ABSTRACT
Middle East respiratory coronavirus (MERS-CoV) is a newly emergent, highly pathogenic coronavirus that is associated with 34% mortality rate. MERS-CoV remains listed as priority pathogen by the WHO. Since its discovery in 2012 and despite the efforts to develop coronaviruses vaccines to fight against SARS-CoV-2, there are currently no MERS-CoV vaccine that has been approved. Therefore, there is high demand to continue on the development of prophylactic vaccines against MERS-CoV. Current advancements in vaccine developments can be adapted for the development of improved MERS-CoV vaccines candidates. Nucleic acid-based vaccines, including pDNA and mRNA, are relatively new class of vaccine platforms. In this work, we developed pDNA and mRNA vaccine candidates expressing S.FL gene of MERS-CoV. Further, we synthesized a silane functionalized hierarchical aluminosilicate to encapsulate each vaccine candidates. We tested the nucleic acid vaccine candidates in mice and evaluated humoral antibodies response. Interestingly, we determined that the non-encapsulated, codon optimized S.FL pDNA vaccine candidate elicited the highest level of antibody responses against S.FL and S1 of MERS-CoV. Encapsulation of mRNA with nanoporous aluminosilicate increased the humoral antibody responses, whereas encapsulation of pDNA did not. These findings suggests that MERS-CoV S.FL pDNA vaccine candidate induced the highest level of humoral responses. This study will enhance further optimization of nanosilica as potential carrier for mRNA vaccines. In conclusion, this study suggests MERS-CoV pDNA vaccine candidate as a suitable vaccine platform for further pivotal preclinical testings.
Introduction
Middle east respiratory coronavirus (MERS-CoV) is a respiratory viral disease that belongs to Orthocoronavirinae subfamily of Coronaviridae.Citation1,Citation2 The Orthocoronaviridae are positive sense, single stranded RNA that consists of 4 genera: alpha-CoV, beta-CoV, gamma-CoV, and delta-CoV.Citation3 Severe acute respiratory syndrome coronavirus-1 (SARS-CoV-1), SARS-CoV-2, and MERS-CoV all belongs to the beta-CoV genus.Citation3,Citation4 However, infection with MERS-CoV is associated with significantly higher mortality rate compared to SARS-CoV-2.Citation2 The MERS-CoV genome is about 30 kilobase (Kb) that consists of 11 open reading frames (ORF).Citation1 S gene in particular is a class I transmembrane glycoprotein. This protein can be cleaved into two non-covalently bond units: N-terminal globular S1 subunit and a membrane proximal S2 subunit.Citation5 The S1 contains the receptor binding domain (RBD) responsible for virus attachment to the host cell receptor, while the S2 contains the fusion domain, thereby mediates the virus entry.Citation5 Unlike with SARS-CoV and SARS-CoV-2 where they use angiotensin converting enzyme 2 (ACE-2) for virus cellular entry, MERS-CoV is dependent on dipeptidyl peptidase 4 (DPP-4 or CD26) for its entry into host cell.Citation6,Citation7 The S protein is the primary antigenic determinants in coronaviruses that is responsible for eliciting antibody mediated responses against the virus. Specifically, humoral antibodies responses have been shown to protect infected animals and humans.Citation8,Citation9
The MERS-CoV case was first reported on June 2012 and has been associated with ongoing sporadic outbreaks since then.Citation10,Citation11 Since then, MERS-CoV infected more than 2500 people globally, including more than 800 deaths. Due to its high fatality rate (~40%), MERS-CoV is categorized as one of the high priority pathogens identified by the WHO.Citation12 Currently, no human vaccines against MERS-CoV have ever been approved. The S protein is the primary target for immune response generated toward MERS-CoV. Therefore, efforts made to develop vaccines candidates against MERS-CoV were mainly focused on S protein.Citation13–15 Several MERS-CoV vaccine candidates had been explored preclinically and clinically including live attenuated, viral vector, and pDNA.Citation16–23 The nucleic acid-based vaccine including pDNA and mRNA vaccines are promising next generation vaccines platforms.Citation24 While conventional vaccines are typically manufactured as bulk production of vaccine in mammalian cells or egg embryos, nucleic acid-based vaccines relays on the host cell machinery to produce the encoded protein antigen. pDNA vaccine, in particular, offers number of advantages compared to conventional vaccines, including easy of production, cost effectiveness, and high stability.Citation25 Contrastingly, mRNA is inherently unstable, and therefore requires encapsulation to physically protect mRNA from degradation by RNase. Furthermore, the delivery of mRNA into host cell requires efficient intracellular delivery.Citation25,Citation26
Several nanoparticles involving encapsulating mRNA have been tested and reported as promising technology with low genotoxicity and high biocompatibility. As such, lipid-based nanoparticle has reached clinical trial application and subsequent approval for mRNA based COVID-19 vaccines.Citation27 In addition, graphene quantum dots (GQD) functionalized with polyethyleneimine polymer has been used in RNA based therapeutics. Specifically, the cationic charge density of polymer shown to improve the RNA complexation and effective delivery to liver cells.Citation28 Further, dendric mesoporous/ZIF-8 nanocomposite has been used in mRNA delivery. The presence of mesopores, tetrasulfide bond on the pore walls assisting nanocrystals of ZIF-8 was reported to influence high mRNA loading and transfection efficacy.Citation29
While the majority of research had focused on the application of lipid nanocarriers for mRNA delivery, less attentions has been paid toward inorganic aluminosilicate nanomaterials. Due to its versatile feature, nanoporous mesoporous silica is a multifunctional platform that has been developed and tested in a wide range of vaccine and drug delivery, including pDNA and mRNA vaccines and therapeutics. Of note, mesoporous silica with tunable pore diameters (2–50 nm) and large surface texture has reported an ideal candidate for gene delivery (~1000 m2/g).Citation30 The efficacy of mesoporous silica capped with template quaternary trimethyl ammonium as functionalized linker along with imidazolo-oxindole PKR inhibitor C16 had been used for the delivery of mRNA.Citation31 The structured silica with functional abilities and uniform particle size is most suited for siRNA mediated gene silencing.Citation32 Silylation using amino functional moieties such as 3-Aminopropyltriethoxysilane (APTES), aminopropyl trimethoxysilane (APTMS) and carbodiimide routes are important strategies to loading RNA and subsequent release of genes into various applications.Citation33
As a proof of principle, we have developed and tested mRNA and pDNA vaccine candidates against MERS-CoV using the S gene. Moreover, we have designed a 3-aminopropyltrimethoxysilane (APTMOS) functionalized hierarchical aluminosilicate (HAS) designated as APTMOS-HAS as nanocarrier for mRNA and pDNA vaccine candidates. The mRNA vaccine candidate was the first to be developed against MERS-CoV and the APTMOS-HAS were explored for first time for nucleic acid vaccine encapsulation. As such, we performed parallel immunological evaluation of non-encapsulated pDNA and mRNA vaccine candidates vs pDNA and mRNA vaccines candidates loaded APTMOS functionalized spherical silica.
Methods
Vaccine synthesis
pDNA synthesis
The S gene sequence for MERS-CoV was retrieved from PubMed (ANF29162.1). The pDNA vaccine was constructed bioinformatically using codon optimization approach.
Negative cis-acting sites (splice sites, TATA boxes, etc.) that can negatively affect the gene expression were eliminated wherever possible. GC contents were adjusted to prolong the half-life of mRNA. Codon optimizations were adapted to Homo Sapiens. NheI and BamHI restriction sites were added upstream and downstream of the synthesized sequence respectively. Kozac sequence and a stop codon were added upstream and downstream of the optimized S gene sequence, respectively. In total, a sequences total of 4059 nucleotides was synthesized.
The vaccine was de novo synthesized (GenArt, Germany). The S.opt.FL gene insert was cloned into pVAX1. The nucleotide sequence was confirmed by gene sequencing. The construct was transformed into 101 HB competent cells and incubated in LB media with Kanamycin at 37°C for an overnight. For pDNA production, successfully cloned pDNA vaccine construct was grown in LB media containing kanamycin and incubated for an overnight at 37°C. A ChargeSwitch-Pro filter plasmid Maxiprep purification kit (Cat#CS31106, Thermo Fisher Scientific, USA) was used to purify the S.opt.FL pDNA.
mRNA synthesis
For mRNA vaccine, the mRNA was in-vitro synthesized using mMessege mMachine T7 kit (Cat#AM1344, Thermo Fisher Scientific, Lithuania). Briefly, a linearized DNA from the S.opt.FL pDNA containing T7 promoter was prepared. The transcribed and capped mRNA was synthesized according to manufacturer’s protocol. Polyadenylation was carried out using PolyA tailing kit (Cat# AM1350, Thermo Fisher Scientific, Lithuania) according to the manufacture’s recommendations. and polyA tailing (300 nucleotides). mRNA purification was carried out using MEGAclear transcription clean up kit (Cat#AM1908, Thermo Fisher Scientific, Lithuania) was used to remove the remaining DNase was added to remove the nucleotides, short oligo nucleotides, proteins, and salts from RNA. The quality and integrity of mRNA was assessed by using 1% agarose TAE gel electrophoresis and was visualized under (UV) using 1 ug/mL ethidium bromide.
Synthesis of silane functionalized hierarchical aluminosilicate
Monodispersed spherical silica (silica) was used (Superior silica, USA). Hierarchical Aluminosilicate (HAS) (SiO2/Al2O3 ratio 80) with micro and meso hexagonal pores was synthesized through top-down approach using nanozeolitic seed and mesoporous template CTAB.Citation34 The parent ZSM-5 (zeolyst) was dissolved in alkaline solution in presence of template and then pH adjusted using dilute sulfuric acid. The material was left for hydrothermal aging for few days and then collected through filtration, washing, drying and calcination steps. The obtained nanomaterial was further modified by refluxing using 3-aminopropyltrimethoxysilane by dispersing in toluene solution. After silane addition, the solution mixture was refluxed for 6 hours under argon atmosphere. Then solution was centrifuged, precipitated sample was washed and dried. The sample was denoted as APTMOS-HAS. For the other sample including silica, a similar refluxing procedure was carried out and denoted as APTMOS-silica.
Characterization
The functionalized nanosupport were extensively characterized using various physico-chemical techniques. The phase of hierarchical mesoporous material was analyzed using X-ray diffraction (XRD). The textural characters (such as surface area, and porosity) was measured by nitrogen adsorption technique (at 77K using an ASAP-2010 porosimeter, Micromeritics Corporation, GA). The morphology of functionalized aluminosilicate was evaluated through electron microscopy technique (HRTEM). The silanol surface of silica will be observed using Mid-infrared spectra on a Nicolet (Avatar 360) and UV-Vis spectrophotometer instrument. Thermogravimetric-differential thermal analysis (TG-DTA) was used to study the amination content. The morphology of HAS and APTMOS-HAS samples were analyzed using transmission electron microscopy (JEM2100F from JEOL Ltd, Tokyo, Japan).
pDNA and mRNA encapsulation
A molecular weight of 1.5 mg with APTMOS-HAS were mixed with 100 ul of TE-buffer (pH 8.1) and using pipette it is homogenized. Then DNA or mRNA of 100 ug were added to each tube and vortexed at 22°C for 24 hours. An evaluation of encapsulation efficiency was determined by calculating the level of pDNA and mRNA in the supernatant. The encapsulation efficiency was calculated as follow: Amount of pDNA or mRNA present in aluminosilicate/Initial amount of pDNA or mRNA taken x100. To evaluate encapsulation stability, we conducted heat inactivation at 95°C for an hour and endonucleases inactivation at 37°C for 20 minutes followed by enzyme deactivation at 70°C for 30 minutes.
Ethics statement
Animal protocols were approved by the Institutional Review Board (IRB-2018-252-IRMC) at Imam Abdulrahman Bin Faisal University (IAU), and experiments were done in compliance with the institution guidelines.
Animal immunizations
6–8-week-old male B57BL/6J were kindly provided by King Faisal Specialist Hospital and Research Center. The mice were randomly assigned to their respective group and were housed and treated at the animal house facility at Imam Abdulrahman Bi Faisal University. Mice were assigned six per group. A total of five groups received either of the following (pDNA, mRNA, pDNA+nanosilica, mRNA+Nanosilica, control). Mice were immunized with 100 ug pDNA in a total volume of 200 of PBS delivered intramuscularly into tibialis interior muscle with needle. Mice were immunized at days 0 and 14. Blood was collected for each mouse prior to first immunization and 2 weeks after each immunization. Sera from all mice groups were collected at weeks 0, 2, 4, and 6 for immunogenicity testing and mice were subsequently sacrificed.
ELISA
96-well plates (Cat#442404Thermos Fisher, Denmark) were coated with 1 ug/ml of the full-length S antigen (Cat#40069-V08B, Sinobiologic, China), S1 antigen (Cat#40069-V08H, Sinobiologic, China) of MERS-CoV or full-length S antigen (Cat#40069-V08B, Sinobiologic, China) or S antigen (Cat#442404, Thermos Fisher, Denmark) of SARS-CoV-2 and incubated for 1 hour at room temperature. Plates were washed five times with 300 ul of 1XPBS. 200 ul of blocking reagent of 5% nonfat dry milk (Cat#170–6404, Bio-Rad, USA) in tris buffer were added to each well and incubated for 1 hour at room temperature. After 5 washes, 100 ul of serially diluted serum from the vaccinated mice was added to each well and incubated for 1 hour at room temperature. After five washes with 300 ul of 1XPBS, 100 ul if rabbit anti-mouse IgG secondary antibody conjugated with horseradish peroxidase (HRP) (Cat#31430, Invitrogen, USA) was added to each well and incubated for 1 hour at room temperature. Plates were washed five times with 300 of 1XPBS and 3,3,’5,5’ TMB substrate (Cat#34021, Thermo Fisher Scientific) was added to each well according to the manufacturer’s instructions. 100 ul of 2 M of sulfuric acid (2 M H2SO4) was added to all wells to stop the reactions. Lastly, absorbance is determined at optical density (OD) value at 450 nm by microplate reader (DIAsource). End-point titer was calculated as reciprocal to the highest sera dilution when OD value is 2 folds higher than pre-bleed serum.
Results
No previous study had explored mRNA vaccine as potential candidate against MERS-CoV. While surface glycoprotein for MERS-CoV is immunogenic, in-vitro synthesis of mRNA encoding S can be challenging. The sequence length of S gene is 4059 bp. Therefore, optimization of gene sequence is necessary prior to pDNA and mRNA synthesis. To develop MERS-CoV nucleic acid vaccine candidates, the S gene was de-novo synthesized. S gene sequence (2016 strain) was codon optimized for mammalian codon preference (Homo sapiens) to enhance gene expression upon immunization. During synthesis of codon-optimized S gene, the following steps were avoided: internal TATA boxes, chi-sites and ribosomal entry sites, and AT rich or CG rich sequences stretches. Furthermore, to avoid premature termination of codon optimized DNA during mRNA synthesis, the following were avoided: RNA instability motifs repeat and RNA secondary structure, cryptic splice donor and acceptor sites in higher eukaryotes, GC contents are adjusted to prolong mRNA half-life, and PolyA adenylation is added after mRNA synthesis of long S transcript (). The optimized S gene sequence was then chemically synthesized (~4059 nucleotides) and cloned into pVax1 vector at BamhI and NheI flanking sites. The successfully cloned pDNA encoding S gene is purified and correct bands sizes are determined by BamHI and NheI ().
Figure 1. Codon quality of MERS-CoV S gene by obtained GeneOptimizer. (a,b) Histograms showing the percentage of sequence codons that fall within selected optimization parameters. The quality value of the most frequently used codons for given amino acids in the homo sapiens is set to 100. The plots show the quality of codons at the indicated codon position. (c) Average GC contents in 40 bp window centered at the indicated nucleotide position with an average GC content equal to 56%.
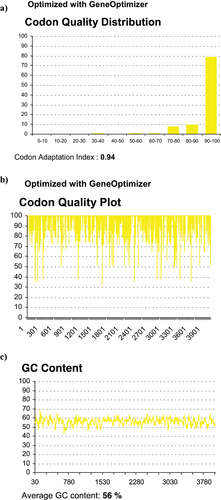
Figure 2. Agarose gel electrophoresis for the synthesized of pDNA and mRNA vaccines encoding S gene of MERS-CoV. (a). Two clones of pDNA expressing S gene. Correct band size of S gene 4000 bp and pVAX1 (3000) is showing in the gel double cut (DC) restriction analysis of BamHI and NheI. (b). In-vitro (IVT) synthesized RNA of. The correct band size of 4000 bp is showing in parallel with RNA marker.
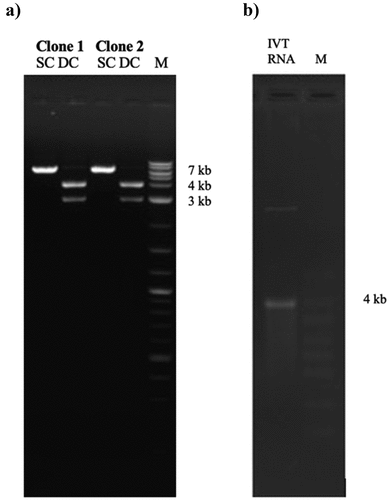
To synthesize mRNA vaccine, the circular S pDNA was linearized by BamHI. The linearized S pDNA serves as template for mRNA synthesis. As such, in-vitro synthesis using T7 polymerase starting at T7 promoter and is terminated at BamHI site was conducted. Purification and evaluation of correct mRNA synthesis was visualized by gel electrophoresis ().
The formation of mesophase and microphase of HAS and APTMOS-HAS were confirmed using X-ray diffraction analysis (). HAS exhibited a high intensity hexagonal structured peaks indicating the presence of (100), (110), and (200) planes of ordered mesoporous MCM-41 structure. For APTMOS-HAS, the mesophase peak intensity decreases (8-fold), while zeolitic phase remains constant with APTMOS functionalization indicating the silane functionalization occupies mostly on the mesophase. Thermogravimetric analysis of APTMOS functionalized on HAS showed a four steps of disintegration pattern (). An initial weight loss up to 140°C is attributed to the water removal, while the disintegration till 300°C corresponds to amino group of silane. Further decomposition up to 600°C is attributed to the chain breaking of silane chain moiety. Subsequently, the fourth step up to 800°C is assigned to the silanol group disintegration. The overall weight loss for APTMOS-HAS was of 20%, while the APTMOS corresponds to about 15%. The ZSM-5 shows the micropore filling effect with lower nitrogen uptake typical to that of MFI structure type. Microporous ZSM-5 showed a lower surface area of 400 m2/g with pore volume of 0.25 cm3/g (). For HAS, an intermediate type IV and I isotherm pattern was observed indicating the presence of hierarchical type of pore (micro/mesopores). The mesoporous surface area increases to as high as 846 m2/g with pore volume of 0.58 cm3/g. The presence of dual type of pores were obvious with pore size distribution at 2.2 and 3.8 nm (). The surface area of APTMOS functionalized HAS reduces the surface area to about 138 m2/g with pore volume of 0.28 cm3/g. The average pore size increases to about 8.2 nm. A reduction in pore volume (48%) and a subsequent increase in pore size from 3.8 nm to 8.2 nm indicates the effect of silylation formation by APTMOS at the external porous surface of hierarchical HAS ().
Figure 3. X-ray diffraction of (a) HAS and (b) APTMOS-HAS, (c and d) Thermogravimetric analysis of APTMOS-HAS, nitrogen adsorption isotherm of (E) ZSM-5, (f) HAS, and (g) APTMOS-HAS and pore size distribution of (h) ZSM-5, (i) HAS, and (j) APTMOS-HAS.
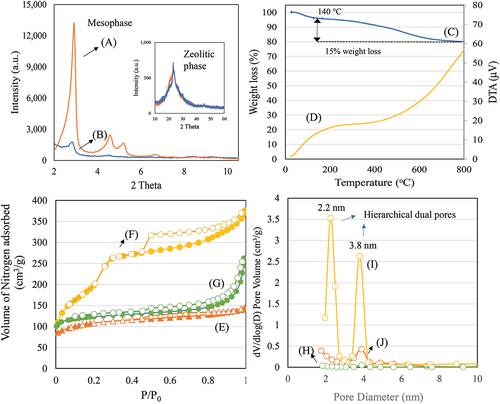
shows the morphology of HAS and APTMOS-HAS. For HAS, the presence of hierarchical dual pores of micropore and mesopore channels can be clearly seen indicating the characteristics of zeolitic micropore nanoclusters and hexagonal MCM-41. For APTMOS-HAS, after silane functionalization, a thick formation occurs on the pores of HAS reflecting a distinct change on the external surface of hierarchical HAS (). These changes of pore ordering correlate with the x-ray diffraction and surface area measurement () further confirming a textural modification with APTMOS. García-González and colleaguesCitation35 observed that such a surface textural decrease with increased pore diameter was mainly due to the enlarged particle diameter attributed to deposition of hydrophobic large silane molecules as aggregates on TiO2 nanoparticles. However, in our case, the presence of large surface area of about 138 m2/g () even after silylation shows that HAS can dispersed in TE buffer for pDNA and mRNA encapsulation.
Figure 4. (a–d) Transmission electron microscope images depicting morphology of (a) HAS and (b–d) APTMOS-HAS.
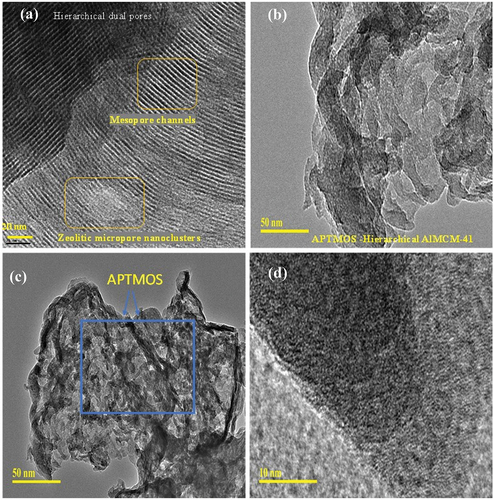
Table 1. Textural characteristics of ZSM-5, HAS and APTMOS-HAS.
For vaccine delivery, two types of silane functionalized nanoparticles such as APTMOS-HAS and APTMOS-silica were synthesized. Following encapsulation efficiency, APTMOS-HAS was determined as the best nanoparticle candidate for mRNA and pDNA, with encapsulation efficiency of 55% and 47%, respectively (). Further, it was determined that APTMOS-HAS protected the nucleic acid from degradation following heat inactivation at 96 as wells after treatment with endonuclease ().
Figure 5. 0.8% agarose gel analysis for the MERS-CoV vaccine candidates. Samples in the left lane were subjected to heat inactivation at 95°C for 1 hour and samples are shown as follow: pDNA (Untreated), pDNA (Heat Inactivated), pDNA+HAS (Heat Inactivated). Samples in the right lane were subjected to TURBO DNase digestion and samples are shown as follow: pDNA (Untreated), pDNA (Turbo DNase), pDNA+HAS (TURBO DNase).
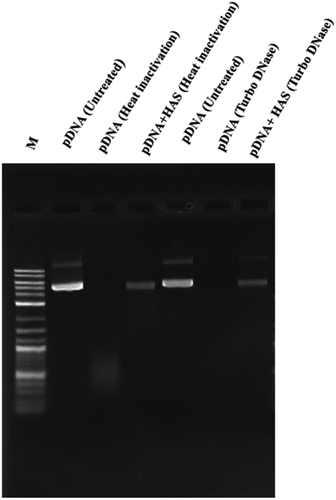
Table 2. Encapsulation efficiency for the NA MERS-CoV vaccine candidates.
To examine the immune responses of pDNA and mRNA induced by either MERS-CoV S (pDNA, pDNA-HAS, mRNA, or mRNA-HAS), we vaccinated 6–8-week-old male B57BL/6J mice twice (day 0 and day 14; IM) (). The humoral antibody responses in mice group were analyzed at weeks 0, 2, 4, and 6. The sera’s reactivity in immunized mice groups were measured against MERS-CoV full length Spike protein (S1+S2) and against S1 domain. pDNA vaccine elicited highest antibody mediated response in all mice group, whereas mRNA elicited significantly lower antibody response following each immunization ().
Figure 6. Humoral immune response in vaccinated mice groups against MERS-CoV S.FL. Binding antibody responses was measured by indirect ELISA measured at week 0 (a), week 2 (b), week 4 (c), week 6 (d). Statistical analysis was performed using one way ANOVA.
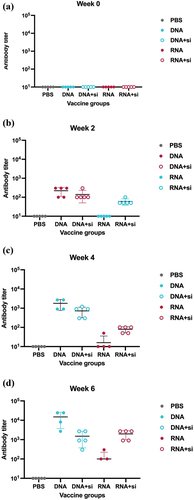
Table 3. Immunization schedule for the MERS-CoV vaccine candidates.
The highest antibody responses were detected at week 6, four weeks after receiving the second dose (). To examine the immunogenicity after HAS encapsulation, the mRNA vaccine had slightly higher immunogenicity compared to non-encapsulated mRNA (). Contrastingly, encapsulation of pDNA with HAS did not enhance the immunogenicity (). Of note, the antibody titer elicited against full length spike (S.FL) were higher than the antibody titer generated against S1 in all vaccine groups (). This verifies the importance of vaccinating the complete domain of MERS-CoV spike.
Discussion
Currently, the priority pathogens listed by WHO include MERS-CoV, SARS-CoV-2, Ebola virus, Marburg virus, Nipah virus, Lassa virus, Rift Valley fever, Zika, and Crimean-Congo hemorrhagic fever. Therefore, continuous efforts on the development of MERS-CoV vaccine candidates remains a global priority.
Previous work on MERS-CoV had focused vaccine platforms such as viral vector, subunit, pDNA platforms. While several mRNA vaccines had been developed against SARS-CoV-2 and influenzas virus, no prior mRNA vaccines were developed against MERS-CoV, except with synthesis of mRNA.Citation36 RNA is an intrinsically unstable molecule, making it a challenging platform. Various techniques have been explored to enhance mRNA stability, including: 5’ capping, 3’ polyA tail length, and encapsulation. As such, we opted to conduct further genetic modifications to increase mRNA stability. Likewise, further development of novel nanoparticles holds great potential for retaining mRNA stability and for efficient intracellular delivery of mRNA vaccines and therapeutics. The selection of nanoparticle can greatly enhance the efficiency of intracellular delivery and protection form nucleases. Mesoporous silica nanoparticles are widely used in pharmaceutical formulations that have been proven to be safe in wide array of pharmaceutical applications. As such, nano-silica has excellent biocompatibility and are chemically stable. Like lipid-based nanoparticles, nano-silica has been shown to play a role in antibody binding and cell interaction.Citation37
Here, we have developed and tested mRNA vaccine against candidates encapsulated with novel hierarchical aluminosilicate tailored specially for the delivery of mRNA with large molecular size. We have investigated hierarchical aluminosilicate and spherical silica with different topology, size, and pore size. Hierarchical aluminosilicate was shown to be a better candidate for pDNA and mRNA encapsulation. These optimizations allowed us to select nanoparticle candidate for pDNA and mRNA encapsulation. We observed slight improvement of immunogenicity after mRNA encapsulation. Humoral immune response was improved after encapsulating mRNA. The low titer antibody was observed in mRNA vaccine groups, suggesting further improvement and optimizations of nanoparticle pore size/design. Therefore, we suggest conducting further improvement in nanoparticle pore size design, to enhance the vaccine immunogenicity tailored for the mRNA size while protecting it from nucleases.
Contrastingly, pDNA vaccine technology is very stable and is highly flexible platform with capability to induce both humoral and cellular immune response without the need of further encapsulation step. This is also achieved through a robust, simple, and scalable GMP production. In our work, the pDNA vaccine elicited highest antibody response compared to mRNA alone or encapsulated mRNA. We did not observe statistically significant immunogenicity enhancement following further pDNA encapsulation. Therefore, further immunogenicity characterizations to evaluate IgG subsets, neutralizing antibody response, and durability of immune response will be critical for determining vaccine efficacy. In addition, conducting evaluating of cellular immune response will be valuable for the assessment of T cell immunity generated by pDNA vaccine platform. In addition, subsequent pivotal preclinical studies including protective efficacy by challenging animals models with live virus inoculum will be conducted in future studies to assesses the efficacy of pDNA vaccine candidates to block MERS-CoV transmission. Over decades of research, it has been well established that pDNA vaccine remains at the injection site and does not persists or biodistribute following IM, or subcutaneous (SC) injections.Citation38–43 Therefore, biodistribution studies are waived for pDNA vaccine based on the WHO guidelines.
Consistent with previous studies, pDNA vaccine developed against S.FL protein of SARS-CoV-1, SARS-CoV-2, and MERS-CoV elicited high immune responses.Citation19,Citation44,Citation45 Further, it will be worthwhile to explore the breadth of MERS-CoV pDNA vaccine candidates against antigenically divergent MERS-CoV strains as well as other human coronaviruses. As such, virus bioinformatic aided resources can be valuable in predicting and screening MERS-CoV strains to significantly reduce laboratory testing for identifying antigenically divergent strains.Citation46–48 In summary, pDNA vaccine candidate alone elicited the highest immune response following two doses without statically significant enhancement of immunogenicity after encapsulation. Therefore, we suggest to further conduct of pivotal preclinical studies using non-encapsulated MERS-CoV pDNA vaccine candidates.
Author contributions
I.A Fund acquisition, I.A & R.J Conceptualization, investigation, formal analysis, writing and review original manuscript, I.A supervision.
Acknowledgments
We thank animal Dr. Abdullah Assiri (King Faisal Specialist Hospital and Research Center, Riyadh, Saudi Arabia) for kindly providing C57BL/6 mice. We also thank Mr.Hussain Alhawaj and Mr. Taleb Alkhamis at the Animal house facility, Imam Abdulrahman Bin Faisal University for their assistance in animal bleeding and vaccine administration. We thank Ms. Nabela Calamata Macadato at Nucleic Acid Vaccine Lab for her assistance in lab work.
Disclosure statement
No potential conflict of interest was reported by the authors.
Additional information
Funding
References
- Woo PC, Lau SK, Huang Y, Yuen KY. Coronavirus diversity, phylogeny and interspecies jumping. Exp Biol Med. 2009;234(10):1117–10. doi:10.3181/0903-MR-94.
- Fehr AR, Perlman S. Coronaviruses: an overview of their replication and pathogenesis. Coronaviruses. 2015:1282;1–23.
- Woo PC, Lau SK, Lam CS, Lau CC, Tsang AK, Lau JH, Bai KY, Teng JLL, Tsang CCC, Wang M, et al. Discovery of seven novel mammalian and avian coronaviruses in the genus deltacoronavirus supports bat coronaviruses as the gene source of alphacoronavirus and betacoronavirus and avian coronaviruses as the gene source of gammacoronavirus and deltacoronavirus. J Virol. 2012;86(7):3995–4008. doi:10.1128/JVI.06540-11.
- Woo PC, Huang Y, Lau SK, Yuen KY. Coronavirus genomics and bioinformatics analysis. viruses. 2010;2(8):1804–20. doi:10.3390/v2081803.
- Wang Q, Wong G, Lu G, Yan J, Gao GF. MERS-CoV spike protein: targets for vaccines and therapeutics. Antiviral Res. 2016;133:165–77. doi:10.1016/j.antiviral.2016.07.015.
- Raj VS, Mou H, Smits SL, Dekkers DH, Müller MA, Dijkman R, Muth D, Demmers JAA, Zaki A, Fouchier RAM, et al. Dipeptidyl peptidase 4 is a functional receptor for the emerging human coronavirus-EMC. Nature. 2013;495(7440):251–4. doi:10.1038/nature12005.
- Kindler E, Jónsdóttir HR, Muth D, Hamming OJ, Hartmann R, Rodriguez R, Geffers R, Fouchier RAM, Drosten C, Müller MA. Efficient replication of the novel human betacoronavirus EMC on primary human epithelium highlights its zoonotic potential. MBio. 2013;4(1):10–1128. doi:10.1128/mBio.00611-12.
- İnandıklıoğlu N, Akkoc T. Immune responses to SARS-CoV, MERS-CoV and SARS-CoV-2. In: Turksen K, editors. Cell biology and translational medicine, volume 9: stem cell-based therapeutic approaches in disease. Springer; 2020. p. 5–12.
- Zhou Y, Jiang S, Du L. Prospects for a MERS-CoV spike vaccine. Expert Rev Vaccines. 2018;17(8):677–86. doi:10.1080/14760584.2018.1506702.
- Ramadan N, Shaib H. Middle East respiratory syndrome coronavirus (MERS-CoV): a review. Germs. 2019;9(1):35. doi:10.18683/germs.2019.1155.
- Mertz GJ, Wormser GP. Zoonoses: infectious diseases transmissible from animals to humans, fourth edition. Clin Infect Dis. 2016;63(1):148.2–9. doi:10.1093/cid/ciw234.
- World Health Organization. Middle east respiratory syndrome coronavirus (MERS-CoV)–update. Disease Outbreak News–MERS-CoV from, 05-05; 2014.
- Al-Amri SS, Abbas AT, Siddiq LA, Alghamdi A, Sanki MA, Al-Muhanna MK, Alhabbab RY, Azhar EI, Li X, Hashem AM. Immunogenicity of candidate MERS-CoV DNA vaccines based on the spike protein. Sci Rep. 2017;7(1):44875. doi:10.1038/srep44875.
- Du L, Yang Y, Zhou Y, Lu L, Li F, Jiang S. MERS-CoV spike protein: a key target for antivirals. Expert Opin Ther Targets. 2017;21(2):131–43. doi:10.1080/14728222.2017.1271415.
- Ma C, Li Y, Wang L, Zhao G, Tao X, Tseng CTK, Zhou Y, Du L, Jiang S. Intranasal vaccination with recombinant receptor-binding domain of MERS-CoV spike protein induces much stronger local mucosal immune responses than subcutaneous immunization: implication for designing novel mucosal MERS vaccines. Vaccine. 2014;32(18):2100–8. doi:10.1016/j.vaccine.2014.02.004.
- Gutiérrez-Álvarez J, Honrubia JM, Fernández-Delgado R, Wang L, Castaño-Rodríguez C, Zúñiga S, Sola I, Enjuanes L. Genetically engineered live-attenuated Middle East respiratory syndrome coronavirus viruses confer full protection against lethal infection. MBio. 2021;12(2):10–1128. doi:10.1128/mBio.00103-21.
- Choi JA, Goo J, Yang E, Jung DI, Lee S, Rho S, Jeong Y, Park Y-S, Park H, Moon Y-H. Cross-protection against MERS-CoV by prime-boost vaccination using viral spike DNA and protein. J Virol. 2020;94(24):10–1128. doi:10.1128/JVI.01176-20.
- Cho H, Jang Y, Park KH, Choi H, Nowakowska A, Lee HJ, Kim M, Kang M-H, Kim J-H, Shin HY. Human endogenous retrovirus-enveloped baculoviral DNA vaccines against MERS-CoV and SARS-CoV2. NPJ Vaccines. 2021;6(1):37. doi:10.1038/s41541-021-00303-w.
- Wang L, Shi W, Joyce MG, Modjarrad K, Zhang Y, Leung K, Lees CR, Zhou T, Yassine HM, Kanekiyo M. Evaluation of candidate vaccine approaches for MERS-CoV. Nat Commun. 2015;6(1):7712. doi:10.1038/ncomms8712.
- Alharbi NK, Padron-Regalado E, Thompson CP, Kupke A, Wells D, Sloan MA, Grehan K, Temperton N, Lambe T, Warimwe G. ChAdOx1 and MVA based vaccine candidates against MERS-CoV elicit neutralising antibodies and cellular immune responses in mice. Vaccine. 2017;35(30):3780–8. doi:10.1016/j.vaccine.2017.05.032.
- Muthumani K, Falzarano D, Reuschel EL, Kraynyak K, Ugen K, Kim P, Maslow J, Kim JJ, Sardesai NY, Kobinger G. A synthetic consensus anti-spike protein DNA vaccine induces protective immunity against Middle East respiratory syndrome coronavirus in non-human primates. Int J Infect Dis. 2016;45:23. doi:10.1016/j.ijid.2016.02.083.
- Folegatti PM, Bittaye M, Flaxman A, Lopez FR, Bellamy D, Kupke A, Mair C, Makinson R, Sheridan J, Rohde C. Safety and immunogenicity of a candidate Middle East respiratory syndrome coronavirus viral-vectored vaccine: a dose-escalation, open-label, non-randomised, uncontrolled, phase 1 trial. Lancet Infect Dis. 2020;20(7):816–26. doi:10.1016/S1473-3099(20)30160-2.
- Koch T, Dahlke C, Fathi A, Kupke A, Krähling V, Okba NM, Halwe S, Rohde C, Eickmann M, Volz A. Safety and immunogenicity of a modified vaccinia virus Ankara vector vaccine candidate for Middle East respiratory syndrome: an open-label, phase 1 trial. Lancet Infect Dis. 2020;20(7):827–38. doi:10.1016/S1473-3099(20)30248-6.
- Gary EN, Weiner DB. DNA vaccines: prime time is now. Curr Opin Immunol. 2020;65:21–7. doi:10.1016/j.coi.2020.01.006.
- Liu MA. A comparison of plasmid DNA and mRNA as vaccine technologies. Nato Adv Sci Inst Se. 2019;7(2):37. doi:10.3390/vaccines7020037.
- Ho W, Gao M, Li F, Li Z, Zhang XQ, Xu X. Next‐generation vaccines: nanoparticle‐mediated dna and mrna delivery. Adv Healthcare Mater. 2021;10(8):2001812. doi:10.1002/adhm.202001812.
- Anselmo AC, Mitragotri S. Nanoparticles in the clinic: an update post COVID‐19 vaccines. Bioeng Transl Med. 2021 Sep;6(3):e10246. doi:10.1002/btm2.10246.
- Liu Y, Zhao C, Sabirsh A, Ye L, Wu X, Lu H, Liu J. A novel graphene quantum dot-based mRNA delivery platform. ChemistryOpen. 2021 Apr 7;10(7):666–71. doi:10.1002/open.202000200.
- Wang Y, Song H, Liu C, Zhang Y, Kong Y, Tang J, Yang Y, Yu C. Confined growth of ZIF-8 in dendritic mesoporous organosilica nanoparticles as bioregulators for enhanced mRNA delivery in vivo. Natl Sci Rev. 2021 Aug;8(8):268. doi:10.1093/nsr/nwaa268.
- Janjua TI, Cao Y, Yu C, Popat A. Clinical translation of silica nanoparticles. Nat Rev Mater. 2021 Dec;6(12):1072–4. doi:10.1038/s41578-021-00385-x.
- Zhang W, Liu Y, Chin JM, Phua KK. Sustained release of PKR inhibitor C16 from mesoporous silica nanoparticles significantly enhances mRNA translation and anti-tumor vaccination. Eur J Pharm Biopharm. 2021 Jun 1;163:179–87. doi:10.1016/j.ejpb.2021.03.011.
- Hom C, Lu J, Liong M, Luo H, Li Z, Zink JI, Tamanoi F. Mesoporous silica nanoparticles facilitate delivery of siRNA to shutdown signaling pathways in mammalian cells. Small. 2010 June 6;6(11):1185–90. doi:10.1002/smll.200901966.
- Zhou Y, Quan G, Wu Q, Zhang X, Niu B, Wu B, Huang Y, Pan X, Wu C. Mesoporous silica nanoparticles for drug and gene delivery. Acta Pharm Sin B. 2018 Mar 1;8(2):165–77. doi:10.1016/j.apsb.2018.01.007.
- Ravinayagam V, Jermy BR. Fabricating hierarchical ZSM-5 to induct long chain antioxidant coenzyme Q10 for biomedical application. J Saudi Chem Soc. 2019;23(4):461–76. doi:10.1016/j.jscs.2018.09.001.
- García-González CA, Fraile J, López-Periago A, Domingo C. Preparation of silane-coated TiO2 nanoparticles in supercritical CO2. J Colloid Interface Sci. 2009;338(2):491–9. doi:10.1016/j.jcis.2009.06.035.
- Tai W, Zhang X, Yang Y, Zhu J, Du L. Advances in mRNA and other vaccines against MERS-CoV. Transl Res. 2022;242:20–37. doi:10.1016/j.trsl.2021.11.007.
- Li Y, Li P, Li R, Xu Q. Intracellular antibody delivery mediated by lipids, polymers, and inorganic nanomaterials for therapeutic applications. Adv Ther. 2020;3(12):2000178. doi:10.1002/adtp.202000178.
- Ledwith BJ, Manam S, Troilo PJ, Barnum AB, Pauley CJ, Griffiths TG 2nd, Nichols WW, Beare CM, Bagdon WJ, Nichols WW. Plasmid DNA vaccines: assay for integration into host genomic DNA. Dev Biol (Basel). 2000;104:33–43. doi:10.1159/000053993.
- Ledwith BJ, Manam S, Troilo PJ, Barnum AB, Pauley CJ, Griffiths TG II, Nichols WW, Beare CM, Bagdon WJ, Nichols WW. Plasmid DNA vaccines: investigation of integration into host cellular DNA following intramuscular injection in mice. Intervirology. 2001;43(4–6):258–72. doi:10.1159/000053993.
- Kim BM, Lee DS, Choi JH, Kim CY, Son M, Sun YS, Baek KH, Park KS, Sung YC, Kim WB. In vivo kinetics and biodistribution of a HIV-1 DNA vaccine after administration in mice. Arch Pharm Res. 2003;26(6):493–8. doi:10.1007/BF02976869.
- Pilling AM, Harman RM, Jones SA, Mccormack NA, Lavender D, Haworth R. The assessment of local tolerance, acute toxicity, and DNA biodistribution following particle-mediated delivery of a DNA vaccine to minipigs. Toxicol Pathol. 2002;30(3):298–305. doi:10.1080/01926230252929864.
- Bureau MF, Naimi S, Ibad RT, Seguin J, Georger C, Arnould E, Scherman D, Blanche F, Delaere P, Scherman D. Intramuscular plasmid DNA electrotransfer: biodistribution and degradation. Biochim Biophys Acta Gene Struct Expression. 2004;1676(2):138–48. doi:10.1016/j.bbaexp.2003.11.005.
- Sheets RL, Stein J, Manetz TS, Duffy C, Nason M, Andrews C, Kong W-P, Nabel GJ, Gomez PL. Biodistribution of DNA plasmid vaccines against HIV-1, Ebola, severe acute respiratory syndrome, or West Nile virus is similar, without integration, despite differing plasmid backbones or gene inserts. Toxicol Sci. 2006;91(2):610–9. doi:10.1093/toxsci/kfj169.
- Almansour I, Macadato NC, Alshammari T. Immunogenicity of multiple doses of pDNA vaccines against SARS-CoV-2. Pharmaceuticals. 2021;14(1):39. doi:10.3390/ph14010039.
- Roper RL, Rehm KE. SARS vaccines: where are we? Expert Rev Vaccines. 2009;8(7):887–98. doi:10.1586/erv.09.43.
- Almansour I, Boudellioua I. hCoronavirusesDB: an integrated bioinformatics resource for human coronaviruses. Database. 2022;2022:baac017. doi:10.1093/database/baac017.
- Almansour I, Alhagri M. MMRdb: measles, mumps, and rubella viruses database and analysis resource. Infect Genet Evol. 2019;75:103982. doi:10.1016/j.meegid.2019.103982.
- Almansour I, Alhagri M, Alfares R, Alshehri M, Bakhashwain R, Maarouf A. IRAM: virus capsid database and analysis resource. Database. 2019;2019:baz079. doi:10.1093/database/baz079.