ABSTRACT
Non-small cell lung cancer (NSCLC) is one of the most malignant tumors. The treatment of advanced NSCLC can be challenging due to drug resistance. The discovery of novel cancer-testis antigens to develop new strategies for advanced metastatic NSCLC is required. AKAP4 is an oncogene discovered in some malignant tumors, and its molecular function of AKAP4 in NSCLC is unknown. This study aimed to explore the potential function of AKAP4 in the development and progression of NSCLC. AKAP-4 was found to be significantly upregulated in both clinical NSCLC tissues and NSCLC cell lines. Cell viability and migration were suppressed, apoptosis was induced, and tube formation was inhibited by the knockdown of AKAP-4, accompanied by the downregulation of VEGF, N-cadherin, EphA2, and MMP-2, and upregulation of c-AMP, PKA, and E-cadherin. In vivo xenograft experiments revealed that tumor growth was inhibited by the knockdown of AKAP4, accompanied by the activation of c-AMP/PKA signaling and inhibition of epithelial-mesenchymal transition progression. Our results show that AKAP4 might be an important target for treating NSCLC because of its function in promoting the migration and proliferation of NSCLC cells.
Introduction
Lung cancer is a malignant tumor with the highest morbidity and mortality worldwide, among which non-small cell lung cancer (NSCLC) accounts for approximately 80%-85% of cases. Local diffusion or distant metastasis is commonly observed in patients diagnosed with NSCLC [Citation1]. Due to drug resistance, the treatment of advanced NSCLC using targeted therapies, such as inhibitors or antagonists of epidermal growth factor receptor (EGFR) and anaplastic lymphoma kinase, can be challenging [Citation2]. In addition, it has been reported that EGFR is positive in a relatively small percentage of the white population and that there are population studies showing variations between Asia, Europe, and America [Citation3,Citation4]. Recently, immunotherapy for the treatment of NSCLC has received great attention, including classic immunotherapy and immune checkpoint inhibitors. Tumor vaccines are regarded as the classic immunotherapy. The current tumor vaccines available include whole-cell vaccines (such as dendritic cell vaccines), tumor peptide vaccines (such as L-BLP25), and genetic engineering vaccines (such as Belagenpumatucel-L) [Citation5,Citation6]. Recently, immune checkpoint inhibitors have been widely applied for the treatment of advanced NSCLC and breast cancer, including CTLA-4 and PD-1 inhibitors [Citation7,Citation8]. Although promising clinical progression has been achieved by these immunotherapies, significant adverse effects and drug resistance still arise, which will limit their application [Citation9]. Therefore, there is an urgent need to develop novel heterogeneous immune antigens for the treatment of advanced NSCLC.
Cancer-testis antigens (CTAs) are a group of heterogeneous antigens that are highly expressed in tumor tissues, testicular tissues, and placental tissues and are rarely expressed in other normal tissues [Citation10]. A high correlation between the expression of CTAs and the progression and metastasis of malignant tumors has been widely reported [Citation11]. As human leukocyte antigens are rarely expressed in testicular tissues and placental tissues, mild autoimmune reactions are induced by CTAs, which makes CTAs potentially superior immune targets in the field of immunotherapy [Citation12]. A-kinase anchor protein 4(AKAP4) is a sperm fibrous sheath protein and is reported to be an important regulator of sperm flagellum function [Citation13]. AKAP4 is a type of CTA with low expression in normal tissues, except for testicular and placental tissues. In addition, AKAP4 is a novel CTA that is abnormally expressed in multiple types of malignant tumors, including ovarian cancer [Citation14], cervical cancer [Citation15], multiple myeloma [Citation16], breast cancer [Citation17], and prostate cancer [Citation18]. Gumireddy et al. identified AKAP4 as a potential biomarker for NSCLC by analyzing CTA messenger ribonucleic acids (mRNAs) in peripheral blood monocytes isolated from 116 patients with NSCLC, which was further verified in 264 patients and 135 healthy volunteers. In addition, the expression level of AKAP4 increased significantly with the development of neoplasm staging. In subsequent follow-up investigations, AKAP4 was found to be greatly downregulated in surgical patients and upregulated in relapse patients [Citation19]. However, the molecular function of AKAP4 in the development and progression of NSCLC remains unclear.
In the present study, we suspected that AKAP4 facilitated the metastasis and growth of NSCLC cells by regulating epithelial-mesenchymal transition (EMT) progression and the c-AMP/PKA pathway. The present study aimed to explore the potential therapeutic targets for the treatment of advanced NSCLC by investigating the regulatory mechanism of AKAP4 on the growth and migration of NSCLC.
Materials and methods
Collection of clinical tissues and cell treatments
Six patients diagnosed with advanced NSCLC underwent surgery to remove tumor tissues, which were connected with para-carcinoma tissues. Tumor tissues and para-carcinoma tissues were identified and separated by an experienced oncologist who performed the surgeries. The surgeries were conducted in Taizhou Hospital of Zhejiang Province Hospital. The collection of tissues was approved by the ethics committee of Taizhou Hospital of Zhejiang Province Hospital. The NSCLC cell lines, including A549, NCI-H1299, and H460 cells, and the normal human lung epithelial cell line, BEAS-2B, were obtained from ATCC (Maryland, USA) and cultured in Dulbecco’s modified eagle medium (DMEM) supplemented with 10% fetal bovine serum at 5% CO2 and 37°C. Human umbilical vein endothelial cells (HUVECs) were purchased from ATCC (Manassas, VA, USA) and cultured in endothelial cell medium at 5% CO2 and 37°C.
Real-time polymerase chain reaction (RT-PCR)
TRIzol© reagent (Invitrogen. California, USA) was used to extract total RNA from the cells in each group, which were further reverse-transcribed to cDNA using the TaqMan miRNA reverse transcription kit (Invitrogen. California, USA). RT-PCR was conducted using SYBR® Green Real‐time PCR Master Mix (Roche Diagnostics, Basel, Switzerland), with the following conditions: 94°C for 5 min, 30 cycles of 94°C for 30 s, and 58–61°C for 30 s, followed by 72°C for 2 min. Glyceraldehyde 3-phosphate dehydrogenase (GAPDH) was used to normalize the relative expression of target genes, which was calculated using the 2−ΔΔCt method [Citation20].
Western blot assay
Following isolation of the total proteins from the treated cells, a BCA kit (Merck, New Jersey, USA) was used to determine the concentration of proteins, and about 40 μg of protein was loaded, followed by separation with 12% SDS-PAGE. Then, the proteins in the SDS-PAGE were transferred to a polyvinylidene difluoride membrane (Merck, New Jersey, USA). The membrane was subsequently incubated with 5% BSA for 2 h, followed by incubation with primary antibodies against E-cadherin (1:800, Abcam, Cambridge, UK), N-cadherin (1:800, Abcam, Cambridge, UK), PKA (1:800, Abcam, Cambridge, UK), vascular endothelial growth factor (VEGF; 1:800, Abcam, Cambridge, UK), EphA2 (1:800, Abcam, Cambridge, UK), MMP-2 (1:800, Abcam, Cambridge, UK), and GAPDH (1:800, Abcam, Cambridge, UK) overnight. After incubation in a solution of secondary antibody (1:2000, Abcam, Cambridge, UK) for 1.5 h, the membrane was exposed to ECL solution (Invitrogen, California, USA), and the relative expression level of target proteins was confirmed by visualization with Image J software [Citation21].
Immunohistochemical analysis
Briefly, tissues were fixed with 4% paraformaldehyde, dehydrated, and embedded in paraffin. Subsequently, tissues were cut into 5 μm sections, which were deparaffinized and rehydrated. After incubation in 5% BSA for half an hour, the sections were incubated with primary antibodies against c-AMP (1:200, Abcam, Cambridge, UK), PKA (1:200, Abcam, Cambridge, UK), AKAP4 (1:200, Abcam, Cambridge, UK), or VEGF (1:200, Abcam, Cambridge, UK), followed by incubation with horseradish peroxidase (HRP)-conjugated secondary antibody. Finally, images were taken using a light microscope (Laird, Missouri, USA). Five high-power fields were selected and imaged randomly on each slide, and the average optical density was calculated using ImageJ software [Citation22].
Transfection
Two independent siRNAs (siRNA 1# and siRNA 2#) were designed to knockdown the expression level of AKAP4. In brief, H460 cells or HUVECs were seeded on 6-well plates at a density of 150,000 cells/well, followed by replacement of the complete culture medium with serum-free and antibiotic-free medium 1 h before transfection. Subsequently, cells were incubated with transfection mixtures containing 30 nM siRNAs using Lipofectamine 3000 (Invitrogen, Carlsbad, USA) according to the manufacturer’s instructions for 5 h, followed by replacement of the medium with the full culture medium. After 24–72 h incubation, cells at approximately 90% confluence were harvested for further analysis. SiNC was used as the negative control. The sequences of the siRNAs were as follows: siNC (5ʹ-ATCTCGCTTGGGCGAGAGTAAG-3ʹ), siRNA #1 (5ʹ-TCTATGTTCACTTGATCGG-3ʹ), and siRNA #2 (5ʹ-CAAGCGAACGGGCAATTTA-3ʹ). qRT-PCR and western blotting assays were used to evaluate the efficacy of the transfection.
Flow cytometry for the analysis of apoptosis
Cells were seeded on 6-well plates and incubated at 37°C for 48 h, followed by centrifugation at 300 × g for 5 min. The cells were collected and resuspended in serum-free medium and approximately 10 μL Annexin V reagent (eBioscience, California, USA) and 5 μL PI reagents (eBioscience, California, USA), followed by incubation for 10 min at room temperature in the dark. Then, the cell suspension was mixed with phosphate-buffered saline in the flow tube and analyzed by BD flow cytometry (BD, New York) for the analysis of apoptosis [Citation23].
Tube formation assay
After pre-cooling, the 96-well plate was coated with 50 μL of growth-factor-reduced Matrigel (BD Biosciences), followed by incubation for 30 min. HUVECs treated with different strategies were starved in serum-free medium for 24 h prior to the assay, followed by planting 2 × 104 HUVECs into each well with 200 μL medium and concentrated 75-fold using an ultrafiltration device (Millipore, USA). Tube formation of HUVECs was observed at different time points during a 12-h experimental period using a microscope. The total tube length was measured and analyzed using Image-Pro-Plus 6.0 [Citation24].
Transwell assay
Approximately 5 × 104 H460 cells were suspended in serum-free DMEM and plated into the upper insert of a 24-well transwell chamber. The cells were then incubated at 37°C for 8 h. The non-migratory cells in the upper layer were removed, and the migratory cells were fixed with 4% paraformaldehyde at room temperature for 10 min, followed by staining with crystal violet solution. Images were photographed under a light microscope (Olympus, Tokyo, Japan) and quantified by counting the number of cells in five randomly selected fields of view for each well [Citation25].
Enzyme-linked immunosorbent assay (ELISA) for the detection of c-AMP
[Citation26]. A commercial ELISA kit (R&D Systems, Minneapolis, MN, USA) was used to detect the concentration of c-AMP in H460 cells. Briefly, after centrifugation, the supernatant was collected and transferred to 96-well plates, followed by incubation in 1% BSA for the removal of non-specific binding proteins. Then, the primary antibody of c-AMP was added after washing, followed by three washes and incubation with the streptavidin- HRP-conjugated secondary antibody for 30 min. After adding the 3,3ʹ,5,5ʹ-Tetramethylbenzidine solution for 20 min, the reaction was terminated using a stop buffer. Finally, a fluorescence microscope (Leica, Wetzlar, Germany) was used to measure the absorbance at 450 nm.
Xenograft model
Female Babl/c nude mice (seven to nine weeks old) were purchased from Shanghai Slac. The xenograft models were established by subcutaneously injecting Babl/c nude mice with 5 × 105 cells/0.1 mL H460 cells, H460 cells transfected with siNC, H460 cells transfected with siRNA #1, and H460 cells transfected with siRNA 2# dissolved in 50% Matrigel® (BD Biosciences, New York, USA), respectively (n = 6 for each group). The length (L) and width (W) of each tumor were measured weekly for the calculation of tumor size according to the formula (L × W2)/2. At the end of the animal experiments, tumor tissues were collected and weighed after sacrificing the animals [Citation27].
Statistical analysis
The data obtained from the present study were expressed as the mean±SD. The data analysis was conducted using GraphPad software. The Student’s t-test was used to analyze data between two groups, and a one-way analysis of variance with the least significant difference test was used to analyze among groups. A P-value of < 0.05 was considered statistically significant.
Results
We suspected that AKAP4 functions as an oncogene in NSCLC development. The present study aimed to investigate the regulatory mechanism of AKAP4 in the growth and migration of NSCLC cells. The relative expression level of AKAP4 was assessed in both NSCLC tissues and cell lines. Subsequently, we established an AKAP4-knockdown H460 cell line. We further explored the proliferation and migration ability of AKAP4-knockdown H460 cells, as well as the angiogenesis of AKAP4-knockdown HUVECs. In addition, the activity of the cAMP-PKA pathway and EMT progression in AKAP4-knockdown H460 cells was evaluated. Lastly, the growth of AKAP4-knockdown H460 cells in xenograft mice was investigated, along with the activity of the cAMP-PKA pathway and EMT progression in xenograft tumor tissues.
AKAP4 was highly expressed in NSCLC tissues and cell lines
To investigate the role of AKAP4 in the progression of NSCLC, we first measured the expression level of AKAP4 in NSCLC tissues and cell lines. As shown in , compared to para-carcinoma tissues, AKAP4 was significantly upregulated in clinical NSCLC tissues (**p < 0.01, vs. para-carcinoma tissues). In addition, compared to the normal human lung epithelial cell line, BEAS-2B cells, the expression level of AKAP4 was dramatically elevated in the NSCLC cell lines (), among which the highest expression level of AKAP4 was observed in H460 cells (**p < 0.01, vs. BEAS-2B cells). Therefore, in subsequent experiments, H460 cells were used as the investigational target in the present study.
Figure 1. A high expression level of AKAP4 was observed in both clinical NSCLC tissues and NSCLC cell lines. (a). RT-PCR was used to determine the gene expression level of AKAP-4 in the NSCLC tissues and para-carcinoma tissues. (b). Immunohistochemical assay was used to determine the protein expression level of AKAP-4 in the NSCLC tissues and para-carcinoma tissues (**p < 0.01 vs. para-carcinoma tissues). (c). Western blotting assay was used to determine the expression level of AKAP4 in the NSCLC cell line and HEAS-2B cells (**p < 0.01 vs. HEAS-2B cells).
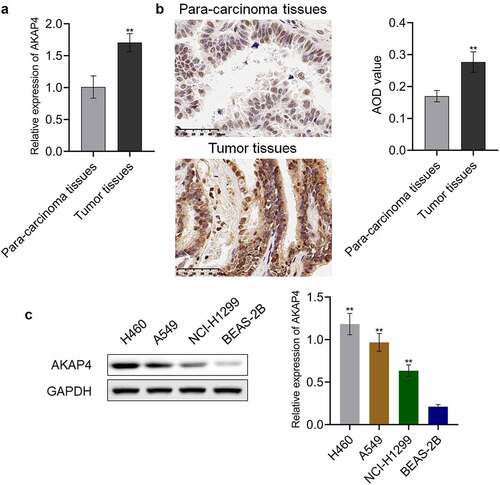
AKAP4 facilitated the proliferation of H460 cells
To explore the effects of AKAP4 in the progression of NSCLC, H460 cells were transfected with siRNA #1 or siRNA #2 to knockdown the expression level of AKAP4. As shown in , the efficacy of siRNAs was verified by RT-PCR and western blotting assays (**p < 0.01, vs. siNC). Then, the proliferation and apoptosis of transfected H460 cells were detected. As shown in , compared to siNC, cell viability was significantly suppressed by the transfection of siRNA #1 and siRNA #2 (**p < 0.01, vs. siNC). In addition, compared to the control group, the apoptotic rate () slightly changed from 7.58% to 7.71% in the siNC group, which was dramatically elevated to 17.65% and 17.60% after transfection with siRNA #1 and siRNA #2, respectively (**p < 0.01, vs. siNC). These data reveal that proliferation was inhibited, and apoptosis was induced by knocking down the expression level of AKAP4 in H460 cells.
Figure 2. Proliferation was suppressed by the knockdown of AKAP4. (a). The gene expression level of AKAP4 was detected by RT-PCR assay. (b). The protein expression level of AKAP4 was detected by western blotting assay. (c). The cell viability was measured by CCK-8 assay. (d). The apoptosis was determined by flow cytometry (**p < 0.01 vs. siNC). The control was H460 cells incubated with blank completed medium.
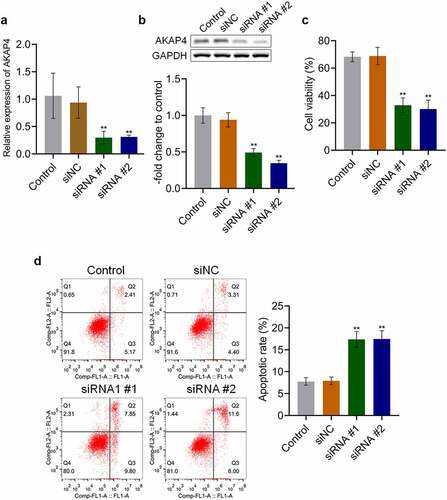
AKAP4 induced the migration of H460 cells and angiogenesis in vitro
We further explored the effects of AKAP4 on the metastasis of H460 cells. As shown in , no significant difference was observed in the number of migrated cells between the control and siNC groups, which was significantly declined in siRNA #1 and siRNA #2 transfected H460 cells (**p < 0.01, vs. siNC). The siRNAs were transfected into HUVECs to investigate the effects of AKAP4 on angiogenesis. The tube length was 32.3 mm and 30.89 mm in the control and siNC groups, respectively. Compared to siNC (), the tube length was significantly decreased to 18.5 mm and 15.09 mm in the siRNA #1 and siRNA #2 groups, respectively (**p < 0.01, vs. siNC). In addition, compared to the control group, the tube number was slightly changed from 76 to 73 by transfection with siNC, which was significantly reduced to 25 and 21 by the transfection of siRNA #1 and siRNA #2, respectively (**p < 0.01, vs. siNC). As shown in , compared to siNC, VEGF was found to be significantly downregulated in the siRNA #1 and siRNA #2 groups (**p < 0.01, vs. siNC). These results indicate that the migration of H460 cells and angiogenesis in vitro were dramatically alleviated by the knockdown of AKAP4.
Figure 3. Migration and angiogenesis were inhibited by the knockdown of AKAP4. (a). Transwell assay was used to determine the migration ability of H460 cells. (b). Tube formation assay was used to evaluate the angiogenesis of HUVECs. C. The expression level of VEGF was determined by western blotting assay (**p < 0.01 vs. siNC). The control was H460 cells incubated with blank completed medium.
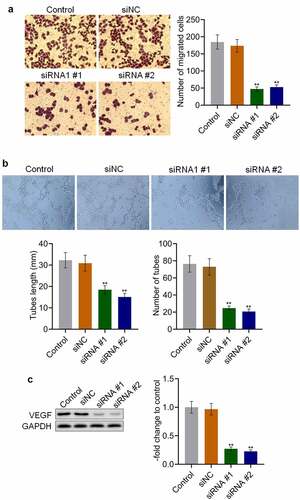
AKAP4 inhibited the c-AMP/PKA pathway and facilitated EMT progression in H460 cells
To explore the potential mechanism underlying the effects of AKAP4 on NSCLC progression, the activity of the c-AMP/PKA pathway and progression of EMT were measured. As shown in , the production of c-AMP was 9.59 U/L and 11.29 U/L in the control and siNC groups, which was dramatically promoted to 36.50 U/L and 31.66 U/L by the transfection of siRNA #1 and siRNA #2, respectively (**p < 0.01, vs. siNC). In addition, compared to siNC, the expression level of PKA () was markedly elevated in the siRNA #1 and siRNA #2 groups (**p < 0.01, vs. siNC), indicating that the c-AMP/PKA pathway was activated following the knockdown of AKAP4. Compared to siNC, the expression level of the epithelial biomarker E-cadherin was greatly elevated, and the expression levels of the mesenchymal biomarkers N-cadherin, EphA2, and MMP-2 were significantly downregulated by the transfection of siRNA #1 and siRNA #2, respectively (**p < 0.01, vs. siNC). These results reveal the effects of AKAP4 on the c-AMP/PKA pathway and EMT progression.
Figure 4. The c-AMP/PKA signaling was activated, and EMT progression was suppressed by the knockdown of AKAP4. (a). The production of c-AMP was measured by ELISA. (b). The expression level of PKA, E-cadherin, N-cadherin, EphA2, and MMP-2 was determined by western blotting assay (**p < 0.01 vs. siNC). The control was H460 cells incubated with blank completed medium.
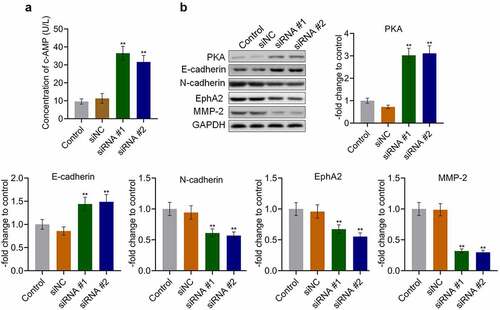
AKAP4 promoted tumor growth in H460 cells and in vivo angiogenesis
We further verified the function of AKAP4 in the H460 xenograft model. As shown in , after a six-week growth, the tumor volume and weight were significantly reduced in the siRNA #1 and siRNA #2 groups compared to the siNC group, respectively (**p < 0.01, vs. siNC), indicating a promising inhibitory effect by knocking down AKAP4. In addition, the biomarker of angiogenesis, VEGF (), was dramatically downregulated in the siRNA #1 and siRNA #2 groups (**p < 0.01, vs. siNC), indicating that the in vivo angiogenesis in H460 tumor tissues was suppressed by the knockdown of AKAP4.
Figure 5. The in vivo growth of the tumor was inhibited by the knockdown of AKAP4. (a). Tumor volumes were calculated every week after the planting. (b). Tumor weights were weighed at the end of the experiments. (c). The images of the tumor tissues in each group. (d). The expression level of VEGF was determined by western blotting assay. (e). The expression level of VEGF was determined by immunohistochemical assay (**p < 0.01 vs. siNC). The controls were nude mice planted with H460 cells incubated with blank completed medium.
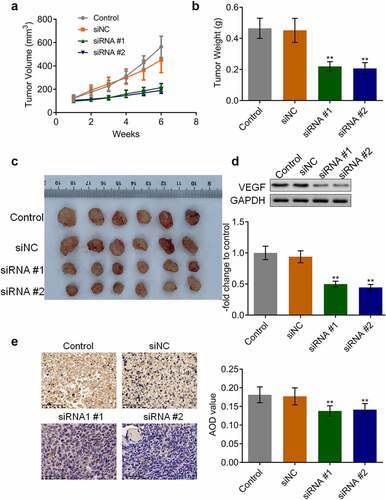
AKAP4 inhibited the c-AMP/PKA pathway and facilitated EMT progression in H460 xenograft tissues
The state of the c-AMP/PKA pathway and EMT progression in the tumor tissues were subsequently investigated. As shown in , compared to siNC, the expression level of AKAP4 was significantly decreased, and the expression levels of c-AMP and PKA were dramatically elevated in the siRNA #1 and siRNA #2 groups, respectively (**p < 0.01, vs. siNC), indicating an activation effect of the c-AMP/PKA pathway by the knockdown of AKAP4. In addition, compared to siNC, E-cadherin expression was greatly upregulated, and N-cadherin, EphA2, and MMP-2 () were significantly downregulated in the siRNA #1 and siRNA #2 groups, respectively (**p < 0.01, vs. siNC), revealing that the EMT progression in H460 xenograft tumor tissues was suppressed by the knockdown of AKAP4.
Figure 6. The c-AMP/PKA signaling in tumor tissues was activated by the knockdown of AKAP4. The expression level of AKAP4, c-AMP, and PKA was determined by immunohistochemical assay (**p < 0.01 vs. siNC). The controls were nude mice planted with H460 cells incubated with blank completed medium.
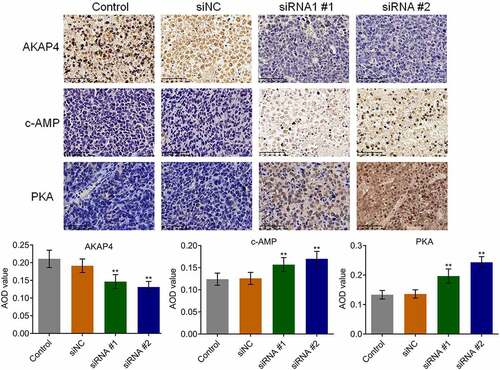
Figure 7. The EMT progression in tumor tissues was inhibited by the knockdown of AKAP4. The expression level of E-cadherin, N-cadherin, EphA2, and MMP-2 was determined by western blotting assay (**p < 0.01 vs. siNC). The controls were nude mice planted with H460 cells incubated with blank completed medium.
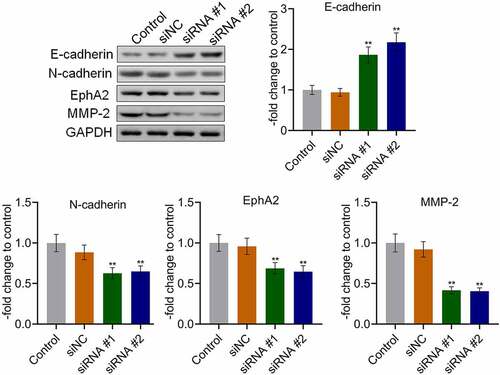
Discussion
High expression of AKAP4 and the biofunction of AKAP4 in the development of malignant tumors have been widely reported. Recently, AKAP4 has been proven to be a promising diagnostic and prognostic biomarker for colorectal cancer [Citation28], NSCLC [Citation19], breast cancer [Citation17], and ovarian serous carcinoma [Citation14]. Jagadish reported that AKAP4 promoted the development and progression of colorectal cancer by regulating EMT [Citation29]. Kumar claimed that the growth and survival of ovarian cancer cells were induced by AKAP4 through the regulation of c-AMP/PKA signaling [Citation30]. However, the role of AKAP4 in NSCLC development has rarely been reported. In the present study, we found that AKAP4 was significantly highly expressed in clinical NSCLC tissues and NSCLC cell lines, which verified the conclusion by Gumireddy [Citation19] that AKAP4 could be used as a potential biomarker for the diagnosis of NSCLC. We further knocked down the expression level of AKAP4 in H460 cells and significantly reduced cell viability, accompanied by increased apoptosis and decreased migration ability. Xenograft experiments verified the facilitation function of AKAP4 in the growth of H460 tumors. In addition, the in vitro and in vivo angiogenesis of HUVECs was suppressed by the knockdown of AKAP4. These functional experiments revealed that AKAP4 is a functional antigen that facilitates the development and progression of NSCLC.
Three domains have been reported to be involved in the structure of AKAP4, including a PKA binding domain, targeting sequence, and binding sites for other signaling molecules. AKAP4 binds to the R subunit of PKA through the PKA binding domain, leading PKA to its specific substrate for catalyzation. Recent reports have claimed that cAMP-PKA suppresses the proliferation, migration, and angiogenesis of malignant tumors [Citation31,Citation32]. In the present study, we found that the expression of PKA and production of c-AMP were negatively regulated by AKAP4 in both H460 cells and the H460 xenograft model, indicating that AKAP4 might promote the progression of NSCLC by regulating cAMP-PKA signaling, which might be related to the degradation of PKA induced by AKAP4 described previously [Citation30].
Tumor migration is mediated by multiple elements, such as EMT, the tumor microenvironment, and angiogenesis. EMT is a progression of transformation from epithelial cells to mesenchymal cells under specific pathological states, contributing to the migration and distant invasion of tumor cells. EMT progression is commonly accompanied by the downregulation of epithelial markers (E-cadherin and keratins) and upregulation of mesenchymal markers (N-cadherin, EphA2, and MMPs) [Citation33,Citation34]. In the present study, we found that EMT progression in H460 cells and xenograft tumor tissues was significantly suppressed by the knockdown of AKAP4, indicating a positive regulatory effect of AKAP4 on EMT progression, which was consistent with the report described in colorectal cancer [Citation29].
However, whether AKAP4 induced the development of NSCLC by regulating the cAMP-PKA pathway, EMT progression, or both needs to be further verified in our future work, such as the co-treatment of the inhibitor of the cAMP-PKA pathway or the activator of EMT progression in siRNA-AKAP4 transfected H460 cells. AKAP4 also binds to other signaling molecules (such as PKC, PP2B, PDE, and PP1) to induce interactions between PKA and these molecules. AKAP4 controls the activity of c-AMP/PKA signaling in the cytoplasm and nucleus via PKA-mediated phosphorylation and dephosphorylation by anchoring PKA to the appropriate site in cells [Citation35,Citation36]. In the future, we will explore more potential functional molecules that interact with AKAP4 to understand better the regulatory mechanism of AKAP4 in the development and processing of NSCLC.
Conclusion
In conclusion, our study has revealed that AKAP4 might be an important target for treating NSCLC because of its function in promoting the migration and proliferation of NSCLC cells.
Highlights
AKAP4 facilitates the development of NSCLC;
AKAP4 activates EMT progression;
AKAP4 inactivates the c-AMP/PKA pathway.
Consent for publication
Consent for publication has been achieved from all authors.
Ethic statements
We declare that all animal experiments involved in this manuscript were authorized by the ethical committee of Taizhou Hospital of Zhejiang Province and carried out according to the guidelines for care and use of laboratory animals and as well as to the principles of laboratory animal care and protection.
Ethics approval and consent to participate
We declare that all animal and human experiments involved in this manuscript were authorized by the ethical committee of Taizhou Hospital of Zhejiang province affiliated to Wenzhou Medical University and carried out according to the guidelines for care and use of laboratory animals and as well as to the principles of laboratory animal care and protection. All patients were aware of the purpose of the samples collected from them and agreed for participation.
Authors’ contributions
Conception and design of the research: Chunguo Wang
Acquisition of data: Jian Zhang and Lilong Xia
Analysis and interpretation of data: Zixian Jin
Statistical analysis: Yuhang Ruan
Drafting the manuscript: Bo Zhang
Revision of manuscript for important intellectual content: Quanteng Hu.
Disclosure statement
The authors declare there is no conflicts of interest regarding the publication of this paper.
Additional information
Funding
References
- Herbst RS, Morgensztern D, Boshoff C. The biology and management of non-small cell lung cancer. Nature. 2018;553:446–454.
- Kris MG, Johnson BE, Berry LD, et al. Using multiplexed assays of oncogenic drivers in lung cancers to select targeted drugs. JAMA. 2014;311:1998–2006.
- Jurisic V, Obradovic J, Pavlovic S, et al. Epidermal growth factor receptor gene in non-small-cell lung cancer: the importance of promoter polymorphism investigation. Anal Cell Pathol. 2018;2018:6192187.
- Jurisic V, Vukovic V, Obradovic J, et al. EGFR polymorphism and survival of NSCLC patients treated with TKIs: a systematic review and meta-analysis. J Oncol. 2020;2020:1973241.
- Rapoport AP, Stadtmauer EA, Binder-Scholl GK, et al. NY-ESO-1-specific TCR-engineered T cells mediate sustained antigen-specific antitumor effects in myeloma. Nat Med. 2015;21:914–921.
- Sakai K, Shimodaira S, Maejima S, et al. Dendritic cell-based immunotherapy targeting Wilms’ tumor 1 in patients with recurrent malignant glioma. J Neurosurg. 2015;123:989–997.
- Lynch TJ, Bondarenko I, Luft A, et al. Ipilimumab in combination with paclitaxel and carboplatin as first-line treatment in stage IIIB/IV non-small-cell lung cancer: results from a randomized, double-blind, multicenter phase II study. J Clin Oncol. 2012;30:2046–2054.
- Ma W, Gilligan BM, Yuan J, et al. Current status and perspectives in translational biomarker research for PD-1/PD-L1 immune checkpoint blockade therapy. J Hematol Oncol. 2016;9:47.
- Dronca RS, Liu X, and Harrington SM, et al. T cell Bim levels reflect responses to anti-PD-1 cancer therapy. JCI Insight. 2016 May 5;1(6): e86014.
- Dasgeb B, Mehregan D, Ring C, et al. Cancer-testis antigens as biomarkers for Merkel cell carcinoma: pitfalls and opportunities. J Cutan Pathol. 2019;46:748–752.
- Grizzi F, Mirandola L, Qehajaj D, et al. Cancer-testis antigens and immunotherapy in the light of cancer complexity. Int Rev Immunol. 2015;34:143–153.
- Park TS, Groh EM, Patel K, et al. Expression of MAGE-A and NY-ESO-1 in primary and metastatic cancers. J immunother (Hagerstown, Md: 1997). 2016;39:1–7.
- Lavergne V, Ghannoum M, Weiss K, et al. Successful prevention of respiratory syncytial virus nosocomial transmission following an enhanced seasonal infection control program. Bone Marrow Transplant. 2011;46:137–142.
- Agarwal S, Saini S, Parashar D, et al. The novel cancer-testis antigen A-kinase anchor protein 4 (AKAP4) is a potential target for immunotherapy of ovarian serous carcinoma. Oncoimmunology. 2013;2:e24270.
- Agarwal S, Saini S, Parashar D, et al. Expression and humoral response of A-kinase anchor protein 4 in cervical cancer. Int J Gynecol Cancer. 2013;23:650–658.
- Mirandola L, Yu Y, Jenkins MR, et al. Tracking human multiple myeloma xenografts in NOD-Rag-1/IL-2 receptor gamma chain-null mice with the novel biomarker AKAP-4. BMC Cancer. 2011;11:394.
- Saini S, Jagadish N, Gupta A, et al. A novel cancer testis antigen, A-kinase anchor protein 4 (AKAP4) is a potential biomarker for breast cancer. PLoS One. 2013;8:e57095.
- Chiriva-Internati M, Yu Y, Mirandola L, et al. Identification of AKAP-4 as a new cancer/testis antigen for detection and immunotherapy of prostate cancer. Prostate. 2012;72:12–23.
- Gumireddy K, Li A, Chang DH, et al. AKAP4 is a circulating biomarker for non-small cell lung cancer. Oncotarget. 2015;6:17637–17647.
- Lin X, Bai D, Wei Z, et al. Curcumin attenuates oxidative stress in RAW264.7 cells by increasing the activity of antioxidant enzymes and activating the Nrf2-Keap1 pathway. PloS One. 2019;14:e0216711.
- Jurisic V. Multiomic analysis of cytokines in immuno-oncology. Expert Rev Proteomics. 2020;17:663–674.
- Vieth M, Kushima R, Mukaisho K, et al. Immunohistochemical analysis of pyloric gland adenomas using a series of Mucin 2, Mucin 5AC, Mucin 6, CD10, Ki67 and p53. Virchows Arch. 2010;457:529–536.
- Jurisic V, Srdic-Rajic T, Konjevic G, et al. TNF-alpha induced apoptosis is accompanied with rapid CD30 and slower CD45 shedding from K-562 cells. J Membr Biol. 2011;239:115–122.
- Gentile MT, Pastorino O, Bifulco M, et al. HUVEC tube-formation assay to evaluate the impact of natural products on angiogenesis. J Vis Exp. 2019. DOI:10.3791/58591
- Justus CR, Leffler N, Ruiz-Echevarria M, et al. In vitro cell migration and invasion assays. J Vis Exp. 2014. DOI:10.3791/51046
- Ma Y, Ren Y, Dai ZJ, et al. IL-6, IL-8 and TNF-alpha levels correlate with disease stage in breast cancer patients. Adv Clin Exp Med. 2017;26:421–426.
- Yan C, Brunson DC, Tang Q, et al. Visualizing engrafted human cancer and therapy responses in immunodeficient zebrafish. Cell. 2019;177:1903–14 e14.
- Tavakoli Koudehi A, Mahjoubi B, Mirzaei R, et al. AKAP4, SPAG9 and NY-ESO-1 in Iranian colorectal cancer patients as probable diagnostic and prognostic biomarkers. Asian Pac J Cancer Prev. 2018;19:463–469.
- Jagadish N, Parashar D, Gupta N, et al. A-kinase anchor protein 4 (AKAP4) a promising therapeutic target of colorectal cancer. J Exp Clin Cancer Res. 2015;34:142.
- Kumar V, Jagadish N, Suri A. Role of A-Kinase anchor protein (AKAP4) in growth and survival of ovarian cancer cells. Oncotarget. 2017;8:53124–53136.
- Jiang K, Yao G, Hu L, et al. MOB2 suppresses GBM cell migration and invasion via regulation of FAK/Akt and cAMP/PKA signaling. Cell Death Dis. 2020;11:230.
- Wang S, Zhang Z, Qian W, et al. Angiogenesis and vasculogenic mimicry are inhibited by 8-Br-cAMP through activation of the cAMP/PKA pathway in colorectal cancer. Onco Targets Ther. 2018;11:3765–3774.
- Manning G, Whyte DB, Martinez R, et al. The protein kinase complement of the human genome. Science. 2002;298:1912–1934.
- van der Flier S, Chan CM, Brinkman A, et al. BCAR1/p130Cas expression in untreated and acquired tamoxifen-resistant human breast carcinomas. Int J Cancer. 2000;89:465–468.
- Malbon CC, Tao J, Wang HY. AKAPs (A-kinase anchoring proteins) and molecules that compose their G-protein-coupled receptor signalling complexes. Biochem J. 2004;379:1–9.
- Malbon CC, Tao J, Shumay E, et al. AKAP (A-kinase anchoring protein) domains: beads of structure-function on the necklace of G-protein signalling. Biochem Soc Trans. 2004;32:861–864.