bitas et al. Citation2015), suggesting the involvement of different compounds in activating the expression of PR1. Unlike the expression of PR1::GUS, PDF1.2::GUS was highly expressed in whole leaves of both control and fungal VC-treated plants ()). Previous studies reported that VCs emitted by several plant growth promoting fungi, including Ampelomyces sp., Cladosporium sp. and Trichoderma sp., reduced disease symptoms through the activation of signalling pathways that involve SA and JA/ET (Naznin et al. Citation2014; Kottb et al. Citation2015). However, Ryu et al. (Citation2004) reported that ISR elicited by VCs emitted by B. amyloliquefaciens GB03 was mediated by ET signalling but did not require the SA or JA signalling pathway. Different responses of A. thaliana to VCs from different microbes could depend on the type and amount of volatile elicitors released by individual microbes.
Figure 5. Expression of two defence-related genes in leaves of A. thaliana after exposure to fungal VCs. Transgenic A. thaliana Col-0 seedlings containing PR1::GUS (a) and PDF1.2::GUS (b), respectively, were co-cultivated with PDA only (control), V. dahliae (PD322) and F. oxysporum (NRRL26379 and NRRL38335) for 7 days. Blue staining indicates the activity of expressed GUS in planta. Scale bar = 500 μm.
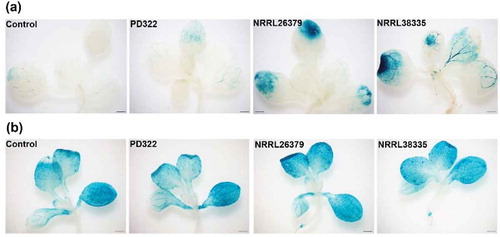
To investigate whether the activation of the SA signalling pathway by F. oxysporum VCs causes enhanced resistance to P. syringae, we infected A. thaliana with Pst DC3000. To eliminate the possibility that F. oxysporum VCs directly suppress P. syringae, we removed F. oxysporum culture from I plate prior to pathogen inoculation. VCs from NRRL26379 significantly reduced disease severity compared to control ()). However, no such effect was observed upon exposure to NRRL38335 VCs. To determine if reduced disease symptom was due to reduced bacterial density in infected leaves, the number of bacterial cells in plant apoplast was quantified. The bacterial cell density after the exposure to F. oxysporum VCs did not look significantly different from that in control plants ()). These results suggested that preexposure to VCs produced by NRRL26379 could reduce disease severity caused by P. syringae infection without suppressing the pathogen population in the leaves. Further studies are needed to understand the molecular mechanism underlying reduced disease severity caused by NRRL26379 VCs. A comprehensive analysis of gene expression profiles in response to VCs from various fungal species and strains and the evaluation of additional A. thaliana mutants defected in different hormonal pathways will help explore this mechanism.
Figure 6. Effect of F. oxysporum VCs on A. thaliana defence against P. syringae. After co-cultivating Col-0 seedlings with PDA only (control) and F. oxysporum (NRRL26379 and NRRL38335) for 12 days, the seedlings were inoculated with Pst DC3000. Disease severity (a) was scored as the percentage of total leaf surface with symptoms, ranging from 0 (no symptoms) to 100 (complete chlorosis or necrosis). The number of bacterial cells in inoculated leaves (b) under each treatment is shown. Values shown correspond to the mean ± SE of data from three replicates (n = 15). Different letters indicate significant difference among treatments, according to Fisher’s LSD test at P = 0.05.
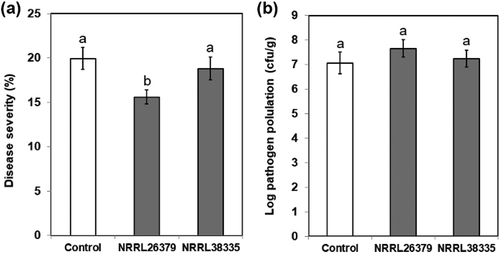
Conclusion
Plants and microbes have co-evolved and employ diverse strategies to affect each other. Some microbes have been shown to enhance the ability of plants to manage abiotic and biotic stresses by releasing phytohormones, siderophore and/or other small molecules (Ortíz-Castro et al. Citation2009; Vaishnav et al. Citation2013). In return, microbes may receive nutrients and better protected ecological niche. Recently, a number of studies suggested the role of microbial VCs in improving plant’s ability to manage different types of stress, including drought, salinity and pathogen infection (Ortíz-Castro et al. Citation2009; Bailly and Weisskopf Citation2012; Bitas et al. Citation2013; Farag et al. Citation2013; Kanchiswamy et al. Citation2015; Chung et al. Citation2016; Li et al. Citation2016; Piechulla et al. Citation2017). Here, we showed that VCs produced by soilborne fungal pathogens F. oxysporum and V. dahliae also help A. thaliana growth better in the presence of salt. The growth response of A. thaliana auxin signalling mutants, auxin response monitored using a reporter gene, and the effect of inhibition of auxin transport suggested that some of its components are needed to confer fungal VC-mediated enhanced salt tolerance. Further studies are needed to evaluate whether fungal VCs affect stress tolerance of plants under field conditions. Further elucidation of the molecular mechanism underlying VC-mediated effects on plants may present a novel strategy for better managing crop growth under biotic and abiotic stresses.
Acknowledgements
This work was supported by the USDA-NIFA Agriculture and Food Research Initiative Competitive Grants Program (award no. 2010-65110-20488), a fellowship from the Storkan-Hanes-McCaslin Foundation, the Penn State College of Agricultural Sciences (Jeanne & Charles Rider Endowment Award and Strategic Collaboration Seed Grant), the Huck Institutes of the Life Sciences at Penn State (the Huck Dissertation Research Award), and the Penn State Center for Environment geoChemistry and Genomics. We would like to thank Sara May for allowing us to use their equipment, Dr. Krishna Subbarao for V. dahliae strains and Dr. Darrell Desveaux for supplying transgenic Col-0 containing DR5::GUS.
Disclosure statement
No potential conflict of interest was reported by the authors.
Additional information
Funding
References
- Bailly A, Groenhagen U, Schulz S, Geisler M, Eberl L, Weisskopf L. 2014. The inter-kingdom volatile signal indole promotes root development by interfering with auxin signaling. Plant J. 80:758–771.
- Bailly A, Weisskopf L. 2012. The modulating effect of bacterial volatiles on plant growth. Plant Signal Behav. 7:79–85.
- Band LR, Wells DM, Fozard JA, Ghetiu T, French AP, Pound MP, Wilson MH, Yu L, Li W, Hijazi HI, et al. 2014. Systems analysis of auxin transport in the Arabidopsis root apex. Plant Cell. 26:862–875.
- Bano A, Fatima M. 2009. Salt tolerance in Zea mays (L). following inoculation with Rhizobium and Pseudomonas. Biol Fertil Soils. 45:405–413.
- Bitas V, Kim H-S, Bennett JW, Kang S. 2013. Sniffing on microbes: diverse roles of microbial volatile organic compounds in plant health. Mol Plant Microbe Interact. 26:835–843.
- Bitas V, McCartney N, Li N, Demers J, Kim J-E, Kim H-S, Brown KM, Kang S. 2015. Fusarium oxysporum volatiles enhance plant growth via affecting auxin transport and signaling. Front Microbiol. 6:1248.
- Chung J, Song GC, Ryu C-M. 2016. Sweet scents from good bacteria: case studies on bacterial volatile compounds for plant growth and immunity. Plant Mol Biol. 90:677–687.
- Contreras-Cornejo HA, Macías-Rodríguez L, Alfaro-Cuevas R, López-Bucio J. 2014. Trichoderma spp. improve growth of Arabidopsis seedlings under salt stress through enhanced root development, osmolite production, and Na+ elimination through root exudates. Mol Plant Microbe Interact. 27:503–514.
- Deinlein U, Stephan AB, Horie T, Luo W, Xu G, Schroeder JI. 2014. Plant salt-tolerance mechanisms. Trends Plant Sci. 19:371–379.
- Farag MA, Zhang H, Ryu C-M. 2013. Dynamic chemical communication between plants and bacteria through airborne signals: induced resistance by bacterial volatiles. J Chem Ecol. 39:1007–1018.
- Forni C, Duca D, Glick BR. 2017. Mechanisms of plant response to salt and drought stress and their alteration by rhizobacteria. Plant Soil. 410:335–356.
- Fu J, Wang S. 2011. Insights into auxin signaling in plant-pathogen interactions. Front Plant Sci. 2:74.
- Fujita M, Fujita Y, Noutoshi Y, Takahashi F, Narusaka Y, Yamaguchi-Shinozaki K, Shinozaki K. 2006. Crosstalk between abiotic and biotic stress responses: a current view from the points of convergence in the stress signaling networks. Curr Opin Plant Biol. 9:436–442.
- Galvan-Ampudia CS, Testerink C. 2011. Salt stress signals shape the plant root. Curr Opin Plant Biol. 14:296–302.
- Garnica-Vergara A, Barrera-Ortiz S, Muñoz-Parra E, Raya-González J, Méndez-Bravo A, Macías-Rodríguez L, Ruiz-Herrera LF, López-Bucio J. 2016. The volatile 6-pentyl-2H-pyran-2-one from Trichoderma atroviride regulates Arabidopsis thaliana root morphogenesis via auxin signaling and ETHYLENE INSENSITIVE 2 functioning. New Phytol. 209:1496–1512.
- Harrison MA. 2012. Cross-talk between phytohormone signaling pathways under both optimal and stressful environmental conditions. In: Khan NA, Nazar R, Iqbal N, Anjum NA, editors. Phytohormones and abiotic stress tolerance in plants. Berlin (Heidelberg): Springer; p. 49–76.
- Hasanuzzaman M, Nahar K, Fujita M. 2013. Plant response to salt stress and role of exogenous protectants to mitigate salt-induced damages. In: Ahmad P, Azooz MM, Prasad MNV, editors. Ecophysiology and responses of plants under salt stress (Vol. 44). New York (NY): Springer; p. 25–87.
- Hiscox JD, Israelstam GF. 1979. A method for the extraction of chlorophyll from leaf tissue without maceration. Can J Bot. 57:1332–1334.
- Hossain MM, Sultana F, Kubota M, Koyama H, Hyakumachi M. 2007. The plant growth-promoting fungus Penicillium simplicissimum GP17-2 induces resistance in Arabidopsis thaliana by activation of multiple defense signals. Plant Cell Physiol. 48:1724–1736.
- Huang G-T, Ma S-L, Bai L-P, Zhang L, Ma H, Jia P, Liu J, Zhong M, Guo Z-F. 2012. Signal transduction during cold, salt, and drought stresses in plants. Mol Biol Rep. 39:969–987.
- Kanchiswamy CN, Malnoy M, Maffei ME. 2015. Bioprospecting bacterial and fungal volatiles for sustainable agriculture. Trends Plant Sci. 20:206–211.
- Katagiri F, Thilmony R, He SY. 2002. The Arabidopsis thaliana-Pseudomonas syringae interaction. Arabidopsis Book. 1:e0039.
- Kishimoto K, Matsui K, Ozawa R, Takabayashi J. 2007. Volatile 1-octen-3-ol induces a defensive response in Arabidopsis thaliana. J Gen Plant Pathol. 73:35–37.
- Kottb M, Gigolashvili T, Großkinsky DK, Piechulla B. 2015. Trichoderma volatiles effecting Arabidopsis: from inhibition to protection against phytopathogenic fungi. Front Microbiol. 6:1–14.
- Ledger T, Rojas S, Timmermann T, Pinedo I, Poupin MJ, Garrido T, Richter P, Tamayo J, Donoso R. 2016. Volatile-mediated effects predominate in Paraburkholderia phytofirmans growth promotion and salt stress tolerance of Arabidopsis thaliana. Front Microbiol. 7:1838.
- Lee B, Farag MA, Park HB, Kloepper JW, Lee SH, Ryu C-M. 2012. Induced resistance by a long-chain bacterial volatile: elicitation of plant systemic defense by a C13 volatile produced by Paenibacillus polymyxa. PLoS One. 7:e48744.
- Lenoir I, Fontaine J, Lounès-Hadj Sahraoui A. 2016. Arbuscular mycorrhizal fungal responses to abiotic stresses: a review. Phytochemistry. 123:4–15.
- Li N, Alfiky A, Vaughan MM, Kang S. 2016. Stop and smell the fungi: fungal volatile metabolites are overlooked signals involved in fungal interaction with plants. Fungal Biol Rev. 30:134–144.
- Li N, Wang W, Bitas B, Subbarao K, Liu XKang S. 2018. Volatile compoundsemitted by diverse verticillium species enhance plant growth by manipulating auxin signaling. Molecular Plant-Microbe Interactions under revision.
- Liu W, Li R-J, Han -T-T, Cai W, Fu Z-W, Lu Y-T. 2015. Salt stress reduces root meristem size by nitric oxide-mediated modulation of auxin accumulation and signaling in Arabidopsis. Plant Physiol. 168:343–356.
- Marchant A. 1999. AUX1 regulates root gravitropism in Arabidopsis by facilitating auxin uptake within root apical tissues. Embo J. 18:2066–2073.
- Marchant A, Bhalerao R, Casimiro I, Eklöf J, Casero PJ, Bennett M, Sandberg G. 2002. AUX1 promotes lateral root formation by facilitating indole-3-acetic acid distribution between sink and source tissues in the Arabidopsis seedling. Plant Cell. 14:589–597.
- Mayak S, Tirosh T, Glick BR. 2004. Plant growth-promoting bacteria confer resistance in tomato plants to salt stress. Plant Physiol Biochem. 42:565–572.
- Metternicht G, Zinck J. 2003. Remote sensing of soil salinity: potentials and constraints. Remote Sens Environ. 85:1–20.
- Munns R, Tester M. 2008. Mechanisms of salinity tolerance. Annu Rev Plant Biol. 59:651–681.
- Naseem M, Kaltdorf M, Dandekar T. 2015. The nexus between growth and defence signaling: auxin and cytokinin modulate plant immune response pathways. J Exp Bot. 66:4885–4896.
- Naznin HA, Kiyohara D, Kimura M, Miyazawa M, Shimizu M, Hyakumachi M. 2014. Systemic resistance induced by volatile organic compounds emitted by plant growth-promoting fungi in Arabidopsis thaliana. PLoS One. 9:e86882.
- Negrão S, Schmöckel SM, Tester M. 2017. Evaluating physiological responses of plants to salinity stress. Ann Bot. 119:1–11.
- Netondo GW, Onyango JC, Beck E. 2004. Sorghum and salinity. Crop Sci. 44:806–811.
- Ortíz-Castro R, Contreras-Cornejo HA, Macías-Rodríguez L, López-Bucio J. 2009. The role of microbial signals in plant growth and development. Plant Signal Behav. 4:701–712.
- Paul D, Park KS. 2013. Identification of volatiles produced by Cladosporium cladosporioides CL-1, a fungal biocontrol agent that promotes plant growth. Sensors. 13:13969–13977.
- Piechulla B, Lemfack MC, Kai M. 2017. Effects of discrete bioactive microbial volatiles on plants and fungi. Plant Cell Environ. 40:2042–2067.
- Pieterse CMJ, Van der Does D, Zamioudis C, Leon-Reyes A, Van Wees SCM. 2012. Hormonal modulation of plant immunity. Annu Rev Cell Dev Biol. 28:489–521.
- Ryu C-M, Farag MA, Hu C-H, Reddy MS, Kloepper JW, Paré PW. 2004. Bacterial volatiles induce systemic resistance in Arabidopsis. Plant Physiol. 134:1017–1026.
- Shrivastava P, Kumar R. 2015. Soil salinity: A serious environmental issue and plant growth promoting bacteria as one of the tools for its alleviation. Saudi J Biol Sci. 22:123–131.
- Song GC, Ryu C-M. 2013. Two volatile organic compounds trigger plant self-defense against a bacterial pathogen and a sucking insect in cucumber under open field conditions. Int J Mol Sci. 14:9803–9819.
- Splivallo R, Fischer U, Göbel C, Feussner I, Karlovsky P. 2009. Truffles regulate plant root morphogenesis via the production of auxin and ethylene. Plant Physiol. 150:2018–2029.
- Ulmasov T, Murfett J, Hagen G, Guilfoyle TJ. 1997. Aux/IAA proteins repress expression of reporter genes containing natural and highly active synthetic auxin response elements. Plant Cell. 9:1963–1971.
- Vaishnav A, Jain S, Kasotia A, Kumari S, Gaur RK, Choudhary DK. 2013. Effect of nitric oxide signaling in bacterial-treated soybean plant under salt stress. Arch Microbiol. 195:571–577.
- Vaishnav A, Kumari S, Jain S, Varma A, Choudhary DK. 2015. Putative bacterial volatile-mediated growth in soybean (Glycine max L. Merrill) and expression of induced proteins under salt stress. J Appl Microbiol. 119:539–551.
- Vaishnav A, Kumari S, Jain S, Varma A, Tuteja N, Choudhary DK. 2016. PGPR-mediated expression of salt tolerance gene in soybean through volatiles under sodium nitroprusside. J Basic Microbiol. 56:1274–1288.
- Wang Y, Li K, Li X. 2009. Auxin redistribution modulates plastic development of root system architecture under salt stress in Arabidopsis thaliana. J Plant Physiol. 166:1637–1645.
- Xiong L, Zhu J-K. 2002. Salt tolerance. Arabidopsis Book. 1:e0048.
- Yang J, Kloepper JW, Ryu C-M. 2009. Rhizosphere bacteria help plants tolerate abiotic stress. Trends Plant Sci. 14:1–4.
- Zhang H, Kim M-S, Krishnamachari V, Payton P, Sun Y, Grimson M, Farag MA, Ryu C-M, Allen R, Melo IS, et al. 2007. Rhizobacterial volatile emissions regulate auxin homeostasis and cell expansion in Arabidopsis. Planta. 226:839–851.
- Zhang H, Kim M-S, Sun Y, Dowd SE, Shi H, Paré PW. 2008. Soil bacteria confer plant salt tolerance by tissue-specific regulation of the sodium transporter HKT1. Mol Plant Microbe Interact. 21:737–744.
- Zhang H, Murzello C, Sun Y, Kim M-S, Xie X, Jeter RM, Zak JC, Dowd SE, Paré PW. 2010. Choline and osmotic-stress tolerance induced in Arabidopsis by the soil microbe Bacillus subtilis (GB03). Mol Plant Microbe Interact. 23:1097–1104.