Abstract
A specialized complex, the tail, is the most common strategy employed by bacterial viruses to deliver their genome without disrupting cell integrity. T7 has a short, non-contractile tail formed by a tubular structure surrounded by fibers. Recent studies showed that incubation of the virus with Escherichia coli lipopolysaccharides (LPS) resulted in complete delivery of the viral genome, demonstrating for the first time that LPS are the T7 receptor. Further screening of the bacterial envelope for proteinaceous compounds that affect T7 ejection showed that porins OmpA and OmpF affect viral particle adsorption and infection kinetics, suggesting that these proteins play a role in the first steps of virus-host interaction. Comparison of the structures before and after ejection showed the conformational changes needed in the tail for genome delivery. Structural similarities between T7 and other viruses belonging to the Podoviridae family suggests that they could also follow a similar DNA ejection mechanism.
Abbreviations
EM | = | electron microscopy |
LPS | = | lipopolysaccharide |
OMP | = | Outer membrane protein. |
Introduction
The bacteriophage tail is the most common mechanism used by bacterial viruses to transport their genetic material to the bacterial cytoplasm.Citation1-3 Although the morphology of the tail structure can differ depending on the bacteriophage family (Siphoviridae, Myoviridae and Podoviridae) and the nature of the bacterial hosts, the structure of the tail machineries share several features. In most of the cases they consist in 6-folded symmetry structures composed by a central tubular assembly surrounded by fibers or spikes.Citation2,4 While the fibers or spikes are usually involved in bacterial receptor recognition, the central channel of the tubular structure is essential for nucleic acid delivery through bacterial membrane. The tail of T7 bacteriophage is a macromolecular complex of around ∼2 MDa and its structure and assembly were defined by cryo-EM and image processing.Citation5 The T7 tail components are sequentially assembled into the capsid during the virus morphogenesis after DNA packaging is completed. First, gp11 interacts with the gp8 dodecameric toroidal connector which builds the DNA translocating vertex of the capsid. Gp11 assembles on the connector as a dodecameric gatekeeper ring which, in turn, serves as the base for subsequent assembly of the hexameric nozzle built by gp12. This set-up prevents the release of DNA from the capsid, probably retained by the gatekeeper protein, and it further nucleates the assembly of the 6 tail fibers, each one being a trimer of gp17.
T7 infects Escherichia coli Gram-negative bacteria. The Gram-negative bacterial envelope is composed of a peptidoglycan layer surrounded by 2 membranes.Citation1,6 During infection, the phage is thought to interact first with a surface molecule that allows correct tail orientation relative to the bacterial envelope, followed by an irreversible interaction with the same or a different receptor; this second interaction is needed to trigger opening of the tail channel.Citation7 Viruses that infect Gram-negative bacteria, have been described to use lipopolysaccharide (LPS) as a receptor, often assisted by porins or outer membrane proteins.Citation8-10 Recently, González-García et al.Citation11 demonstrated that rough LPS are able to trigger the conformational changes needed to deliver the T7 viral genome. Before this work the identity of the T7 bacterial receptor was unknown. Although some studies had pointed out the capability of rough LPS to neutralize T7Citation8,12 their role was defined mainly as a primary phage receptor that mediated reversible binding to the bacterial surface, while another secondary receptor was suspected to be essential for irreversible binding and infection.Citation7,13 In their work, González-García et al.Citation11 used biochemical assays together with cryo-EM and image processing to characterize the T7 ejection reaction triggered by rough LPS. Their studies allowed to describe the conformational changes that take place in the tail complex during DNA exit and to hypothesize a possible schema of the early steps followed by the virus during infection. Here we screened the envelope of rough E. coli BL21 strain to search for a putative proteinaceous secondary receptor that could help in the initial fiber-LPS binding mechanism. The resulting work provides a more complete view of the T7 infection pathway that would help to understand the molecular mechanism involved in T7 genome delivery.
Lipopolysaccharides act as the main T7 receptor in vitro
The outer membrane of Gram-negative bacteria presents a conspicuous component known as lipopolysaccharides (LPS), which play a key role in the interaction of bacteria with the environment. LPS are large polymeric polysaccharide chains covalently linked to a specific lipid and they present an overall hydrophobic nature. It has been proposed since a long time that certain bacteriophages might interact with a subclass of LPS known as rough LPS, which are lacking the outermost O antigen domain.Citation8,14 Gonzalez-García et al.Citation11 carried out the incubation of T7 with purified rough LPS, and found that the virions were inactivated. Analysis by electron microscopy of T7 incubated with rough LPS revealed that the viral particles had lost the packaged DNA, and they were empty (), while the incubation with smooth LPS did not affect the DNA content of T7 particles.Citation11 These results suggested that the inactivation of T7 was due to the release of DNA from the virus capsid triggered by the interaction of the T7 particle with purified rough LPS.
Figure 1. (A) Cryo-EM micrograph showing T7 phages attached to LPS layers during the ejection reaction.Citation11 (B) Denaturing gel electrophoresis of T7 phages before (right) and after (left) incubation with rough LPS.Citation11
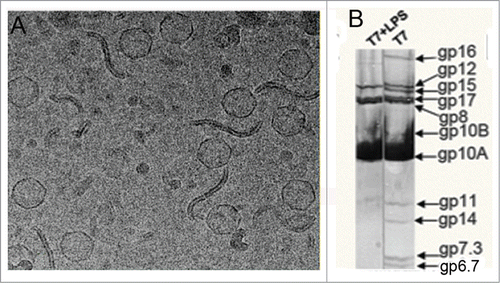
The production of empty T7 particles after incubation with rough LPS also suggested that the release of the DNA was accompanied by the disappearance of the core proteins, which in the prohead and the mature capsid build a prominent complex in the interior of the capsid.Citation15 These observations were supported by the electrophoretic analysis of the proteins present in T7 particles. The core proteins (gp14, gp15 and gp16), together with the small structural proteins gp7.3, gp6.7 and gp13, were lost from the particles after rough LPS interaction (), suggesting that the triggering of the DNA release caused the core to disassemble and the core proteins to be proteolyzed.Citation11
The simultaneous release of DNA and disappearance of the core proteins from the T7 viral particles is characteristic of the infection process in vivo.Citation16 The fact that these processes also take place after interaction of T7 viral particles with purified rough LPS in vitro suggests that this single interaction is able to mimic the main steps of the DNA ejection process during T7 infection of E. coli. Based on these assumptions, Gonzalez-Garcia et al.Citation11 performed a series of in vitro assays to follow DNA ejection rates, and to determine the virus-LPS interaction constant, as well as the opening of the DNA release mechanism (kopen). The kopen value of 4.2 × 104 × s−1, was found to be of the same order of magnitude as previously reported for podovirus P22 (kopen 4.5 × 104 × s−1).Citation17 It is interesting to note that the slower Podoviridae DNA release kinetics, as compared with other virus of the Siphoviridae family,Citation17 might be caused by the simultaneous translocation of the internal core proteins together with the DNA in the case of Podoviridae.
LPS triggers conformational changes in the T7 tail allowing DNA release
The viral component responsible for genome transfer into the infected bacteria is the tail, which is attached to the capsid through the connector vertex.Citation4,18 T7 tail is composed by 3 major proteins (gp11, gp12 and gp17). Cuervo et al.Citation5 performed a detailed analysis of the structure and assembly of T7 tail using cryo-electron microscopy and 3-dimensional reconstruction at 1.2–1.6 nm resolution. This infection machinery is representative of the main features of the Podoviridae tailsCitation19 in the conformation that keeps the DNA inside the virus capsid ().
Figure 2. (A) Side view and (B) end-on view of the 3-dimensional reconstruction of the T7 tail before (left, EMD-5689Citation5) and after (right, EMD-2717Citation11) DNA ejection at ˜20 Å resolution. The capsid, connector and gatekeeper were colored in gray while components playing the main conformational changes during DNA ejection, the fibers and the nozzle were colored in blue and multiple colors, respectively.
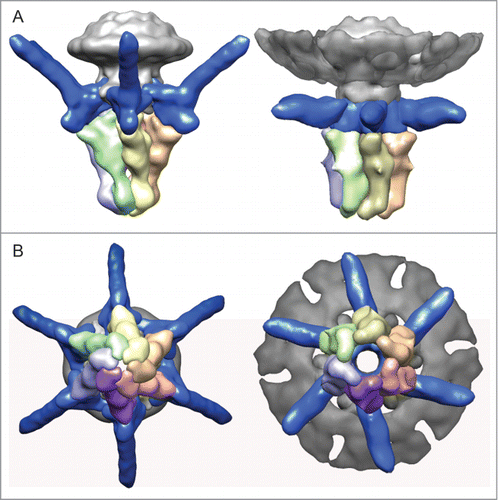
As described above, the interaction of rough LPS with T7 particles leads to in vitro DNA ejection, and this offers a simple model system amenable for structural analysis. Using this assay, González-Garcia et al.Citation11 studied the tail complex of viruses after DNA delivery using cryo-electron microscopy and 3-dimensional reconstruction (). The post-ejected tail structure at ∼20 Å resolution showed similar characteristic features of the T7 tail in the pre-ejection stateCitation5: the connector, embedded into the capsid, interacts directly with the gp11 gatekeeper, and the gp17 N-terminal domain of the fibers is attached to the interface between the gatekeeper and the hexameric nozzle. These similarities allowed segmentation of the different protein components in the post-ejected conformation despite the lower resolution.
The comparison of the 2 structures of the tail corresponding to the pre- and post-DNA ejection conformation shows the major structural rearrangements involved in the DNA delivery process. The more evident is the change in the fiber orientation respect to the tail axis. While the fibers in the pre-ejection state are assembled with a 60° angle respect to the tail axis, they are almost perpendicular in the post-ejection state. The existence of a change in the orientation of the fibers was already observed in cryo-tomographic reconstructions of T7 interacting with bacterial envelopes.Citation20 Although the C-terminal end of the fibers is not present in our reconstructions due to its intrinsic conformational variability, it is evident that the 30° bending of the amino-terminal domain of the fiber drives the C-terminal of the fiber downwards from its upward position to contact the LPS (or bacterial) surface, thus offering the possibility for the tip of the C-terminus to interact with the proper receptor sites in a permanent way.Citation21 Another consequence of the fiber bending is the change in the domain corresponding to the contact area with gp11 and gp12 complexes. Actually, the fiber bending takes place as a rigid body movement that produces the inner tail channel opening in that area, which is the point where the DNA end is halted in the pre-ejection state ( in Gonzalez-Garcia et al.Citation11).
Figure 3. (A) analysis of T7 infection of E. coli WT and porine-defective mutant strain. The image shows lysis plaques obtained after plating T7 bacteriophage with E. coli WT (left) and mutant ΔlamB ΔompF::Tn5 ΔompA ΔompC (37) (right) at a moi of 3. (B) One-step growth of phage T7 on E. coli WT (solid line) and porine-defective E. coli mutant (dashed line). Titer was tested every 10 min; the inset shows the same experiment tested every 2.5 min. (C) Adsorption of viruses to the bacterial surface. Micrographs of 2% (w/v) PTA negatively-stained samples showing T7 particles incubated with E. coli WT bacteria (left) and porine-lacking mutant (right) at a moi of 100 for 7.5 min. The arrow indicates an emptied virus.
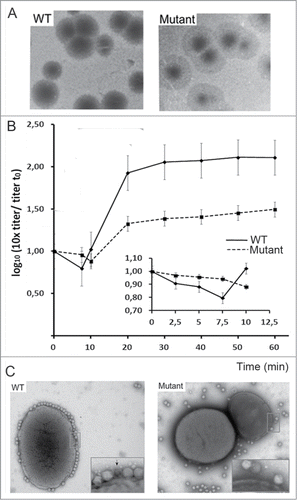
Figure 4. (A) Adsorption of phage T7 to immobilized cell wall proteins by the virus overlay protein binding assay (VOPBA). E. coli vesicles were obtained after osmotic shock and Frech preasure cell (Thermo Scientific) treatment to the bacteria, the pellet of the cellular debris was then purified in a 60% (w/v) sucrose cushion. Membrane proteins were solubilized from E. coli vesicles in 1.25% (w/v) N-dodecyl β-d-maltoside. VOPBA was performed as describedCitation23 with some modifications. Solubilized membrane proteins were loaded in a 5.5% native polyacrylamide gel and stained with Coomassie blue or electroblotted (left). Nitrocellulose membranes were incubated alone (control) or with 5 ml of 1011 p.f.u./ml T7 particles, then incubated with anti-gp10 capsid protein antibody, and developed with an enhanced chemilumiscence kit (ECL, GE Healthcare). The band for the reactive protein complex in native conditions was subjected to a second-dimension 17% SDS-PAGE in denaturing conditions and treated as above. (B) The 2 reactive bands were identified by mass spectrometry as OmpA and OmpF as described in . Protein lenght of OmpA (up) and OmpF (bottom) are represented with matched parts depicted in gray.
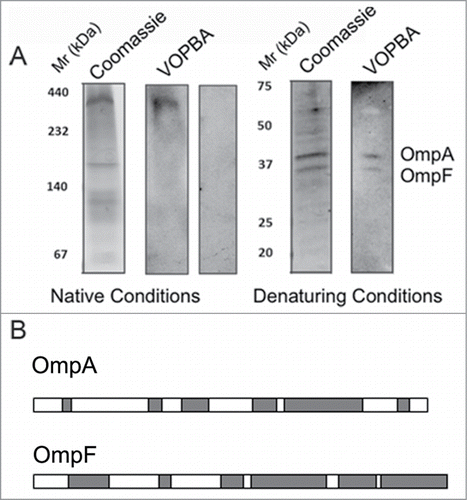
Table 1. Mass spectrometric analisys results of VOPBA detected gel bandsFootnotea
Another main structural difference related to the ejection of the DNA is the change in the gp12 nozzle. The structure in the post-ejection state is wider in the terminal end. The movement of each gp12 subunit follows also a rigid body 10° shift and bending around the axis of the gp12 apical lobe domain to adopt a more vertical orientation and, as a consequence of the concerted movement of the 6 gp12 subunits, this lead to the full opening of the inner channel ().
Altogether the results obtained by González-García et al. allowed to propose a model for DNA delivery. The fibers in close contact to the head would change to a downwards conformation after interaction with the LPS. The fact that the nozzle channel opening occurs concomitant to the opening in the gatekeeper-fiber interface suggests that both structural changes might be coupled someway to assure the acquisition of the open conformation required for DNA translocation. It is tempting to suggest that the interaction of tail fibers with rough LPS triggers the opening of the valve at the gatekeeper level, which in turn liberates DNA from the packaged state. As a result of the DNA release along the tail channel, the nozzle subunits change their conformation opening the channel to allow the full DNA translocation from the viral capsid.Citation11
OmpA and OmpF porins participate in early steps of bacteriophage T7-host interaction
In the T7 infection model proposed in our previous workCitation11 the first interaction of the virus with the bacterial outer membrane remained unknown. While it was clear that LPS acted as the main T7 receptor in vitro triggering the conformational change of the fibers and the nozzle we could not define how the fibers that are found attached to the head in the mature virusCitation20 are able to interact with the LPS layer. Studies carried on bacteriophages belonging also to the Podoviridae family suggests that porine membrane proteins may be involved together with LPS in bacteriophage infection: Yep-phi specifically recognizes OmpF and Ail porins, and uses LPS from rough Yersinia pestis strains as a receptor.Citation10
In an attempt to check whether other components of the bacterial envelope, specially porins, had a role in the interaction of T7 with E. coli, we carried out new biochemical experiments. As a mechanism similar to Yep-phi bacteriophage could be expected for T7, to explore T7 infection requirement for porins we used an E. coli triple mutant lacking OmpA, OmpF and OmpCCitation22 to perform phage adsorption assays. The formation of lysis plaques and recovery of viable phages from them indicated that the virus was able to infect that highly modified bacteria and, consequently, that porins are not essential for T7 infection (). However, comparison of the lysis plaques from wild type (WT) and mutant showed a decrease in plaque size and formation of a surrounding opaque halo around the plaques in the assays using E. coli porin defective mutant (), suggesting that OmpA, OmpF or OmpC porin proteins might play some role that affects the kinetics of viral infection. To further study the role of these porins in T7 infection kinectics we follow one-step growth curves for up to 60 min. In these assays, E. coli WT and porine defective mutant strains were infected by T7 viruses at a moi of 3 and plated (). The early part of the curve showed a decrease in infective virus while the genome was internalized into the bacteria, followed by an exponential increase due to the newly formed progeny. In WT bacteria, the minimum viral titer was observed at 7.5 min post-infection (, black line), whereas the porin-lacking mutant showed a smaller drop in viral titer, which only reached a minimum at 10 min post-infection (, dotted line). This delayed and smaller infection level resulted in a ∼10-fold lower titer for phages that infected the mutant as compared to WT bacteria. Altogether these results suggest that porin proteins might act as helpers during early interaction steps, facilitating viral adsorption. We used electron microscopy (EM) to test phage adsorption to the cells in negatively-stained samples. T7 viruses were incubated with WT and mutant E. coli strains at a moi of 100 for 7.5 minutes. While WT E. coli was fully covered by T7 phages, mutant bacteria had a much smaller number of phages attached to the bacterial surface at the same incubation time (). Although we can not rule out the possibility that the absence of porins affects the membrane difficulting viral interaction, our results point the possibility that some porins act as a secondary viral receptor during T7 infection.
In order to specifically determine the protein component in the E. coli membrane capable to interact with T7 bacteriophage we used virus overlay protein binding assays (VOPBA).Citation23 T7 infection could be triggered by using E. coli vesicles (data not shown), indicating that these samples contain the T7 receptor. Membrane proteins solubilized from these E. coli vesicles were resolved on a native acrylamide gel, transferred to a nitrocellulose membrane and incubated with bacteriophage T7, followed by detection with anti-gp10 T7 antibody. One band was specifically recognized by T7 as it was not detected in the control incubated without virus (). The reactive band was then loaded on a second gel under denaturing conditions, followed by VOPBA, and 2 reactive bands around ∼37 kDa were detected (). These two bands were cut away from the gel and subjected to mass spectrometry analysis. They were determined to correspond to the outer membrane proteins OmpA and OmpF ( and ). These data are consistent with previously published experiments in which OmpA and OmpF proteins were recovered from T7 interacting vesicles.Citation24 The interaction of T7 with OmpA and OmpF, even under denaturing conditions, suggests that the virus recognition occurs through linear epitopes of these membrane proteins. These results suggest that porins (specifically OmpA and OmpF) act as host-interacting proteins, playing some accessory role in the early events in T7 infection. However, as the virus is able to infect the porin-lacking mutant, these proteins are non-essential for infection, but they rather increase the speed of the DNA ejection reaction, probably by aiding someway the viral adsorption process to the bacterial surface.
Discussion
Phages and their host bacteria are in a constant evolutive interaction in which receptor recognition is a key factor for a successful viral infection. One strategy to increase the host range is to recognize more than one bacterial receptor. Mutations in viral genes that encode for receptor-binding proteins (RBP) can give rise to new interactions between the phage and the surface of the host bacteria, as previously reported for phage Ox2Citation25 and phage lambda.Citation26 González-García et al.Citation11 showed that LPS from rough E. coli strains are sufficient to trigger the release of the viral genome in vitro. Nevertheless it remained unclear how the C-terminal domain of the fibers that is interacting with the viral head could get in contact with the LPS bacterial receptor. This prompted us to search for additional components which might account for the receptor. It is well-known that LPS interact very tightly with proteins of the outer membrane,Citation27 and inner core sugars from LPS, the region that seems to be recognized by T7 particles,Citation28 are implicated in the assembly and maintenance of OmpA and OmpF proteins in the outer membrane.Citation29,30 These results, together with the identification of porin proteins as receptors for other members of the Podoviridae family, suggested that porin proteins act as secondary receptor possible candidates. In the present study we have observed differences concerning morphology of the plaques and intracellular growth curves between WT and porin-lacking E. coli strains suggesting that porins are involved in T7 adsorption and we have identified that OmpA and OmpF interact specifically with T7 viral particles using VOPBA assays. Furthermore, alignment of the sequences of T7 and Yep-phi fiber tip domain revealed a 38% of identity.Citation10 Since Yep-phi fibers specifically recognize rough LPS and outer membrane proteins (OmpF and Ail) on the surface of Yersinia pestis, we suggest that T7 fibers are also involved in the specific recognition of OmpA and OmpF proteins. Nevertheless, the fact that bacteria lacking these 2 porins are infected by T7, although at a much smaller rate than WT bacteria, suggests that LPS are the main T7 bacterial receptor and we hypothesized that these porins could then act as primary receptors, guiding viral particles toward the LPS core sugars.
Besides the biochemical characterization of the T7 receptor, González-García et al.Citation11 analyzed also in their previous work the conformational changes needed in the T7 tail structure to deliver the viral DNA. Comparison of the reconstructions of T7 tail complex before and after DNA ejection revealed a drastic change in fibers conformation, together with the opening of the internal tail channel up to 40 Å in overall diameter. The electrophoretic analysis of post-ejection particles suggest that core proteins are also ejected through this tail channel. These results are in concordance with the previous visualization of an extended tail in T7 particles attached to the E. coli envelope by cryo-electron tomography.Citation20 Hu and collaborators propose that T7 core proteins would be ejected to form a conduit across the bacterial membranes allowing the safe internalization of viral DNA into the bacterial cytoplasm. Later on, the core channel would be disassembled or degraded to restore inner membrane integrity.Citation11,20
Analysis of the available 3D models for phages of T7 group previously evidenced a structural conservation for tail nozzle proteins,Citation19 suggesting that this mechanism of DNA delivery could be extended to other members of the Podoviridae family. In fact, similar conformational changes in the fiber orientation and opening of the tail nozzle were also described for marine podovirus P-SSP7.Citation31 A close observation of the fiber-docking region in T7, K1E, P-SSP7 and ϵ15 revealed that the first DNA segment to be ejected is trapped in the tail conduit at the gatekeeper-fiber level (). This finding suggests that the conformational changes in the fibers, induced by their interaction with the receptor (LPS for T7), are transduced to the gatekeeper-fiber interface triggering the opening signal to the gatekeeper proteins that retain the DNA. The force of the released DNA could participate in the untwist of nozzle proteins and, as a consequence, the internal channel would open allowing the ejection of the viral DNA and the core proteins.
Figure 5. Cross section of the 3-dimensional volumes of T7 (EMD-5689Citation15), K1E (EMD-1333Citation32), P-SSP7 (EMD-1715Citation31) and ϵ15 (EMD-1175Citation33). The internal channel DNA fragment has been colored in yellow. The arrow points to the fiber-gatekeeper interface region.
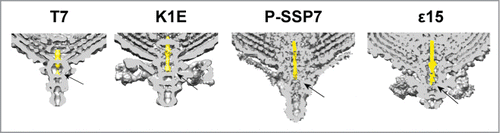
Disclosure of Potential Conflicts of Interest
No potential conflicts of interest were disclosed.
Acknowledgements
Authors thank Carmela Garcia-Doval and Mark J van Raaij for their previous contribution to this work. We are indebted to Luis Ángel Fernández for kindly providing bacterial strains. Authors thank Mónica García-Gallo, Mercedes Llorente and Leonor Kremer at the Protein Tools Unit facility at the CNB for gp10 antibody production and Sergio Ciordia at CNB Proteomics Service for peptide mass fingerprinting by MALDI TOF MS analysis.
Funding
This work was supported by grants BFU2011-29038 (to JLC) and BFU2011-25090 (to JM-B) from the Ministry of Economy and Competitiveness.
References
- Vinga I, Sao-José C, Tavares P, Santos M. Bacteriophage entry in the host cell. In: Modern Bacteriophage Biology and Biotechnology. ed. Wegrzyn G. Kerala, India: Research Signpost; 2006.
- Veesler D, Cambillau C. A common evolutionary origin for tailed-bacteriophage functional modules and bacterial machineries. Microbiol Mol Biol Rev 2011; 75:423-33; PMID:21885679; http://dx.doi.org/10.1128/MMBR.00014-11
- Hershey AD, Chase M. Independent functions of viral protein and nucleic acid in growth of bacteriophage. J Gen Physiol 1952; 36:39-56; PMID:12981234; http://dx.doi.org/10.1085/jgp.36.1.39
- Cuervo A, Carrascosa JL. Bacteriophages: Structure. eLS. Chichester. UK: John Wiley & Sons, Ltd; 2012.
- Cuervo A, Pulido-Cid M, Chagoyen M, Arranz R, Gonzalez-Garcia VA, Garcia-Doval C, Caston JR, Valpuesta JM, van Raaij MJ, Martin-Benito J, et al. Structural characterization of the bacteriophage t7 tail machinery. J Biol Chem 2013; 288:26290-9; PMID:23884409; http://dx.doi.org/10.1074/jbc.M113.491209
- Silhavy TJ, Kahne D, Walker S. The bacterial cell envelope. Cold Spring Harb Perspect Biol 2010; 2:a000414; PMID:20452953; http://dx.doi.org/10.1101/cshperspect.a000414
- Casjens SR, Molineux IJ. Short noncontractile tail machines: adsorption and DNA delivery by podoviruses. Adv Exp Med Biol 2012; 726:143-79; PMID:22297513
- Lindberg AA. Bacteriophage receptors. Ann Rev Microbiol 1973; 27:205-41; PMID:4584686; http://dx.doi.org/10.1146/annurev.mi.27.100173.001225
- Parent KN, Erb ML, Cardone G, Nguyen K, Gilcrease EB, Porcek NB, Pogliano J, Baker TS, Casjens SR. OmpA and OmpC are critical host factors for bacteriophage Sf6 entry in Shigella. Mol Microbiol 2014; 92:47-60; PMID:24673644; http://dx.doi.org/10.1111/mmi.12536
- Zhao X, Cui Y, Yan Y, Du Z, Tan Y, Yang H, Bi Y, Zhang P, Zhou L, Zhou D, et al. Outer membrane proteins ail and OmpF of Yersinia pestis are involved in the adsorption of T7-related bacteriophage Yep-phi. J Virol 2013; 87:12260-9; PMID:24006436; http://dx.doi.org/10.1128/JVI.01948-13
- Gonzalez-Garcia VA, Pulido-Cid M, Garcia-Doval C, Bocanegra R, van Raaij MJ, Martin-Benito J, Cuervo A, Carrascosa JL. Conformational Changes Leading To T7 DNA Delivery Upon Interaction With The Bacterial Receptor. J Biol Chem 2015; 290:10038-44; PMID:25697363
- Kruger DH, Schroeder C. Bacteriophage T3 and bacteriophage T7 virus-host cell interactions. Microbiol Rev 1981; 45:9-51; PMID:6261110
- Molineux IJ. No syringes please, ejection of phage T7 DNA from the virion is enzyme driven. Mol Microbiol 2001; 40:1-8; PMID:11298271; http://dx.doi.org/10.1046/j.1365-2958.2001.02357.x
- Koike M, Iida K. Effect of polymyxin on the bacteriophage receptors of the cell walls of gram-negative bacteria. J Bacteriol 1971; 108:1402-11; PMID:4109866
- Agirrezabala X, Martin-Benito J, Caston JR, Miranda R, Valpuesta JM, Carrascosa JL. Maturation of phage T7 involves structural modification of both shell and inner core components. EMBO J 2005; 24:3820-9; PMID:16211007; http://dx.doi.org/10.1038/sj.emboj.7600840
- Kemp P, Garcia LR, Molineux IJ. Changes in bacteriophage T7 virion structure at the initiation of infection. Virology 2005; 340:307-17; PMID:16054667; http://dx.doi.org/10.1016/j.virol.2005.06.039
- Andres D, Roske Y, Doering C, Heinemann U, Seckler R, Barbirz S. Tail morphology controls DNA release in two Salmonella phages with one lipopolysaccharide receptor recognition system. Mol Microbiol 2012; 83:1244-53; PMID:22364412; http://dx.doi.org/10.1111/j.1365-2958.2012.08006.x
- Bhardwaj A, Olia AS, Cingolani G. Architecture of viral genome-delivery molecular machines. Curr Opin Struct Biol 2014; 25:1-8; PMID:24878339; http://dx.doi.org/10.1016/j.sbi.2013.10.005
- Cuervo A, Chagoyen M, Pulido-Cid M, Camacho A, Carrascosa JL. Structural characterization of T7 tail machinery reveals a conserved tubular structure among other Podoviridae family members and suggests a common mechanism for DNA delivery. Bacteriophage 2013; 3:e27011; http://dx.doi.org/10.4161/bact.27011
- Hu B, Margolin W, Molineux IJ, Liu J. The bacteriophage t7 virion undergoes extensive structural remodeling during infection. Science 2013; 339:576-9; PMID:23306440; http://dx.doi.org/10.1126/science.1231887
- Garcia-Doval C, van Raaij MJ. Crystallization of the C-terminal domain of the bacteriophage T7 fibre protein gp17. Acta Crystallogr Sect F Struct Biol Cryst Commun 2012; 68:166-71; PMID:22297990; http://dx.doi.org/10.1107/S1744309111051049
- Prilipov A, Phale PS, Van Gelder P, Rosenbusch JP, Koebnik R. Coupling site-directed mutagenesis with high-level expression: large scale production of mutant porins from E. coli. FEMS Microbiol Lett 1998; 163:65-72; PMID:9631547; http://dx.doi.org/10.1111/j.1574-6968.1998.tb13027.x
- Puig A, Araujo R, Jofre J, Frias-Lopez J. Identification of cell wall proteins of Bacteroides fragilis to which bacteriophage B40-8 binds specifically. Microbiology 2001; 147:281-8; PMID:11158345
- Serwer P, Wright ET, Hakala KW, Weintraub ST. Evidence for bacteriophage T7 tail extension during DNA injection. BMC Res Notes 2008; 1:36; PMID:18710489; http://dx.doi.org/10.1186/1756-0500-1-36
- Drexler K, Dannull J, Hindennach I, Mutschler B, Henning U. Single mutations in a gene for a tail fiber component of an Escherichia coli phage can cause an extension from a protein to a carbohydrate as a receptor. J Mol Biol 1991; 219:655-63; PMID:1829115; http://dx.doi.org/10.1016/0022-2836(91)90662-P
- Meyer JR, Dobias DT, Weitz JS, Barrick JE, Quick RT, Lenski RE. Repeatability and contingency in the evolution of a key innovation in phage lambda. Science 2012; 335:428-32; PMID:22282803; http://dx.doi.org/10.1126/science.1214449
- Rocque WJ, Coughlin RT, McGroarty EJ. Lipopolysaccharide tightly bound to porin monomers and trimers from Escherichia coli K-12. J Bacteriol 1987; 169:4003-10; PMID:2442135
- Qimron U, Marintcheva B, Tabor S, Richardson CC. Genomewide screens for Escherichia coli genes affecting growth of T7 bacteriophage. Proc Natl Acad Sci U S A 2006; 103:19039-44; PMID:17135349; http://dx.doi.org/10.1073/pnas.0609428103
- Ried G, Hindennach I, Henning U. Role of lipopolysaccharide in assembly of Escherichia coli outer membrane proteins OmpA, OmpC, and OmpF. J Bacteriol 1990; 172:6048-53; PMID:2170338
- Beher MG, Schnaitman CA. Regulation of the OmpA outer membrane protein of Escherichia coli. J Bacteriol 1981; 147:972-85; PMID:7024253
- Liu X, Zhang Q, Murata K, Baker ML, Sullivan MB, Fu C, Dougherty MT, Schmid MF, Osburne MS, Chisholm SW, et al. Structural changes in a marine podovirus associated with release of its genome into Prochlorococcus. Nat Struct Mol Biol 2010; 17:830-6; PMID:20543830; http://dx.doi.org/10.1038/nsmb.1823
- Leiman PG, Battisti AJ, Bowman VD, Stummeyer K, Muhlenhoff M, Gerardy-Schahn R, Scholl D, Molineux IJ. The structures of bacteriophages K1E and K1-5 explain processive degradation of polysaccharide capsules and evolution of new host specificities. J Mol Biol 2007; 371:836-49; PMID:17585937; http://dx.doi.org/10.1016/j.jmb.2007.05.083
- Jiang W, Chang J, Jakana J, Weigele P, King J, Chiu W. Structure of epsilon15 bacteriophage reveals genome organization and DNA packaging/injection apparatus. Nature 2006; 439:612-6; PMID:16452981; http://dx.doi.org/10.1038/nature04487