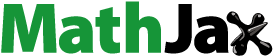
ABSTRACT
Agricultural residues are constantly increasing with increased farming processes, and improper disposal is detrimental to the environment. Majority of these waste residues are rich in lignocellulose, which makes them suitable substrate for bacterial fermentation in the production of value-added products. In this study, bacterial cellulose (BC), a purer and better form of cellulose, was produced by two Komagataeibacter sp. isolated from rotten banana and kombucha drink using corncob (CC) and sugarcane bagasse (SCB) enzymatic hydrolyzate, under different fermentation conditions, that is, static, continuous, and intermittent agitation. The physicochemical and mechanical properties of the BC films were then investigated by Fourier Transformed Infrared Spectroscopy (FTIR), Thermogravimetry analysis, Field Emission Scanning Electron Microscopy (FE-SEM), and Dynamic mechanical analysis. Agitation gave a higher BC yield, with Komagataeibacter sp. CCUG73629 producing BC from CC with a dry weight of 1.6 g/L and 1.4 g/L under continuous and intermittent agitation, respectively, compared with that of 0.9 g/L in HS medium. While BC yield of dry weight up to 1.2 g/L was obtained from SCB by Komagataeibacter sp. CCUG73630 under continuous agitation compared to that of 0.3 g/L in HS medium. FTIR analysis showed BC bands associated with cellulose I, with high thermal stability. The FE-SEM analysis showed that BC fibers were highly ordered and densely packed. Although the BC produced by both strains showed similar physicochemical and morphological properties, the BC produced by the Komagataeibacter sp. CCUG73630 in CC under intermittent agitation had the best modulus of elasticity, 10.8 GPa and tensile strength, 70.9 MPa.
GRAPHICAL ABSTRCAT
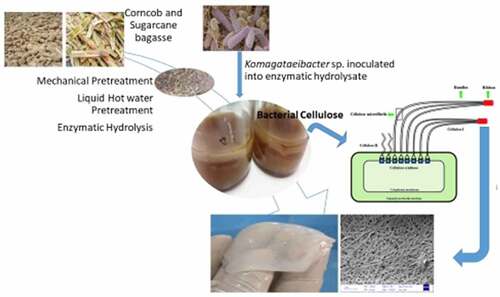
1. Introduction
Cellulose is a major component of plant cell wall and hence the most abundant polymer on earth, about 1.5 trillion tons of cellulose is produced annually [Citation1,Citation2]. Cellulose can also be synthesized by some fungi, bacteria, tunicates, and algae. Bacterial cellulose (BC) is the most common among the non-plant sources of cellulose, it serves as a suitable alternative for plant cellulose in various pharmaceutical and industrial applications. Moreover, BC is purer and has better physicochemical properties like higher crystallinity, tensile strength, and water-holding capacity over plant cellulose, although both these types present similar structural properties as they are made up of glucose monomers linked together by β-1, 4 glycosidic linkages [Citation3].
Several bacteria of different genus can produce cellulose [Citation4–6], but only strains of the genus Gluconacetobacter, with some strains renamed as Komagataeibacter, [Citation7] have been found to produce promising quantities of cellulose [Citation8,Citation9]. Regarding these promising strains, investigations for the utilization of cost-effective feedstock, as an alternative to expensive synthetic media, is very important. Agricultural residues are rich in lignocellulosic materials and are suitable substrates in different biological processes. Renewable low-cost agricultural residues and industrial by-products, such as fruit peels and juice, rice husk, molasses, wheat straw, palm date fruits, olive oil mill wastewater have been studied previously as substrates for BC production [Citation10–14]. Furthermore, there is an increased interest in the commercial applications of BC; thus, new cost-effective production technologies using innovative and cheap feedstocks, as well as the scaling up of bioprocess techniques for industrial applications have become more and more important [Citation15].
About 2 billion tons of agricultural wastes are accumulated globally, with an increase in the numbers over time [Citation16]. With intensified farming to combat hunger, there is a continuous development in crop production. This will in turn result in high amounts of agricultural wastes with negative environmental effect if not properly managed. Therefore, there is a need for these wastes to be transformed into sustainable value-added products. Nigeria is the largest producer of corn in Africa, with a production of 10.5 million metric tons in 2017 [Citation17]. The cob is the waste generated from corn processing after removing the grains. Furthermore, Nigeria is the second-largest sugar market in sub-Saharan African after South Africa, with sugarcane is mostly grown in Northern Nigeria where the weather and soil condition are most conducive. Foreign Agricultural Service (FAS) Lagos forecasts Nigeria’s domestic cane sugar production in marketing year (MY) 2021/22 to reach roughly 70,000 metric tons (raw value), down about 7% compared to 75,000 metric tons in MY 2020/21 [Citation18]. However, increasing sugar production through sugarcane farming, will lead to generation of large amounts of bagasse waste, as 1 ton of sugarcane will generate 270 kilos of bagasse [Citation19].
Corncob (CC) and sugarcane bagasse (SCB) are agro-wastes rich in lignocellulosic residues, generated in large quantities. In Nigeria, they are usually left to dry on the farm before been burnt off or they are found littering the streets and drainages [Citation20,Citation20]. This ineffective disposal methods results in environmental pollution, which further contributes to global climate challenges.
The challenge in utilization of lignocellulosic residues is their recalcitrant structure. These types of wastes must therefore undergo a pre-treatment, to break down their complex structure [Citation21]. Lignocellulosic materials are composed of cellulose, hemicellulose, and lignin, which are associated with each other in a heteromatrix. The aim with introducing a pre-treatment process step is to increase the accessibility of cellulose and hemicellulose for efficient enzymatic hydrolysis, as hydrolysis releases fermentable sugars, which in turn can be utilized by the microorganisms [Citation22].
Even though lignocellulosic materials have been used for BC production, in most of these cases, non-sustainable hydrolysis methods were applied [Citation23,Citation24]. Citation24,used CC acid hydrolyzate for BC production, Citation25,also used acetic acid pretreated bagasse for BC production, in previous studies. However, these acid hydrolyzates cannot be used directly for BC production, hence they need to be detoxified prior to their utilization. This additional process step would negatively affect the BC production process both environmentally and economically, there is therefore a need to investigate other chemical-free approaches within the BC production process.
There has been no study on enzymatic hydrolysis combined with mechanical pre-treatment of CC and SCB for production of BC. The aim of this study was therefore to investigate the effects of different pre-treatment steps using environmentally friendly methods prior to enzymatic hydrolysis. The hydrolyzates obtained from CC and SCB were then used as substrates for the bacterial growth and cellulose production under three different fermentation conditions that is, static or continuous, as well as intermittent-agitations, in order to optimize BC production by Komagataeibacter sp. Moreover, since the effect of different fermentation conditions on the physicochemical properties of BC has not yet been investigated, BC was finally characterized using Fourier Transformed Infrared Spectroscopy (FTIR), Field-Emission Scanning Electron Microscopy (FE-SEM), and Thermogravimetric Analysis (TGA). Furthermore, the mechanical properties which were determined with a Dynamic Mechanical Analyzer (DMA).
1.1 List of abbreviations
BC – Bacterial Cellulose
CC – Corncob
SCB – Sugarcane bagasse
LHW – Liquid Hot Water
MWA – Microwave Assisted
2. Materials and methods
2.1 Materials
The CC used was obtained from dumpsite at Oremeji area of Ibadan Oyo state Nigeria and the SCB was obtained from a sugarcane juice factory dumpsite in Ajah, Lagos state, Nigeria. The feedstocks were sundried immediately after collection and the dried samples were stored in air-tight plastic bags.
2.2 Pre-treatment of corncob and sugarcane bagasse
The sundried CC and SCB were milled using a locally fabricated grinding machine to particle size of about 0.5–2.0 mm for CC and 0.125–2.0 mm for SCB.
Pre-treatment of the substrates was performed using MWA and LHW pre-treatment methods. The MWA pre-treatment was carried out according to the method described by Citation26,using a microwave oven [Ethos Up, High Performance Microwave Digestion System]. Substrates were loaded into 10 mL vials at a loading of 0.2 g of substrate in 10 mL of solvent, using 0.4 M acetic acid as solvent, and treated at 120°C for 20 mins. The liquid hot water pre-treatment was performed according to the method of Citation27,using an oil bath [JULABO Circulator]. Samples were loaded into 150 mL stainless steel reactors, with a solid loading of 10% w/v (10 g substrate in 100 mL Milli-Q water). The reactors were then placed in an oil bath set to 160°C, for 10 min. Thereafter, the pre-treated samples were removed from the reactor as the solid fraction was separated from the liquid fraction. The solid fraction was then dried in an oven at 70°C overnight. Finally, the dried samples were stored in air tight plastic containers at room temperature prior to enzymatic hydrolysis.
2.3 Enzymatic hydrolysis of corncob and sugarcane bagasse
The microwave or liquid hot water pre-treated CC and SCB samples were enzymatically hydrolyzed by using a method described by Nair et al. [Citation28]. Cellulase enzyme, Cellic Ctec2 (Novozymes, Denmark), with an enzyme activity of 130 FPU/mL was applied, and the substrate loading used was 7.5% w/v, with an enzyme load of 15 FPU/g dry weight of substrate. The hydrolysis was performed using 250 mL Erlenmeyer flasks with working volume of 200 mL at pH 5.5 ± 0.2, and at 35°C with 125 rpm in a shaking water bath (Grant OLS 200, Grant instrument Ltd, UK). Samples were taken regularly, at 0, 4, 8, 12, 36 and 48 hours. The amounts of sugars released were quantified using high-performance liquid chromatography (HPLC).
2.4 Isolation and identification of cellulose producing bacteria
Cellulose producing bacteria was isolated either from rotten banana gotten from Oje market in Ibadan, Nigeria or from Kombucha drink (Roots of Malmö Kombucha), in Sweden. Approximately 1 g of the banana sample was incubated in 4% ethanol for 7 days [Citation29], and furthermore, 1 mL of the kombucha drink was incubated in sterile saline at 30°C with shaking at 100 rpm in a water bath shaker (Grant OLS 200, Grant instrument Ltd, UK) for 18–24 hours. The samples were serially diluted with sterile water and spread onto GYC agar containing (g L−1) glucose, 3; yeast [Citation30]extract,10; CaCO3, 10; agar, 15. Colonies that produced clear zone of solubilization of CaCO3 were selected and purified. The colonies were purified by repeated streaking on Hestrin and Schramm (HS) Agar (2% glucose, 0.5% yeast extract, 0.5% peptone, 0.115% citric acid, 0.27% Na2HPO4, 1.5% agar) at pH 6.0, and incubated at 30°C for 3 days [Citation31]. The purified cultures were grown in HS broth and the production of pellicle at the air-liquid interface of the medium was then followed up. The selected strains were stored in 30% glycerol broth at −20°C prior to further use.
Identification of the selected cellulose-producing strains was done using 16S rRNA gene sequence analysis at the Culture Collection of the University of Gothenburg, Sweden. The DNA of the isolates were extracted, and amplified using Polymerase chain reaction (PCR), thereafter, the gene were sequenced, using the method of Citation32. The sequences were compared to known sequences in the Genebank. The two strains selected for further investigations were identified as Komagataeibacter sp. CCUG73629 (Accension number OM779139) and Komagataeibacter sp. CCUG73630 (Accension numbers OM779138)
2.5 Production of biocellulose using corncob and sugarcane bagasse hydrolyzates
Precultures of Komagataeibacter sp. CCUG73629 and Komagataeibacter sp. CCUG73630 were performed in flasks containing HS medium and incubated statically at 30°C for 2–3 days. The pH was adjusted to 6.0 using 5 M NaOH or 5 M HCl prior to incubation. The enzymatic hydrolyzates of CC and SCB supplemented with other nutrients () were then used as substrates for the BC production applying static conditions, continuous agitation, or intermittent agitation. Seed cultures, prepared as described above, were transferred into the CC or SCB enzymatic hydrolyzates (), achieving 5 mL of seed culture in 100 mL of hydrolyzate for each assay, and then the fermentation was performed in blue-capped bottles that were loosely capped under the three different conditions at 30°C for 10 days, as it is shown in . Samples were taken regularly from all assays to monitor the substrate consumption pattern of the strains during BC production. All assays were performed in duplicates.
Table 1. The composition of different fermentation media for bacterial cellulose production under different conditions
2.6 Treatment and purification of bacterial cellulose
After fermentation, BC pellicles were harvested and purified using 1 M NaOH at 80°C for 1 hour, to remove all remnant cells and medium components and then washed with distilled water until pH 7 was reached. Finally, the BC pellicles were air dried overnight and then kept in air-tight plastic bags until further investigations.
2.7 Analytical methods
Compositional analysis of the untreated and pre-treated CC and SCB was done using the NREL method to determine the total solids, structural carbohydrate, and lignin components [Citation33]. The concentration of the various components in the hydrolyzate and/or fermentation medium was analyzed by HPLC (Walters Corporation, Milford, USA). A hydrogen-based column (Aminex HPX-87 H, Bio-Rad, Hercules, USA) working at 60°C using 0.6 mL/min of 5 mM H2SO4 solution as the eluent, was used for the detection and quantification of sugars, acetic acid, and glycerol.
At the end of the fermentation, the final pH of the broth was recorded using a pH meter. The dry weight of the BC pellicle after drying was determined and expressed as gram dry weight of cellulose per liter of fermenting medium (g/L). BC yield and moisture content were determined as follows;
% BC Yield =
BC dry weight (g/L) = the weight of BC after drying
Carbon Source used (g/L) = the amount of the carbon source used for BC production in g/L.
% Moisture content =
2.8 Statistical analysis
Statistical analysis of the results obtained were performed using MINITAB 17.0 Software. Analysis of variance (ANOVA) was performed using general linear models with 95% confidence interval, followed by Tukey’s pairwise comparison test. All experiments were performed in duplicate and error bars presented on the graphs represent two standard deviations.
2.9 Characterization of bacterial cellulose.
2.9.1 Fourier Transformed Infra-red spectroscopy (FTIR)
FTIR was performed using a FTIR spectrometer (Nicolet iS10, Thermo Fisher Scientific, Waltham, USA). The BC samples were analyzed by placing the dried film on the diamond accessory. The FTIR spectra were recorded in the range of 4000–500 cm−1 wavenumbers, with an accumulation of 32 scans.
2.9.2 Field-Emission Scanning Electron Microscopy (FE-SEM)
Surface morphology of the BC film was studied by FE-SEM (Zeiss, Sigma, Germany) imaging. The films were attached to a carbon tape and covered with gold. Photomicrographs were taken at 8,000, 15,000, and 20,000 x magnifications, using an accelerating voltage of 5 kV.
2.9.3 Thermogravimetric Analysis (TGA)
Thermogravimetric analysis of dried BC films was performed on a TA instrument (Q500 TA instrument, Waters LLC, New Castle, DE, USA), to determine the thermal properties of the BC. The samples, with a weight of 5–10 mg, were heated in aluminia pans from room temperature to 600°C at a heating rate of 20°C/min in a nitrogen atmosphere with a flow rate of 10.0 mL/min.
2.9.4 Mechanical Properties
The mechanical properties of BC were determined by a dynamic mechanical analyzer (DMA) (DMA Q800, TA instruments, Waters LLC, USA). The analysis was operated in a stress/strain mode, using a tension film clamp. The film was cut in a typical width of 5.3 mm, with a length of approximately 17 mm. The test was performed at room temperature. The stress (σ), strain (ℇ and Young’s modulus were then calculated.
3 Results and discussion
Generally, the production of BC has a direct impact on its supramolecular structure, mechanical and physical properties [Citation15], there is therefore a need to investigate cost-effective and sustainable technologies, substrates, culture conditions as well as different strains for BC production. This study investigated BC production, using CC and SCB as substrates. Both CC and SBC are widely available cheap lignocellulose-rich substrates, however, due to the recalcitrant structure of lignocelluloses, these types of substrates need to be subjected to pretreatment aiming to liberate fermentable sugars. Environmentally friendly LHW, and MWA pretreatment methods were applied to break up the lignocellulose structure prior to enzymatic hydrolysis. The obtained enzymatic hydrolyzates were then subjected to fermentation using two different Komagataeibacter sp. under different conditions, i.e., static, as well as using continuous and intermitten agitation. Finally, physicochemical properties of the produced BC were determined using methods as FTIR, FE-SEM, TGA, and DMA.
3.1 Pre-treatment and enzymatic hydrolysis of the substrates
Pre-treatment of CC and SCB, using MWA and LHW pre-treatment methods, was investigated, in order to determine the best method for enhanced enzymatic hydrolysis.
Pre-treatment of lignocellulosic biomass is generally applied aiming to improve enzymatic digestibility of the substrate [Citation26]. Enzymatic hydrolysis of both untreated and pre-treated CC and SCB resulted in higher glucose concentrations than that of pentoses (), due to a higher cellulose content compared to that of hemicelluloses, as it was also determined by the compositional analysis of the untreated substrates. Furthermore, the pre-treatment applied resulted in a somewhat higher released sugars after the enzymatic hydrolysis in case of CC, however the difference obtained for untreated vs treated CC was not statistically significant (). Since according to the results, there was no difference obtained regarding the effectiveness of MWA or LHW, LHW pre-treatement was chosen for the subsequent investigations, as it is highly efficient and economically feasible, as well as environmentally friendly, reducing the usage of chemicals and their effects on the environment. The LHW dissolves hemicelluloses and lignin, which are transferred into the liquid phase, while leaving cellulose as solid. Consequently, the biomass digestibility increases as cellulose becomes more accessible to enzymatic hydrolysis, where partial hydrolysis of cellulose can occur as a result of acetic acid formation [Citation34].
Table 2. Sugar concentrations obtained in enzymatic hydrolyzates of untreated and pre-treated corncob (CC) and sugarcane bagasse (SCB) after 48 hrs of enzymatic hydrolysis
Enzymatic hydrolysis of SCB showed that the glucose and pentose yield was higher (20.6 g/L and 12.6 g/L respectively) in the hydrolyzate of untreated (milled) SCB than those in the hydrolyzate of LHW pre-treated SCB, which had glucose and pentose yield of 15.8 g/L and 9.6 g/L, respectively (). This is contrary to the report of Citation26, who recorded higher C5 and glucose yields after using MWA oxalic acid pre-treatment and then hydrolysis. However, in this study a milled, that is, mechanically pre-treated SCB was subjected to further pre-treatment with only LHW, as the samples were lost during MWA pre-treatment experimentation due to technical difficulties. Milling aiming to reduce the particle size, will lead to an increase in accessible surface area, which in turn can improve enzymatic digestibility [Citation26]. Moreover, milling as a mechanical size reduction provides a non-chemical, green route for pre-treatment of lignocellulosic materials [Citation22].
3.2 Bacterial cellulose production using lignocellulosic biomass as substrate
When lignocellulosic biomass is subjected to a pre-treatment one of the goals is to make cellulose accessible for the subsequent hydrolysis steps to release sugars. It may rise the question, why do one need to convert cellulose present in lignocellulosic biomass to cellulose produced by bacteria. The production of bacterial cellulose has the benefits of purer cellulose without lignin and hemicellulose, easy to extract, nontoxic, biodegradable with human compatibility. After enzymatic hydrolysis of a substrate, the hydrolyzate may contain compounds that can potentially stimulate the synthesis of a polysaccharide or substances, which may act inhibitory to cell growth and metabolism [Citation35]. Bacteria of different genera have the ability to utilize different sugars for growth and metabolism. Komagataeibacter sp. are acetic acid bacteria known to produce cellulose in large quantities, as they also have the ability to utilize a wide variety of substrates [Citation8].
In this study, BC production from CC and SCB hydrolyzate was investigated using two different strains of Komagataeibacter sp., Komagataeibacter sp. CCUG73629 and Komagataeibacter sp. CCUG73630. Both strains showed the ability to consume glucose and pentose sugars during fermentation leading to the production of bacterial cellulose.
Generally, during cellulose synthesis, glucose acts as an energy source as well as a precursor for the synthesis [Citation36]. Media supplementation with nitrogen and phosphate sources improved BC production and yield. Moreover, it was found that the use of agitation will also enhance BC production, because better air diffusion into the media and then to the cells will increase metabolic activity and thereby the production rate of BC [Citation37]. Hence, aerobic cells, like acetic acid bacteria, will access higher oxygen circulation, which may enhance their metabolic activity. Furthermore, there was a reduction in the pH observed after BC production by both strains in the production media (), which may be the result of the accumulation of acids produced by the strains. These strains are acetic acid bacteria, with the ability to synthesize acetic acid, and moreover these strains have also the ability to utilize the acetic acid that has been synthesized [Citation38].
Table 3. Bacterial cellulose production by komagataeibacter sp. CCUG73629 from corncob (CC) at different fermentation conditions
Table 4. Bacterial cellulose production by komagataeibacter sp. CCUG73630 from corncob (CC) at different fermentation conditions
Table 5. Bacterial cellulose production by komagataeibacter sp. CCUG73629 from sugarcane bagasse (SCB) at different fermentation conditions
Table 6. Bacterial cellulose production by Komagataeibacter sp. CCUG73630 from sugarcane bagasse (SCB) at different fermentation conditions
During BC production, the highest BC yields, i.e., 14.1 and 11.9% could be achieved at respectively, continuous and intermittent agitation conditions by Komagataeibacter sp. CCUG73629 in CC hydrolyzate (). While defined HS medium gave the highest BC yield by Komagataeibacter sp. CCUG73630, nevertheless it was much lower, i.e., 4.5% () than those observed in any conditions in CC hydrolyzate, whereas Komagataeibacter sp. CCUG73629 gave a yield of 7.9% in defined HS medium (). This means that, the complex composition of CC hydrolyzate clearly favored the BC production process by Komagataeibacter sp. CCUG73629. The produced dry weight of BC obtained during continuous and intermittent agitation i.e. 1.6 g/L and 1.4 g/L, respectively (), were higher than that of 0.65 g/L observed during agitation in CC acid hydrolyzate reported by Citation24.
Figure 1. Dry weight of BC produced by Komagataeibacter sp. CCUG73629 and Komagataeibacter sp. CCUG73630 in corncob (CC) hydrolyzate at different fermentation conditions.
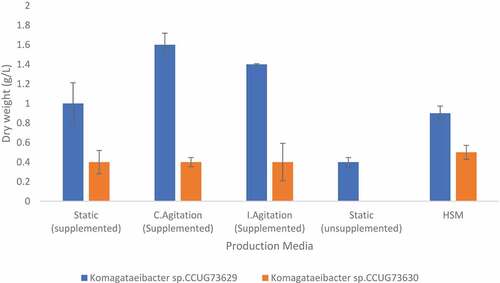
Although, the use of HS medium supported a higher yield of BC produced by Komagataeibacter sp. CCUG73630, because the strain thrives best in glucose predominant medium, which was also confirmed here in this study. Previous reports on the production of BC using wastes and by-products shows that the utilization of some of the waste materials resulted in higher BC yield than those obtained with the use of HS medium. For instance, waste from lipid fermentation resulted in a BC production of 0.4–0.6 g/L [Citation39] while from molasses the production of BC was 1.64 g/L [Citation40], and using olive oil mill wastewater as substrate gave a higher BC of 5.33 g/L than that observed in HS medium [Citation14]. However, Citation41,reported a higher BC production in HS (6.7 g/L) than that from cashew tree exudate and cashew gum (2.8 g/L and 2.3 g/L, respectively). Citation42,also reported that BC produced by Gluconacetobacter xylinum from polysaccharide wastewater was lower (1.177 g/L) than that produced by the same strain in HS medium (1.757 g/L).
There was no significant difference between the BC yields observed under static vs agitation conditions in cases when Komagataeibacter sp. CCUG73630 was cultivated in CC hydrolyzate, meaning that different cultivation conditions did not have any significant effect on the BC yield (). This is in line with Citation43, who found that there was no statistically significant difference in the yield of cellulose produced by Gluconacetobacter xylinus in the same media under static and agitated condition. They explained that although oxygen might be a limiting factor in cellulose production, however, allowing access to more oxygen by agitating did not increase the cellulose yields.
In SCB hydrolyzate, continuous agitation increased the BC yield in case of both strains, i.e., 9.4% and 12.7% were observed with Komagataeibacter sp. CCUG73629 () and Komagataeibacter sp. CCUG73630 (), respectively. While intermittent agitation resulted in a higher BC yield (7.9%) for Komagataeibacter sp. CCUG73629 () than that (4.7%) with Komagataeibacter sp. CCUG73630 (). The production of BC was higher, i.e., 1.2 g/L dry weight, under continuous agitation in SCB hydrolyzate () than that recorded by Citation38,who reported a production of 1.09 g/L and 0.42 g/L in bagasse acid and enzymatic hydrolyzate, respectively.
Figure 2. Dry weight of BC produced by Komagataeibacter sp. CCUG73629 and Komagataeibacter sp. CCUG73630 in sugarcane bagasse (SBC) hydrolyzate at different fermentation conditions.
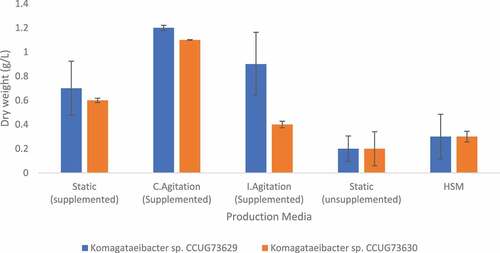
Agitation generally enhanced BC yield greatly compared to that observed under static culture condition. In static condition, as BC mass increases in the medium, oxygen circulation reduces, with cells having little or no access to oxygen. Oxygen has been reported to be a limiting factor in bacterial cellulose production [Citation44], thus allowing access to oxygen via agitation increases even access to nutrients and thereby increases metabolic activity [Citation45]. Aydin and Aksoy [Citation46] reported 120 rpm as the best agitation condition for cell growth and BC production by Gluconacetobacter hansenii P2A, while Citation37,reported agitation at 200 rpm for BC production by Gluconacetobacter hansenii NCIM 2529. Citation47,reported 150 rpm as the maximum agitation speed for cellulose production by Acetobacter xylinum KJ-1.
The low yield of BC in the CC hydrolyzate not supplemented with nitrogen and phosphate sources, i.e., 2.6% and zero production, by Komagataeibacter sp. CCUG73629 () and Komagataeibacter sp. CCUG73630 (), respectively, emphasizes the importance of nutritional supplements in a culture medium to favor BC production [Citation48]. Also, a lower yield of BC i.e. 1.7% and 1.2% by Komagataeibacter sp. CCUG73629 () and Komagataeibacter sp. CCUG73630 (), respectively, was recorded in the unsupplemented SCB hydrolyzate, when compared with those produced in SCB hydrolyzate supplemented with nitrogen and phosphate sources. Yeast extract and peptone as organic nitrogen source, when added to culture medium for BC production is indispensable for a significant BC production [Citation13,Citation49]. This also agrees with Coban and Biyik [Citation50], who reported a higher BC production in a glucose media supplemented with yeast extract as nitrogen source.
3.3 Characterization of bacterial cellulose
After production of a biomaterial, it is important to investigate the structural features because the physicochemical properties of such materials can be influenced by the composition and physical properties of the culture medium. In this study, it was observed that the fermentation condition and type of substrate had impact on some of the structural and physicochemical properties.
3.3.1 Chemical structure of bacterial cellulose
The spectra of all BC produced under different conditions were identical and exhibited characteristic bands of cellulose I. The hydroxyl, aldehyde, alkane, and alkene functional groups were present in all samples. The functional groups and fingerprint regions associated with cellulose can be found between 1800 and 500 cm−1; that is, peaks around 1647 cm−1 indicating CO stretching, peaks around 1427 cm−1 indicating – OH bending, peaks around 1160 cm−1 indicating C-O-C asymmetric stretching at β – glycosidic linkage, peaks around 1108 cm−1 indicating C-O bond stretching, peaks around 1030 cm−1 indicating C-O-C ring skeletal vibration, and peaks around 1314 cm−1 indicating CH2 wagging at C-6 were associated with cellulose. It was observed that only BC produced under agitation in SCB by Komagataeibacter sp. CCUG73629 (), had slightly different spectra, presenting two peaks at around 2919 and 2851 cm−1representing C-H stretching vibration of sugar rings. These probably occur due to the agitation as only single peaks were observed for BC obtained at static conditions. Similar functional groups and peaks for BC have been reported by various researchers [Citation51–53].
Figure 3. FTIR spectra of BC produced by Komagataeibacter sp. CCUG73629 from sugarcane bagasse (SCB) hydrolyzate at different fermentation conditions.
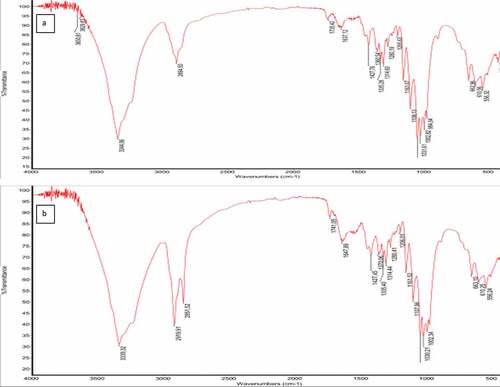
The absence of peaks around 1540 cm−1 and 1640 cm−1 which corresponds to amine bonds, are associated with proteins from culture media or residual bacterial biomass. Their absence indicates that the BC membrane was properly cleaned and is pure [Citation54,Citation54].
The absence of peaks around 3440 cm−1 to 3495 cm−1 indicating OH stretching due to intramolecular hydrogen bonds confirms the absence of cellulose II [Citation55,Citation56]. This therefore confirms that the BC produced by Komagataeibacter sp. CCUG73629 and Komagataeibacter sp. CCUG73630 under different fermentation conditions is cellulose I.
3.3.2 Morphology of bacterial cellulose
The morphology of BC determined using SEM considers the fibril density, size, and arrangement, which can be dependent on the media composition, viscosity, and activity of the BC producing bacteria [Citation45,Citation57].
BC produced by Komagataeibacter sp. CCUG73629 and Komagataeibacter sp. CCUG73630 in SCB and CC hydrolyzate medium were densely packed showing thin BC fibers (). Although, most of the BC fibers displayed longer fibrous networks, they had varying microfibril diameters ranging from 42 to 120 nm. The densely packed network of cellulose with thinner fibers indicate that BC has more hydrogen-bonding pattern, a more compact pattern that may result in higher tensile strength of the BC [Citation58].
Figure 4. Scanning electron micrograph of BC produced from corncob (CC) hydrolyzate by Komagataeibacter sp CCUG73629 (a-c) and Komagataeibacter sp CCUG73630 (d-f) under (a, d) Static, (b, e) Intermittent agitation, and (c, f) Continuous agitation conditions at a magnification of 20,000 X.
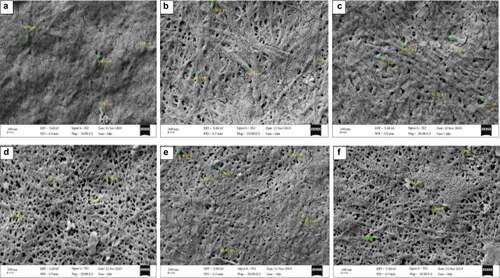
Figure 5. Scanning electron micrograph of BC produced from sugarcane (SCB) hydrolyzate by Komagataeibacter sp. CCUG73629 (a-c) and Komagataeibacter sp. CCUG73630 (d-f) under (a, d) Static, (b, e) Intermittent agitation and (c, f) Continuous agitation conditions at a magnification of 15,000 X.
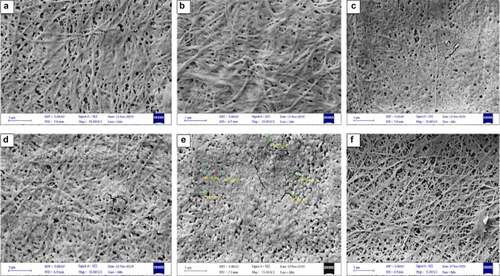
Citation10,stated that no major variations were observed in the dimension of the four bacterial nano cellulose produced. However, in the present study, variations were observed in the BC morphology, which we believe are due to the different fermentation conditions, bacterial strains, and the production medium applied. For example, a porous network with thin fibers were observed in BC produced by Komagataeibacter sp. CCUG73630 under static condition in CC hydrolyzate () than BC produced by Komagataeibacter sp. CCUG73629 under static condition () in the same medium. The porous nature within the fibril arrangement gives BC high porosity and water accumulating properties, which are responsible for water retention, an important property for application in biomedicine [Citation45].
3.4.3 Thermal properties
The thermal properties of BC is determined by its thermal stability during degradation. Cellulose degradation shows loss of weight due to degradation and decomposition of the glycosyl units. The BC produced by Komagataeibacter sp. CCUG73629 and Komagataeibacter sp. CCUG73630 in CC and SCB hydrolyzate medium under all fermentation conditions showed higher stability, i.e. (396–364°C) and (314–399°C), respectively, except those produced by Komagataeibacter sp. CCUG73630 under continuous agitation in SCB (314°C) (). Furthermore, the BC produced in the CC and SCB hydrolyzate by Komagataeibacter sp. CCUG73629 and Komagataeibacter sp. CCUG73630 showed higher thermal stability than those produced in the defined HS medium i.e. 353°C and 346°C, respectively (). BC-producing medium can form a more effective chemical interactions (like hydrogen bonds) with hydroxyls group of bacterial cellulose and hence increase its thermal stability [Citation41]. Thermal degradation of cellulose skeleton starts around 220°C and overall degradation is completed above 300°C; thus, the variations in the degradation temperature may be due to the variations in fibril size, arrangement, and compactness, with higher thermal degradation indicating higher crystallinity [Citation45]. Maximum degradation temperature is a criterion for thermal stability.
Table 7. Maximum degradation temperature of bacterial cellulose produced by Komagataeibacter sp. CCUG73629 and Komagataeibacter sp. CCUG73630 from corncob (CC) and sugarcane bagasse (SCB) hydrolyzate
3.4.4 Mechanical properties
The mechanical properties of BC are dependent on the physical nature of the fibrils together with the strength of intermolecular hydrogen bonding between the cellulose chains [Citation59]. Moreover, the fermentation condition can also influence the mechanical properties of BC. DMA was used for these investigations in this study due to the size of the BC film obtained. BC produced by Komagataeibacter hansenii CCUG73629 in HS medium had a high modulus of elasticity (7.1 GPa) and tensile strength (76.2 MPa) (). Intermittent agitation increased the mechanical properties of BC produced by both strains in CC hydrolyzate medium () and Komagataeibacter sp. CCUG73630 in SCB hydrolyzate medium (). BC produced under intermittent agitation by Komagataeibacter sp. CCUG73629 in CC had modulus of 7.0 GPa and tensile strength of 57.8 MPa (). In addition, BC produced by Komagataeibacter sp. CCUG73630 under intermittent agitation in CC had modulus of 10.8 GPa and tensile strength of 70.9 MPa, while in SCB, had modulus of 9.9 GPa and tensile strength of 58.4 MPa (). The high modulus of elasticity of BC produced by both strains under intermittent agitation showed that the intermittent agitation supported production of a stiffer BC, with strong hydrogen bonding.
Figure 6. Tensile strength, Elongation at break and Young’s modulus of BC produced by Komagataeibacter sp. CCUG73630 from sugarcane bagasse (SCB) and corncob (CC) hydrolyzate at different fermentation conditions.
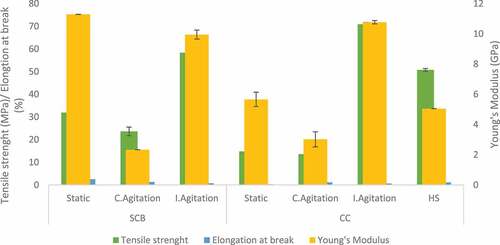
Figure 7. Tensile strength, Elongation at break and Young’s modulus of BC produced by Komagataeibacter sp. CCUG73629 from sugarcane bagasse (SCB) and corncob (CC) hydrolyzate at different fermentation conditions.
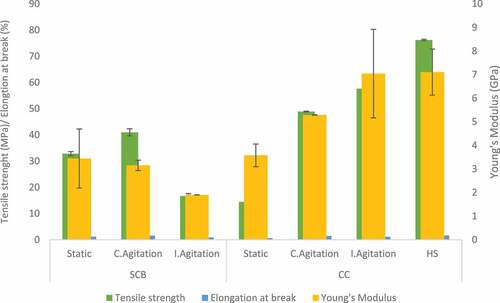
Citation42,reported that BC produced by G. xylinum in HS medium had a higher modulus of elasticity, tensile strength, and strain at break when compared to BC produced in polysaccharide fermentation wastewater. Citation60,also reported that BC produced in glucose, yeast extract, and peptone medium by G. hansenii had tensile strength of 76.7 MPa. Citation56,also reported tensile strength of 46.9 MPa and a modulus of 3.2 GPa for BC produced by Komagataeibacter rhaeticus.
The mechanical properties depicts high strength of BC samples, as the fibril size and arrangement also influences these properties. Uniform size of well-arranged fibrils provides higher strength to BC [Citation45]. The modulus of elasticity of a polymer relates directly to the stiffness of the material, i.e., the higher the modulus of elasticity, the stiffer the material [Citation61].
Conclusion
Bacterial cellulose, an extracellular matrix secreted by bacteria is an appropriate alternative to plant cellulose, with excellent physicochemical properties that makes it suitable for several applications. This research showed that CC and SCB after applying mechanical and LHW pretreatments prior to enzymatic hydrolysis are suitable substrates for BC production. Intermittent agitation proved to be a promising alternative to continuous agitation with good BC yield and properties. Furthermore, it was also found that different fermentation conditions can influence the physicochemical properties of the BC.
Acknowledgements
This work was supported by the Swedish Centre for Resource Recovery, University of Borås, SE 501 90 Borås, Sweden.
Disclosure statement
No potential conflict of interest was reported by the author(s).
References
- McNamara JT, Morgan JL, Zimmer J. A molecular description of cellulose biosynthesis. Annu Rev Biochem. 2015;84(1):895–921.
- Moniri M, Moghaddam AB, Azizi S, et al. Production and status of bacterial cellulose in biomedical engineering. Nanomaterials. 2017;7(257):1–26.
- Bayon B, Berti IR, Gagneten AM, et al. 2018. Waste to wealth. Singhania RK, Rani R, Kumar RP, et al ed. January
- Brown RM. Cellulose structure and biosynthesis: what is in store for the 21st century? J Polym Sci A Polym Chem. 2004;42(3):487–495.
- Deinema MH, Zevenhuizen LP. Formation of cellulose fibrils by gram-negative bacteria and their role in bacterial flocculation. Arch Microbiol. 1971;78(1):42–51.
- Morgan JLW, Strumillo J, Zimmer J. Crystallographic snapshot of cellulose synthesis and membrane translocation. Nature. 2013;493(7431):181–186.
- Tang WH, Jia SR, Jia YY, et al. The influence of fermentation conditions and post-treatment methods on porosity of bacterial cellulose membrane. World J Microbiol Biotechnol. 2010;26(1):125–131.
- Mohammadkazemi F, Azin M, Ashori A. Production of bacterial cellulose using different carbon sources and culture media. Carbohydr Polym. 2015;117:518–523.
- Tanskul S, Amornthatree K, Jaturonlak N. A new cellulose-producing bacterium, rhodococcus sp. MI 2: screening and optimization of culture conditions. Carbohydr Polym. 2013;92(1):421–428.
- Abol-Fotouh D, Hassan MA, Shokry H, et al. Bacterial nanocellulose from agro-industrial wastes: low-cost and enhanced production by komagataeibacter saccharivorans MD1. Sci Rep. 2020;10(1):3491.
- Adebayo-Tayo BC, Akintunde MO, Alao SO. Comparative effect of agrowastes on bacterial cellulose production by acinetobater sp BAN1 and Acetobacter pasteurianus PW1. Turkish J Agric Nat Sci. 2017;4(2):145–154.
- Gomes FP, Silva NHCS, Trovatti E, et al. Production of bacterial cellulose by gluconacetobacter sacchari using dry olive mill residue. Biomass Bioenergy. 2013;55:205–211.
- Kurosumi A, Sasaki C, Yamashita Y, et al. Utilization of various fruit juices as carbon source for production of bacterial cellulose by acetobacter xylinum NBRC 13693. Carbohydr Polym. 2009;76(2):333–335.
- Sar T, Akbas MY. Potential use of olive oil mill wastewater for bacterial cellulose production. Bioengineered. 2022;13(3):7659–7669.
- Reshmy R, Eapen P, Deepa T, et al. Bacterial nanocellulose: engineering, production, and applications. Bioengineered. 2021;12(2):11463–11483.
- Millati R, Cahyono RB, Ariyanto T, et al. Chapter 1 – agricultural. In: Taherzadeh MJ, Bolton K, Wong J, et al, editors. Industrial, municipal, and forest wastes: an overview. Elsevier; 2019. p. 1–22. Sustainable Resource Recovery and Zero Waste Approaches.
- FAO, 2017. GIEWS - Global Information and Early Warning System: country briefs, Nigeria. http://www.fao.org/giews/countrybrief/country.jsp?code=NGA
- Boluwade E. Sugar annual report (NI2021-0003). approved by Gerald Smith. In: Global Agricultural Information Network (GAIN), United States Department of Agriculture (USDA), Foreign agricultural services. Lagos Nigeria; 2021. p. 1–10.
- Ogwo JN, Dike OC, Matthew SC, et al. Overview of biomass energy production in Nigeria: implication and challenges. Asian J Nat Appl Sci. 2012;1(4):48–51.
- Mohlala LM, Bodunrin MO, Awosusi AA, et al. Beneficiation of corncob and sugarcane bagasse for energy generation and materials development in Nigeria and South Africa : a short overview. Alexandria Eng J. 2016;55(3):3025–3036.
- Liu H, Zhang YX, Hou T, et al. Mechanical deconstruction of corn stover as an entry process to facilitate the microwave-assisted production of ethyl levulinate. Fuel Process Technol. 2018;174:53–60.
- Kucharska K, Rybarczyk P, Hołowacz I, et al. Pretreatment of lignocellulosic materials as substrates for fermentation processes. Molecules. 2018;23(11):1–32.
- Al-Abdallah W, Dahman Y. Production of green biocellulose nanofibers by Gluconacetobacter xylinus through utilising the renewable resources of agriculture residues. Bioprocess Biosyst Eng. 2013;36(11):1735–1743.
- Huang C, Yang XY, Xiong L, et al. Utilization of corncob acid hydrolysate for bacterial cellulose production by Gluconacetobacter xylinus. Appl Biochem Biotechnol. 2015;175(3):1678–1688.
- Cheng Z, Yang R, Liu X. Production of bacterial cellulose by acetobacter xylinum through utilizing acetic acid hydrolysate of bagasse as low-cost carbon source. BioResources. 2017;12(1):1190–1200.
- Yan Y, Zhang C, Lin Q, et al. Microwave-Assisted oxalic acid pretreatment for the enhancing of enzyme hydrolysis in the production of xylose and arabinose from bagasse. Molecules. 2018;23(4):1–13.
- Imman S, Laosiripojana N, Champreda V. Effect of liquid hot water pretreatment on enzymatic hydrolysis and physicochemical changes of corncobs. Appl Biochem Biotechnol. 2018;184(2):432–443.
- Nair RB, Lundin M, Brandberg TL, et al. Dilute phosphoric acid pretreatment of wheat bran for enzymatic hydrolysis and subsequent ethanol production by edible fungi Neurospora intermedia. Ind Crops Prod. 2015;69:314–323.
- Diba F, Alam F, Talukder AA. Screening of acetic acid producing microorganisms from decomposed fruits for vinegar production. Adv Microbiol. 2015;5(5):291–297.
- Dubey S, Singh J, Singh RP. Biotransformation of sweet lime pulp waste into high-quality nanocellulose with an excellent productivity using komagataeibacter europaeus SGP37 under static intermittent fed-batch cultivation. Bioresour Technol. 2018;247:73–80.
- Hestrin S, Schramm M. Synthesis of cellulose by acetobacter xylinum. 2. Preparation of freeze-dried cells capable of polymerizing glucose to cellulose. Biochem J. 1954;58(2):345–352.
- Yamada Y, Yukphan P, LanVu HT, et al. Description of Komagataeibacter gen. nov., with proposals of new combinations (Acetobacteraceae). J Gen Appl Microbiol. 2012;58(5):397–404.
- Sluiter A, Hames B, Ruiz R, et al. 2012. NREL laboratory analytical procedure for determination of structural carbohydrates and Lignin in biomass. Technical Report; NREL/TP-510-42618.
- Kumar P, Barrett DM, Delwiche MJ, et al. Methods for pretreatment of lignocellulosic biomass for efficient hydrolysis and biofuel production. Ind Eng Chem Res. 2009;48(8):3713–3729.
- Bae SO, Shoda M. Production of bacterial cellulose by Acetobacter xylinum BPR2001 using molasses medium in a jar fermentor. Appl Microbiol Biotechnol. 2005;67(1):45–51.
- Castro C, Zuluaga R, Alvarez C, et al. Bacterial cellulose produced by a new acid-resistant strain of Gluconacetobacter genus. Carbohydr Polym. 2012;89(4):1033–1037.
- Mohite BV, Salunke BK, Patil SV. Enhanced production of bacterial cellulose by using Gluconacetobacter hansenii NCIM 2529 strain under shaking conditions. Appl Biochem Biotechnol. 2013;169(5):1497–1511.
- Qi GX, Luo MT, Huang C, et al. Comparison of bacterial cellulose production by Gluconacetobacter xylinus on bagasse acid and enzymatic hydrolysates. J Appl Polym Sci. 2017;134(28):45066–45072.
- Huang C, Yang XX, Guo L, et al. Using wastewater after lipid fermentation as substrate for bacterial cellulose production by Gluconacetobacter xylinus. Carbohydr Polym. 2016;136:198–202.
- Çakar F, Özer I, Aytekin AO, et al. Improvement production of bacterial cellulose by semi-continuous process in molasses medium. Carbohydr Polym. 2014;106:7–13.
- Pacheco G, Nogueira CR, Meneguin AB, et al. Development and characterisation of bacterial cellulose produced by cashew tree residues as alternative carbon source. Ind Crops Prod. 2017;107:13–19.
- Zhao H, Li J, Zhu K. Bacterial cellulose production from wastes products and fermentation conditions optimisation. Mat Sci Eng. 2018;394(2):1–6.
- Ruka DR, Simon GP, Dean KM. Altering the growth conditions of Gluconacetobacter xylinus to maximize the yield of bacterial cellulose. Carbohydr Polym. 2012;89(2):613–622.
- Krystynowicz A, Czaja W, Polomorski L, et al. Factors affecting the yield and properties of bacterial cellulose. J Ind Microbiol Biotechnol. 2002;29(4):189–195.
- Khattak WA, Khan T, Ul-Islam M, et al. Production, characterization and biological features of bacterial cellulose from scum obtained during preparation of sugarcane jaggery (gur). J Food Sci Technol. 2015;52(12):8343–8349.
- Aydın YA, Aksoy ND. Isolation and characterization of an efficient bacterial cellulose producer strain in agitated culture: gluconacetobacter hansenii P2A. Appl Microbiol Biotechnol. 2014;98(3):1065–1075.
- Son HJ, Kim HG, Kim KK, et al. Increased production of bacterial cellulose by Acetobacter sp. V6 in synthetic media under shaking culture conditions. Bioresour Technol. 2003;86(3):215–219.
- Costa AFS, Almeida FCG, Vinhas GM, et al. Production of bacterial cellulose by gluconacetobacter hansenii using corn steep liquor as nutrient sources. Front Microbiol. 2017;8:2027–2039.
- Jung HI, Jeong JH, Lee OM, et al. Influence of glycerol on production and structural–physical properties of cellulose from Acetobacter sp. V6 cultured in shake flasks. Bioresour Technol. 2010;101(10):3602–3608.
- Çoban EP, Biyik H. Evaluation of different pH and temperatures for bacterial cellulose production in HS (Hestrin-Scharmm) medium and beet molasses medium. Afr J Microbiol Res. 2011;5:1037–1045.
- Castro C, Zuluaga R, Putaux J-L, et al. Structural characterization of bacterial cellulose produced by Gluconacetobacter swingsii sp. from Colombian agroindustrial wastes. Carbohydr Polym. 2011;84(1):96–102.
- Mohite BV, Patil SV. Physical, structural, mechanical and thermal characterization of bacterial cellulose by G. hansenii NCIM 2529. Carbohydr Polym. 2014;106:132–141.
- Thorat MN, Dastager SG. High yield production of cellulose by a Komagataeibacter rhaeticus PG2 strain isolated from pomegranate as a new host. Royal Soc Chem Adv. 2018;8:29797–29805.
- Andritsou, de Melo ME, Tsouko E, et al. Synthesis and characterisation of bacterial cellulose from citrus-based sustainable resources. ACS Omega. 2018;3(8):10365–10373.
- Dayal MS, Goswami N, Sahai A, et al. Effect of media components on cell growth and bacterial cellulose production from acetobacter aceti MTCC 2623. Carbohydr Polym. 2013;94(1):12–16.
- Machado RTA, Gutierrez J, Tercjak A, et al. Komagataeibacter rhaeticus as an alternative bacteria for cellulose production Carbohydr Polym. 2016;152:841–849.
- Ul-Islam M, Khan T, Park JK. Water holding and release properties of bacterial cellulose obtained by in situ and ex situ modification. Carbohydr Polym. 2012;88(2):596–603.
- Zhong C, Zhang GC, Liu M, et al. Metabolic flux analyses of gluconacetobacter xylinus for bacterial cellulose production. Appl Microbiol Biotechnol. 2013;97(14):6189–6199.
- Ha JH, Shah N, Ul-Islam M, et al. Bacterial cellulose production from a single sugar α-linked glucuronic acid-based oligosaccharide. Process Biochem. 2011;46(9):1717–1723.
- Shezad O, Khan S, Khan T, et al. Physicochemical and mechanical characterization of bacterial cellulose produced with an excellent productivity in static conditions using a simple fed-batch cultivation strategy. Carbohydr Polym. 2010;82(1):173–180.
- Almeida IF, Pereira T, Silva NH, et al. Bacterial cellulose membranes as drug delivery systems: an in vivo skin compatibility study. European Journal of Pharmaceutics and Biopharmaceutics. 2014;86(3):332–336.