ABSTRACT
As a mechanically condensed product of Coleman fat, extracellular matrix/stromal vascular fraction gel (ECM/SVF-gel) eliminates adipocytes, concentrates SVF cells, and improves fat graft retention. This study aims to compare SVF cell composition between Coleman fat and ECM/SVF-gel. Matched Coleman fat and ECM/SVF-gel of 28 healthy women were subjected to RNA-seq, followed by functional enrichment and cell-type-specific enrichment analyses, and deconvolution of SVF cell subsets, reconstructing SVF cell composition in the transcriptome level. ECM/SVF-gels had 9 upregulated and 73 downregulated differentially expressed genes (DEGs). Downregulated DEGs were mainly associated with inflammatory and immune responses, and enriched in fat macrophages. M2 macrophages, resting CD4+ memory T cells, M1 macrophages, resting mast cells, and M0 macrophages ranked in the top five most prevalent immune cells in the two groups. The proportions of the principal non-immune cells (e.g., adipose-derived stem cells, pericytes, preadipocytes, microvascular endothelial cells) had no statistical differences between the two groups. Our findings reveal ECM/SVF-gels share the same dominant immune cells beneficial to fat graft survival with Coleman fat, but exhibiting obvious losses of immune cells (especially macrophages), while non-immune cells necessary for adipose regeneration might have no significant loss in ECM/SVF-gels and their biological effects could be markedly enhanced by the ECM/SVF-gel’s condensed nature.
1. Introduction
Autologous fat grafting has become very prevalent in tissue reconstruction and aesthetic surgery. The Coleman fat obtained by concentrating the fat portion of the lipoaspirate has been adopted by aesthetic surgeons around the world [Citation1–3]. However, mature adipocytes easily undergo necrosis under hypoxia after fat grafting, which induces chronic inflammation and resultant oil cysts and calcification [Citation4,Citation5]. In contrast, most adipose-derived stem cells (ADSCs) can survive under hypoxic conditions and be activated, contributing to adipose regeneration. Yao et al. further mechanically processed and condensed Coleman fat into an injectable extracellular matrix/stromal vascular fraction gel (ECM/SVF-gel) by eliminating most of the mature adipocytes and oil portion, and maximizing enrichment of SVF cells and native adipose ECM [Citation6]. ECM/SVF-gels are highly condensed in volume and have greatly increased density of ADSCs and vascular endothelial cells [Citation6]. ECM/SVF-gel preparation does not require enzymes and other chemical reagents, which decreases the risk of both exogenous material and biological contamination.
The nude mouse Coleman fat/SVF-gel graft model has demonstrated that Coleman fat grafts have large areas of necrosis in the centre, numerous large oil cysts, and marked fibrosis, whereas ECM/SVF-gel grafts have no significant necrosis, oil cysts, and fibrosis, and exhibit a better long-term volume retention rate and greater adipose tissue integrity, with a retention volume more than 80% of the initial injection volume 90 days after grafting [Citation7]. Clinically, ECM/SVF-gel grafting, compared with transplantation of Coleman fat, botulinum toxin A, or nanofat combined with high-density fat, shows fewer complications and long-term therapeutic efficacy in volume augmentation and facial or neck rejuvenation [Citation8–11]. As a natural biomaterial, ECM/SVF-gels provide a valuable alternative to stem cell-based therapy for clinical translation.
SVF cells are the key elements for fat grafting success. As a heterogeneous cell population comprised of ADSCs, preadipocytes, endothelial cells, endothelial progenitor cells, smooth muscle cells, pericytes, fibroblasts, and a variety of immune cells [Citation12,Citation13], SVF cells have been demonstrated to have proangiogenic, proadipogenic, and antiinflammatory properties [Citation14–16]. The ECM niche of ECM/SVF-gels provides a beneficial scaffold for SVF cells to survive and exert their biological function. Flow cytometry shows that condensed ECM/SVF-gels contain higher SVF cell density, as compared with Coleman fat, despite that the total live cell number of SVF cells decreases due to cell death and loss during mechanical processing of the ECM/SVF-gel [Citation6,Citation17]. Previous studies [Citation6,Citation17] usually used flow cytometry, only according to cellular surface marker expression, to compare the SVF cell content between the two groups, and mainly focused on ADSCs, endothelial cells, pericytes, and CD45+ haematopoietic cells, and also did not further identify immune cell subsets. In order to better understand the cellular basis underlying favourable efficacy of ECM/SVF-gels in regenerative medicine, this study uses bioinformatics to more fully portray and compare the SVF cell composition and cell-type-specific gene expression between Coleman fat and ECM/SVF-gels. We performed bulk RNA-seq in Coleman fat and ECM/SVF-gels, followed by function enrichment analysis and tissue-cell-type specific enrichment analysis of differentially expressed genes (DEGs), and used deconvolution algorithms (CIBERSORT and xCell) to further identify cell types and quantify relative cell proportions based on the gene expression profiles.
2. Results
2.1. Comparison of transcriptional signals between Coleman fat and ECM/SVF-gels
In this study, we prepared matched Coleman fat and ECM/SVF-gels from lipoaspirates of 28 healthy women (), performed bulk RNA-seq on Coleman fat and ECM/SVF-gels, and compared the transcriptional signals between these two groups (Supplemental Table S1). We identified 82 DEGs (fold change in expression > 2 and FDR < 0.05) in the ECM/SVF-gel group, including 9 upregulated (LINC00987, LINC00840, LINC02367, MTCO3P12, NDUFC2, RPS26P15, RPS26P3, SCN3A, SNORD14A) and 73 downregulated genes ( and Supplemental Table S2). Among the 9 upregulated DEGs, only NDUFC2 and SCN3A were protein-coding RNAs, the remaining genes being long noncoding RNAs, pseudogenes, and small nucleolar RNAs. 73 downregulated DEGs included 71 protein-coding RNAs and 2 noncoding RNAs. Because ECM/SVF-gels are a purely mechanically processed and condensed product of Coleman fat, accompanied by different extents of cell death and loss of different SVF cell types, the gene expression differences between the two groups before grafting were mainly caused by their cell-type proportion variances. We only focused on the 73 downregulated DEGs. In order to explore the possible cell types mainly responsible for downregulated DEGs, the 73 downregulated DEGs were subjected to Gene Ontology (GO) functional enrichment analysis for biological process. The top 20 biological processes identified by the DAVID database, and the top 20 biological processes identified by the Metascape platform were mainly involved in inflammatory response and immune response (). These enrichment analysis results indicate immune cells might have undergone greater loss than other types of SVF cells during ECM/SVF-gel preparation, and thus were the principal cells accounting for downregulated DEGs. Single-cell RNA-seq (scRNA-seq) technology has successfully depicted cellular heterogeneity in human tissue. Although scRNA-seq was not conducted in this study, we used the web-based cell-type-specific enrichment analysis (WebCSEA) platform integrating scRNA-seq data [Citation18] to explore the tissue- and cell-type-specificity of the 73 downregulated DEGs according to tissue-cell-type signature genes (). In descending order, the top 3 significantly enriched tissue-cell types were identified as vasculature macrophages, muscle macrophages, and fat macrophages. WebCSEA results, combined with the presence of M2 macrophage-specific surface markers (CD163 and CD206 [Citation19–21]), rather than those of M1 macrophages (CD80, CD86, TLR2 and TLR4 [Citation22]), in the 73 downregulated DEGs, suggested the loss of macrophages, especially M2 macrophages, might be predominant in the process of making an ECM/SVF-gel.
Figure 1. Preparation process of Coleman fat and ECM/SVF gels. ECM/SVF-gels are highly condensed in volume compared with Coleman fat. The final volume of the ECM/SVF gel was about one-quarter that of the Coleman fat.
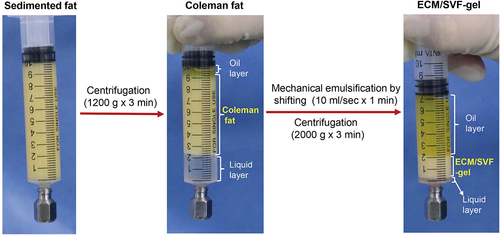
Figure 2. Volcano plot for DEGs, between Coleman fat and ECM/SVF-gels, revealing 9 upregulated and 73 downregulated DEGs in the ECM/SVF-gel. Each dot represents a detected gene; blue dots denote downregulated DEGs, and red dots represent upregulated DEGs. DEGs, differentially expressed genes.
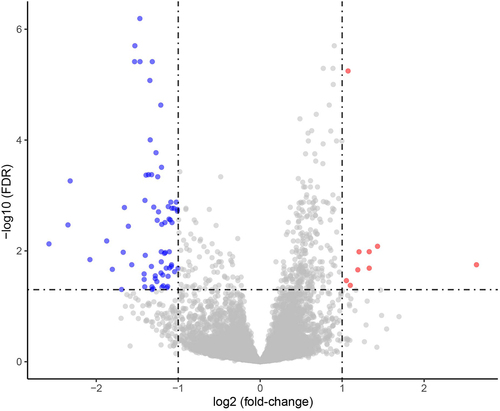
Figure 3. Gene Ontology (GO) functional enrichment analysis of 73 downregulated DEGs performed using the DAVID database (A) and Metascape platform (B), revealing enrichment of biological processes mainly associated with inflammatory response and immune response. DEGs, differentially expressed genes.

Figure 4. Web-based cell-type-specific enrichment analysis (WebCSEA) of 73 downregulated DEGs, revealing vasculature macrophages, muscle macrophages, and fat macrophages as the top 3 significantly enriched tissue-cell types. The X-axis represents 11 human organ systems. Y-axis indicates the – log10 (raw p-value) for each tissue-cell type from WebCSEA result. Each dot represents one tissue-cell type in the group on the X-axis differentiated by colour. The most significant dots are highlighted and annotated with their corresponding tissue-cell types. The red dashed line indicates the Bonferroni-corrected significance (p = 3.69 × 10−5) by 1355 tissue-cell types. The grey solid line indicates the nominal significance (p = 1 × 10−3). DEGs, differentially expressed genes.
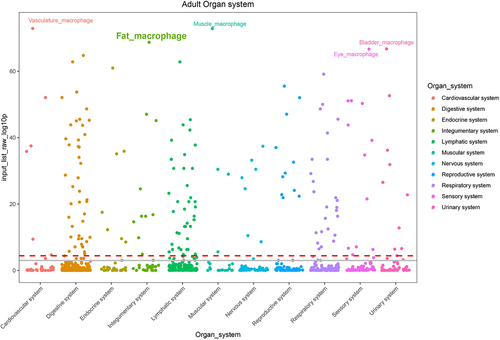
We further performed gene set enrichment analysis (GSEA) on whole gene expression profiles to identify hallmark gene sets enriched in Coleman fat and ECM/SVF-gels. Only two gene sets, involving interferon (IFN)-α response and IFN-γ response, showed a normalized enrichment score (NES) higher than 2.0 (FDR <0.05) and were enriched in ECM/SVF-gels (), suggesting that the cells involved in these responses, such as M1 macrophages, comprised a higher cell proportion in ECM/SVF-gels than in Coleman fat. It is known that pro-inflammatory M1 macrophages can be polarized by Th1 cytokines, such as IFN-γ or tissue necrosis factor (TNF)-α [Citation23,Citation24]. Inflammatory response and adipogenesis gene sets were the top 2 gene sets enriched in Coleman fat (both NESs were −1.67, FDR < 0.05) (), and this might be because immune cells might have undergone greater loss than the other SVF cells in ECM/SVF-gels, and Coleman fat contains massive amounts of mature adipocytes.
Figure 5. Gene set enrichment analysis (GSEA) based on whole gene expression profiles. (A and B) Gene sets involved in responses to interferon (IFN)-α and IFN-γ were enriched in the ECM/SVF-gel (FDR <0.05). (C and D) Gene sets involved in inflammatory response and adipogenesis were the top 2 gene sets enriched in Coleman fat (FDR <0.05). NES, normalized enrichment score.
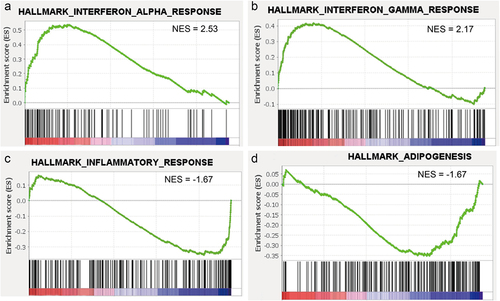
2.2. Comparison of SVF cell content between Coleman fat and ECM/SVF-gels
The CIBERSORT algorithm can accurately characterize immune cell composition. We used the CIBERSORT algorithm to evaluate the relative proportions of 22 immune cell subsets in Coleman fat and ECM/SVF-gels (), and the proportions of these cell types were summed to 1. Among 22 immune cell types, only naïve CD4+ T cells were not detected in any samples. Compared with Coleman fat, ECM/SVF-gels contained a lower proportion (FDR <0.05) of M2 macrophages, resting mast cells, and monocytes, and a higher proportion (FDR <0.05) of M1 macrophages, but the proportions of the other immune cells were not statistically different between the two groups. Despite the decrease of M2 macrophage and resting mast cell proportions in ECM/SVF-gels, M2 macrophages (28.8% vs. 23.2%), resting CD4+ memory T cells (17.9% vs. 21.5%), M1 macrophages (7.3% vs. 9.8%), resting mast cells (13.8% vs. 9.4%), and M0 macrophages (6.6% vs. 8.8%) represented the top five most abundant immune cell types both in Coleman fat and ECM/SVF-gels, with M2 macrophages being the most prevalent.
Figure 6. Composition of 22 immune cells in Coleman fat and the ECM/SVF-gel estimated by the CIBERSORT algorithm (only naïve CD4+ T cells were not detected in any of the samples). A. Relative proportion of 22 immune cells in each sample; B. Overall distribution of 22 immune cells in Coleman fat and the ECM/SVF-gel, indicating that M2 macrophages (28.8% vs. 23.2%), resting CD4+ memory T cells (17.9% vs. 21.5%), M1 macrophages (7.3% vs. 9.8%), resting mast cells (13.8% vs. 9.4%), and M0 macrophages (6.6% vs. 8.8%) represented the top five infiltrating cell types both in Coleman fat and the ECM/SVF-gel. In particular, M2 macrophages were the most prevalent. Each bar plot shows the cell type and relative percentage. Different colours represent different cell types, which are shown in the right side.
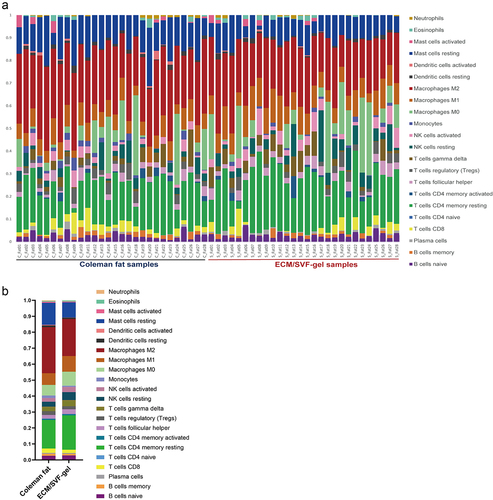
We further used the xCell algorithm to characterize the non-immune cellular composition in Coleman fat and ECM/SVF-gels. xCell scores predict relative enrichment for cell types, resembling the fractions of the cell types, but not the exact cellular proportions. The principal non-immune SVF cell types involved mesenchymal stem cells (MSCs), pericytes, preadipocytes, microvascular endothelial cells (mv endothelial cells), smooth muscle cells, and fibroblasts, none of which showed any statistical differences in xCell scores between Coleman fat and ECM/SVF-gels, despite that xCell scores of the first three cell types decreased and xCell scores of the remaining three cell types increased in ECM/SVF-gels (). Here, MSCs detected by xCell in adipose tissue actually were ADSCs.
Figure 7. The xCell-inferred enrichment scores (xCell scores) of non-immune cell types in Coleman fat (blue) and the ECM/SVF-gel (red), indicating no statistical differences in xCell scores between the two groups. The xCell scores predict relative enrichment for cell types, resembling the fractions of the cell types, but not the exact cellular proportions. The error bar denotes standard error for the sample mean. Mesenchymal stem cells detected by xCell in adipose tissue actually were ADSCs. mv Endothelial cells, microvascular Endothelial cells.
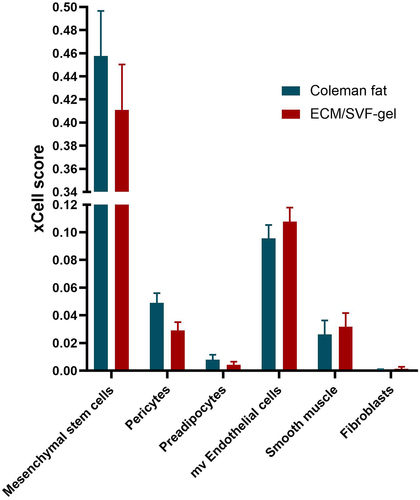
3. Discussion
As an alternative to stem cell-based therapy, ECM/SVF-gels might markedly enhance biological effects of SVF cells through maximizing enrichment of SVF cells and native adipose ECM. In the current study, we utilized WebCSEA to estimate the tissue-cell types enriched by DEGs and conducted deconvolution algorithms to characterize SVF cell subsets of Coleman fat and ECM/SVF-gels. WebCSEA and deconvolution algorithms, respectively integrating published scRNA-seq data and pure human cell type transcriptome data, are powerful bioinformatics analysis tools for unveiling the tissue cellular heterogeneity from tissue expression profiles [Citation18,Citation25,Citation26], providing more detailed cell composition information than flow cytometry, which has to depend on selection of suitable antibodies.
During the mechanical processing of ECM/SVF-gels, some cell types undergo markedly higher extents of loss, which will be reflected in prominent downregulation of cell-type gene expression in ECM/SVF-gels compared to Coleman fat. GO functional enrichment analysis result of 73 downregulated DEGs in ECM/SVF-gels implies immune cells undergo greater cellular loss than other SVF cell types, which is consistent with findings described by Yao et al., who developed ECM/SVF-gels and showed the density of ADSCs (CD45-/CD31-/CD34+) and endothelial cells (CD45-/CD31+/CD34+) increased markedly, while the CD45+ haematopoietic cell density fell in ECM/SVF-gels, compared to Coleman fat, whereas the density of other cells (CD45-/CD31-/CD34-) had no statistical differences, indicating predominant loss of CD45+ haematopoietic cells in ECM/SVF-gel formation [Citation6]. We know immune cells are CD45+ haematopoietic cells. Although the mechanical process did not concentrate CD45+ haematopoietic cells, it is still necessary to characterize the immune cell composition of ECM/SVF-gels in view of the role of immune cells in improving fat graft survival.
WebCSEA result of 73 downregulated DEGs, combined with macrophage-specific surface markers detected in downregulated DEGs, revealed that macrophages, especially M2 macrophages, were the principal immune cells lost in ECM/SVF-gel. CIBERSORT analysis indicated that M2 macrophages had a decreased cell proportion in ECM/SVF-gels, however, were still the most abundant immune cells in Coleman fat and ECM/SVF-gels. Notably, the immune cell types dominating the immune landscape of both groups are the same, including M2 macrophages, resting CD4+ memory T cells, M1 macrophages, M0 macrophages, and resting mast cells. Animal experiments have shown macrophages play a central role in both angiogenesis and adipogenesis [Citation27], with the earlier and more intensive infiltration of M2 macrophages being crucial for angiogenesis [Citation28,Citation29]. M2 macrophages can be polarized by Th2 cytokines, exerting anti-inflammatory effects and contributing to angiogenesis and tissue repair by producing anti-inflammatory cytokines and proangiogenic factors (e.g. IL-4, IL-10, VEGF, TGF-β) [Citation23,Citation24,Citation30]. As important antigen-presenting cells in adipose tissue, macrophages may initiate CD4+ T cell activation [Citation31]. Considering that differentiated CD4+ T cells have plasticity [Citation32,Citation33], there is the possibility that resting CD4+ memory T cells may be activated and polarized towards an anti-inflammatory Th2 phenotype, primarily by M2 macrophages. Mast cells can stimulate angiogenesis by releasing FGF, VEGF, and TGF-β [Citation34], and function as an alternative modulator of adipogenesis through 15-deoxy-delta-12, 14-prostaglandin J2 [Citation35]. Therefore, resting mast cells, once activated, would also promote angiogenesis and adipogenesis. We propose that the immune background of Coleman fat and ECM/SVF-gels may create a proangiogenic, proadipogenic and anti-inflammatory microenvironment. However, in ECM/SVF-gels, the effect of such an immune microenvironment might not be enhanced in view of the decreased density of CD45+ haematopoietic cells. These dominant immune cells that we identified in both groups, especially M2 macrophages, may serve as potential cell targets for optimizing the immune microenvironment of fat grafts.
In our study, xCell analysis showed no statistical differences between Coleman fat and ECM/SVF-gel in terms of the fractions of ADSCs, pericytes, preadipocytes, mv endothelial cells, smooth muscle cells, and fibroblasts. Combined with the losses of immune cells being predominant in ECM/SVF-gels, the result from xCell analysis indicates that these non-immune SVF cells, as a necessary cell source of adipose regeneration, might have no significant loss and thus be concentrated in ECM/SVF-gels. Yao et al. reported approximately 80.5% of ADSCs and 74.2% of the endothelial cells remained within the ECM/SVF-gel [Citation6]. ADSC-derived exosomes have been reported to facilitate early macrophage infiltration in fat grafts by upregulating the expression of MIP-1 and MCP-1 [Citation36], and drive M2 macrophage polarization through transactivation of arginase-1, delivery of circ-Snhg11 or circ-Rps5, activating S1P/SK1/S1PR1 signalling, or overexpressing MiR-30d-5p [Citation37–41]. ADSCs also can improve angiogenesis by upregulating the expression of HGF and bFGF, and inhibit the production of pro-inflammatory cytokines in activated macrophages [Citation36,Citation42]. We infer that the condensed nature of ECM/SVF-gels can prominently enhance proangiogenic, proadipogenic, and anti-inflammatory effects of these principal non-immune cell types, and create a better microenvironment that could efficiently promote macrophage infiltration and M2 macrophage polarization in the early stage of transplantation, as compared with an equal volume of Coleman fat. Dong et al. proposed that the long-term angiogenic effects of SVF cells may be achieved through the facilitation of M2 macrophages [Citation43]. The very first study on regeneration mode of ECM/SVF-gels showed that ECM/SVF-gels induce prompt macrophage infiltration, angiogenesis and adipogenesis in the early stage after transplantation, and notably, a large number of infiltrating M2 macrophages were observed [Citation7]. Our inference on the biological efficacies of ECM/SVF-gels still need to be verified by further functional experiments, for example, collecting conditioned culture medium from short-term culture of Coleman fat and ECM/SVF-gels to perform in vitro capillary network formation and macrophage polarization experiments.
In summary, this is the first study to determine SVF cell composition in Coleman fat and ECM/SVF-gels based on tissue transcription profiling, WebCSEA and deconvolution algorithms. The dominant immune cell types of the two groups are the same and may create a proangiogenic, proadipogenic and anti-inflammatory microenvironment. However, the loss of immune cells, especially macrophages, were predominant during ECM/SVF-gel preparation, and thus optimizing the immune microenvironment would be an effective measure to improve ECM/SVF-gel efficacy. The principal non-immune cells necessary for adipose regeneration show no significant loss in ECM/SVF-gels, and thus we infer that a condensed ECM/SVF-gel would prominently enhance biological effects of these cell types.
Our study suggests that ECM/SVF-gels are a promising alternative to stem cell-based therapy because of more favourable pro-angiogenic, anti-inflammatory and immunomodulatory mechanisms. Optimizing the immune microenvironment and promoting early M2 macrophage infiltration in fat grafts might be a critical strategy for improving fat graft retention rate.
4. Materials and methods
4.1. Patients
Lipoaspirated samples were obtained from 28 healthy women who underwent liposuction of the abdomen and/or thighs between March 2021 and June 2021 in the Medical Cosmetic Center of the First Affiliated Hospital of Shantou University Medical College (March 2021-June 2021). The women had a mean age of 32.79 ± 6.48 years, and a mean body mass index of 20.93 ± 2.29 kg/m2. This study was approved by the ethics committee of the First Affiliated Hospital of Shantou University Medical College (B-2021-256), and the written informed consent for the lipoaspirate samples to be used for research purposes was obtained from all participants.
4.2. Preparation of Coleman fat and ECM/SVF-gels
Under general anaesthesia, the patients had liposuction performed using a 3-mm multiport cannula containing several sharp side holes of 1 mm in diameter (Tulip Medical Products, San Diego, Calif.), at −0.75 Atm of suction pressure. The lipoaspirate was processed to prepare Coleman fat and ECM/SVF-gel according to the method of Yao et al. previously described [Citation6]. Briefly, lipoaspirate collected in a syringe was allowed to stand for 10 min on ice, then the lower liquid portion was discarded to obtain the upper sedimented fat layer. The sedimented fat was centrifuged at 1200 g for 3 min to remove the upper oil layer and lower liquid layer, and only the middle fat layer (Coleman fat) was retained. The Coleman fat was then mechanically emulsified to destroy mature adipocytes by shifting, at a rate of 10 ml/sec for 1 min, between two 10-ml syringes connected by a female-to-female Luer-Lok connector (specially designed for transfer) with an internal diameter of 2.4 mm. The emulsified fat was centrifuged at 2000 g for 3 min to remove the upper oil layer and lower liquid layer, and the middle fat layer was collected and defined as the ECM/SVF-gel ().
4.3. Library construction and bulk RNA-seq
RNA from Coleman fat and ECM/SVF-gel was isolated using TRIzol (Thermo Fisher Scientific, USA). RNA integrity was assessed using an Agilent 2100 bioanalyzer (Agilent Technologies, USA). RNA sequencing libraries were prepared using an NEBNext Ultra RNA Library Prep Kit for Illumina (NEB, USA), according to the manufacturer’s instructions, with 3 μg total RNA per sample used as the input. Qualities of sequencing libraries were assessed with an Agilent 2100 bioanalyzer (Agilent Technologies, USA). Libraries were sequenced on an Illumina NovaSeq 6000 platform at the Novogene Bioinformatics Institute (Guangzhou). Finally, 150-bp paired-end reads were generated.
4.4. Raw data processing and differential gene expression identification
Raw data (raw reads) in FASTQ format were processed through in-house Perl scripts to remove reads containing adapters, poly-N, and low-quality reads, and obtain clean data (clean reads). Clean reads were mapped to the human reference genome (hg19) by HISAT2 (v2.0.5). FeatureCounts (v1.5.0-p3) was used to count the read numbers mapped to each gene. Gene expression was estimated by using fragments per kilobase transcript sequence per million base pairs sequenced (FPKM) values.
DEGs were identified using the DESeq2 R package (1.16.1). False-discovery rate (FDR)-adjusted p-values were calculated using the Benjamini-Hochberg method. Genes with an FDR value of < 0.05 and fold-change in expression > 2 were considered differentially expressed. DEGs were visualized by volcano plot using the ggplot2 package (version 3.4.2).
4.5. Biological functional enrichment analysis and cell-type-specific enrichment analysis
DEGs were subjected to GO functional enrichment analysis for biological processes using the Database for Annotation, Visualization and Integrated Discovery (DAVID v 6.7, https://david.ncifcrf.gov/home.jsp) and Metascape (http://metascape.org). An FDR < 0.05 was set as the cut-off value. The enriched biological processes (FDR <0.05) were visualized as a bar plot using GraphPad 8.0. GSEA based on whole gene expression profiles was performed using GSEA software [Citation44]. The number of permutations was set to 1000, the permutation type was set as a ‘gene set’, and gene set databases were set as Hallmark gene sets, which were downloaded from the Molecular Signatures Database (MSigDB, http://software.broadinstitute.org/gsea/msigdb/index.jsp). The WebCSEA (https://bioinfo.uth.edu/webcsea/) platform, established on 111 scRNA-seq panels of human tissues and 1,355 tissue-cell types from 61 different general tissues across 11 human organ systems, was used to estimate the tissue- and cell-type-specificity of the DEGs [Citation18].
4.6. Assessment of cell composition of Coleman fat and ECM/SVF-gels
CIBERSORT (http://cibersortx.stanford.edu) and xCell (http://xCell.ucsf.edu/) online tools were carried out to identify cell types and quantify cell relative proportions from gene expression profiles obtained by bulk RNA-seq of Coleman fat and ECM/SVF-gels [Citation25,Citation26]. CIBERSORT, a deconvolution algorithm based on linear support vector regression, can distinguish 22 human haematopoietic cell phenotypes. xCell, a gene signature-based method integrating the advantages of gene set enrichment with deconvolution approaches, was used to infer 64 immune and stromal cell types.
Comparison of relative proportions of cell types between Coleman fat and ECM/SVF-gels was performed using multiple t tests, and differences with an FDR < 0.05 were considered statistically significant.
Author contributions
Yingchang Ji and Guohong Zhang designed the research. Yingchang Ji, Mengmeng Wang, and Zhehui Chen performed liposuction and preparation of Coleman and ECM/SVF-gels. Changhao Lu and Guangping Zhang collected Coleman and ECM/SVF-gel samples and did RNA extraction. Xiaoyun Li and Guohong Zhang performed the data analysis. Xiaoyun Li wrote the manuscript. Yingchang Ji and Guohong Zhang revised the manuscript critically for important intellectual content. All authors are in agreement with the content of the manuscript and approved the submitted version.
Supplemental Material
Download Zip (15.3 MB)Disclosure statement
No potential conflict of interest was reported by the author(s).
Data availability statement
The detailed information of our raw data link: The raw sequence data reported in this manuscript have been deposited in the Genome Sequence Archive (Genomics, Proteomics & Bioinformatics 2021) in National Genomics Data Center (Nucleic Acids Res 2022), China National Center for Bioinformation/Beijing Institute of Genomics, Chinese Academy of Sciences (GSA-Human: HRA007009) that are publicly accessible at https://ngdc.cncb.ac.cn/gsa-human.
Supplementary material
Supplemental data for this article can be accessed online at https://doi.org/10.1080/21623945.2024.2360037
Additional information
Funding
References
- Coleman SR. Structural fat grafting. Aesthet Surg J. 1998;18(5):386–12. doi: 10.1016/S1090-820X(98)70098-6 Epub 1998/09/01. PubMed PMID: 19328166.
- Coleman SR. Structural fat grafting: more than a permanent filler. Plast Reconstr Surg. 2006;118(3 Suppl):108S–120S. doi: 10.1097/01.prs.0000234610.81672.e7 Epub 2006/08/29. PubMed PMID: 16936550.
- Egro FM, Roy E, Rubin JP, et al. Evolution of the Coleman Technique. Plast Reconstr Surg. 2022;150(2):329e–336e. doi: 10.1097/PRS.0000000000009355 Epub 2022/06/07. PubMed PMID: 35666154.
- Suga H, Eto H, Aoi N, et al. Adipose tissue remodeling under ischemia: death of adipocytes and activation of stem/progenitor cells. Plast Reconstr Surg. 2010;126(6):1911–1923. doi: 10.1097/PRS.0b013e3181f4468b Epub 2010/12/03. PubMed PMID: 21124131.
- Mineda K, Kuno S, Kato H, et al. Chronic inflammation and progressive calcification as a result of fat necrosis: the worst outcome in fat grafting. Plast Reconstr Surg. 2014;133(5):1064–1072. doi: 10.1097/PRS.0000000000000097 Epub 2014/04/30. PubMed PMID: 24776542.
- Yao Y, Dong Z, Liao Y, Zhang P, Ma J, Gao J, et al. Adipose extracellular matrix/stromal vascular fraction gel: a novel adipose tissue-derived injectable for stem cell therapy. Plast Reconstr Surg. 2017;139(4):867–879. doi: 10.1097/PRS.0000000000003214 Epub 2016/12/22. PubMed PMID: 28002250.
- Zhang Y, Cai J, Zhou T, et al. Improved long-term volume retention of stromal vascular fraction gel grafting with enhanced angiogenesis and adipogenesis. Plast Reconstr Surg. 2018;141(5):676e–686e. doi: 10.1097/PRS.0000000000004312 Epub 2018/01/16. PubMed PMID: 29334574.
- Yao Y, Cai J, Zhang P, et al. Adipose stromal vascular fraction gel grafting: a new method for tissue volumization and rejuvenation. Dermatol Surg. 2018;44(10):1278–1286. doi: 10.1097/DSS.0000000000001556 Epub 2018/05/22. PubMed PMID: 29781904.
- Jiang S, Quan Y, Wang J, Cai J, Lu F. Fat grafting for facial rejuvenation using stromal vascular fraction gel injection. Clin Plast Surg. 2020;47(1):73–79. doi: 10.1016/j.cps.2019.09.001 Epub 2019/11/20. PubMed PMID: 31739900.
- Cai J, Wang J, Hu W, et al. Mechanical micronization of lipoaspirates for the treatment of horizontal neck lines. Plast Reconstr Surg. 2020;145(2):345–353. doi: 10.1097/PRS.0000000000006456 Epub 2020/01/28. PubMed PMID: 31985619.
- Zhu Y, Yang F, Yang Y, et al. A retrospective study of SVF-gel compared with nanofat combined with high-density fat in the treatment of early periorbital aging. Ophthalmic Plast Reconstr Surg. 2022;38(4):340–347. doi: 10.1097/IOP.0000000000002103 Epub 2021/12/11. PubMed PMID: 34889312.
- Guo J, Nguyen A, Banyard DA, et al. Stromal vascular fraction: A regenerative reality? Part 2: Mechanisms of regenerative action. J Plast Reconstr Aesthet Surg. 2016;69(2):180–188. doi: 10.1016/j.bjps.2015.10.014 Epub 2015/11/08. PubMed PMID: 26546112.
- Ramakrishnan VM, Boyd NL. The adipose stromal vascular fraction as a complex cellular source for tissue engineering applications. Tissue Eng Part B Rev. 2018;24(4):289–299. doi: 10.1089/ten.TEB.2017.0061 Epub 2017/03/21. PubMed PMID: 28316259.
- Fu S, Luan J, Xin M, et al. Fate of adipose-derived stromal vascular fraction cells after co-implantation with fat grafts: evidence of cell survival and differentiation in ischemic adipose tissue. Plast Reconstr Surg. 2013;132(2):363–373. doi: 10.1097/PRS.0b013e31829588b3 Epub 2013/07/31. PubMed PMID: 23897335.
- Zhu M, Dong Z, Gao J, Liao Y, Xue J, Yuan Y, et al. Adipocyte regeneration after free fat transplantation: promotion by stromal vascular fraction cells. Cell Transplant. 2015;24(1):49–62. doi: 10.3727/096368913X675133 Epub 2013/11/01. PubMed PMID: 24172865.
- Zhu M, Xue J, Lu S, et al. Anti-inflammatory effect of stromal vascular fraction cells in fat transplantation. Exp Ther Med. 2019;17(2):1435–1439. doi: 10.3892/etm.2018.7082 Epub 2019/01/27. PubMed PMID: 30680025.
- Ye Y, Zou J, Tan M, et al. Phenotypic and cellular characteristics of a stromal vascular fraction/extracellular matrix gel prepared using mechanical shear force on human fat. Front Bioeng Biotechnol. 2021;9:638415. doi: 10.3389/fbioe.2021.638415 Epub 2021/03/16. PubMed PMID: 33718340.
- Dai Y, Hu R, Liu A, Cho KS, Manuel AM, Li X, et al. WebCSEA: web-based cell-type-specific enrichment analysis of genes. Nucleic Acids Res. 2022;50(W1):W782–W90. doi: 10.1093/nar/gkac392 Epub 2022/05/25. PubMed PMID: 35610053.
- Law SK, Micklem KJ, Shaw JM, et al. A new macrophage differentiation antigen which is a member of the scavenger receptor superfamily. Eur J Immunol. 1993;23(9):2320–2325. doi: 10.1002/eji.1830230940 Epub 1993/09/01. PubMed PMID: 8370408.
- Stein M, Keshav S, Harris N, et al. Interleukin 4 potently enhances murine macrophage mannose receptor activity: a marker of alternative immunologic macrophage activation. J Exp Med. 1992;176(1):287–292. doi: 10.1084/jem.176.1.287 Epub 1992/07/01. PubMed PMID: 1613462.
- Gundra UM, Girgis NM, Ruckerl D, et al. Alternatively activated macrophages derived from monocytes and tissue macrophages are phenotypically and functionally distinct. Blood. 2014;123(20):e110–22. doi: 10.1182/blood-2013-08-520619 Epub 2014/04/04. PubMed PMID: 24695852.
- Mantovani A, Sica A, Sozzani S, et al. The chemokine system in diverse forms of macrophage activation and polarization. Trends Immunol. 2004;25(12):677–686. doi: 10.1016/j.it.2004.09.015 Epub 2004/11/09. PubMed PMID: 15530839.
- Russo L, Lumeng CN. Properties and functions of adipose tissue macrophages in obesity. Immunology. 2018;155(4):407–417. doi: 10.1111/imm.13002 Epub 2018/09/20. PubMed PMID: 30229891.
- Shapouri-Moghaddam A, Mohammadian S, Vazini H, et al. Macrophage plasticity, polarization, and function in health and disease. J Cell Physiol. 2018;233(9):6425–6440. doi: 10.1002/jcp.26429 Epub 2018/01/11. PubMed PMID: 29319160.
- Newman AM, Liu CL, Green MR, et al. Robust enumeration of cell subsets from tissue expression profiles. Nat Methods. 2015;12(5):453–457. doi: 10.1038/nmeth.3337 Epub 2015/03/31. PubMed PMID: 25822800.
- Aran D, Hu Z, Butte AJ. xCell: digitally portraying the tissue cellular heterogeneity landscape. Genome Biol. 2017;18(1):220. doi: 10.1186/s13059-017-1349-1 Epub 2017/11/17. PubMed PMID: 29141660.
- Debels H, Galea L, Han XL, et al. Macrophages play a key role in angiogenesis and adipogenesis in a mouse tissue engineering model. Tissue Eng Part A. 2013;19(23–24):2615–2625. doi: 10.1089/ten.TEA.2013.0071 Epub 2013/07/13. PubMed PMID: 23844978.
- Cai J, Feng J, Liu K, et al. Early macrophage infiltration improves fat graft survival by inducing angiogenesis and hematopoietic stem cell recruitment. Plast Reconstr Surg. 2018;141(2):376–386. doi: 10.1097/PRS.0000000000004028 Epub 2017/10/17. PubMed PMID: 29036027.
- Phipps KD, Gebremeskel S, Gillis J, et al. Alternatively activated M2 macrophages improve autologous Fat Graft survival in a mouse model through induction of angiogenesis. Plast Reconstr Surg. 2015;135(1):140–149. doi: 10.1097/PRS.0000000000000793 Epub 2014/12/30. PubMed PMID: 25539302.
- Kadomoto S, Izumi K, Mizokami A. Macrophage polarity and disease control. Int J Mol Sci. 2021;23(1). doi: 10.3390/ijms23010144 Epub 2022/01/12. PubMed PMID: 35008577.
- Cho KW, Morris DL, DelProposto JL, et al. An MHC II-dependent activation loop between adipose tissue macrophages and CD4+ T cells controls obesity-induced inflammation. Cell Rep. 2014;9(2):605–617. doi: 10.1016/j.celrep.2014.09.004 Epub 2014/10/15. PubMed PMID: 25310975.
- Yamane H, Paul WE. Memory CD4+ T cells: fate determination, positive feedback and plasticity. Cell Mol Life Sci. 2012;69(10):1577–1583. doi: 10.1007/s00018-012-0966-9 Epub 2012/04/07. PubMed PMID: 22481436.
- Saravia J, Chapman NM, Chi H. Helper T cell differentiation. Cell Mol Immunol. 2019;16(7):634–643. doi: 10.1038/s41423-019-0220-6 Epub 2019/03/15. PubMed PMID: 30867582.
- Karimi A, Shahrooz R, Hobbenagh R, Delirezh N, Amani S, Garssen J, et al. Histological evidence for therapeutic induction of angiogenesis using mast cells and platelet-rich plasma within a bioengineered scaffold following Rat Hindlimb Ischemia. Cell J. 2020;21(4):391–400. doi: 10.22074/cellj.2020.6287 Epub 2019/08/04. PubMed PMID: 31376320.
- Tanaka A, Nomura Y, Matsuda A, et al. Mast cells function as an alternative modulator of adipogenesis through 15-deoxy-delta-12, 14-prostaglandin J2. Am J Physiol Cell Physiol. 2011;301(6):C1360–7. doi: 10.1152/ajpcell.00514.2010 Epub 2011/08/26. PubMed PMID: 21865589.
- Chen B, Cai J, Wei Y, et al. Exosomes are comparable to source adipose stem cells in fat graft retention with up-regulating early inflammation and angiogenesis. Plast Reconstr Surg. 2019;144(5):816e–827e. doi: 10.1097/PRS.0000000000006175 Epub 2019/08/07. PubMed PMID: 31385891.
- Zhao H, Shang Q, Pan Z, et al. Exosomes from adipose-derived stem cells attenuate adipose inflammation and obesity through polarizing M2 macrophages and beiging in white adipose tissue. Diabetes. 2018;67(2):235–247. doi: 10.2337/db17-0356 Epub 2017/11/15. PubMed PMID: 29133512.
- Shi R, Jin Y, Zhao S, et al. Hypoxic ADSC-derived exosomes enhance wound healing in diabetic mice via delivery of circ-Snhg11 and induction of M2-like macrophage polarization. Biomed Pharmacother. 2022;153:113463. doi: 10.1016/j.biopha.2022.113463 Epub 2022/09/10. PubMed PMID: 36076572.
- Yang H, Tu Z, Yang D, et al. Exosomes from hypoxic pre-treated ADSCs attenuate acute ischemic stroke-induced brain injury via delivery of circ-Rps5 and promote M2 microglia/macrophage polarization. Neurosci Lett. 2022;769:136389. doi: 10.1016/j.neulet.2021.136389 Epub 2021/12/14. PubMed PMID: 34896256.
- Deng S, Zhou X, Ge Z, et al. Exosomes from adipose-derived mesenchymal stem cells ameliorate cardiac damage after myocardial infarction by activating S1P/SK1/S1PR1 signaling and promoting macrophage M2 polarization. Int J Biochem Cell Biol. 2019;114:105564. doi: 10.1016/j.biocel.2019.105564 Epub 2019/07/06. PubMed PMID: 31276786.
- Jiang M, Wang H, Jin M, et al. Exosomes from MiR-30d-5p-ADSCs reverse acute ischemic stroke-induced, autophagy-mediated brain injury by promoting M2 microglial/macrophage polarization. Cell Physiol Biochem. 2018;47(2):864–878. doi: 10.1159/000490078 Epub 2018/05/29. PubMed PMID: 29807362.
- Kotani T, Masutani R, Suzuka T, et al. Anti-inflammatory and anti-fibrotic effects of intravenous adipose-derived stem cell transplantation in a mouse model of bleomycin-induced interstitial pneumonia. Sci Rep. 2017;7(1):14608. doi: 10.1038/s41598-017-15022-3 Epub 2017/11/04. PubMed PMID: 29097816.
- Dong Z, Fu R, Liu L, Lu F. Stromal vascular fraction (SVF) cells enhance long-term survival of autologous fat grafting through the facilitation of M2 macrophages. Cell Biol Int. 2013;37(8):855–859. doi: 10.1002/cbin.10099 Epub 2013/03/26. PubMed PMID: 23526646.
- Subramanian A, Tamayo P, Mootha VK, et al. Gene set enrichment analysis: a knowledge-based approach for interpreting genome-wide expression profiles. Proc Natl Acad Sci U S A. 2005;102(43):15545–15550. doi: 10.1073/pnas.0506580102 Epub 2005/10/04. PubMed PMID: 16199517.