ABSTRACT
To investigate the compositional effects on thermal-diffusion kinetics in concentrated solid-solution alloys, interdiffusion in seven diffusion couples with alloys from binary to quinary is systematically studied. The alloys with higher compositional complexity exhibit in general lower diffusion coefficients against homologous temperature, however, an exception is found that diffusion in NiCoFeCrPd is faster than in NiCoFeCr and NiCoCr. While the derived diffusion parameters suggest that diffusion in medium and high entropy alloys is overall more retarded than in pure metals and binary alloys, they strongly depend on specific constituents. The comparative features are captured by computational thermodynamics approaches using a self-consistent database.
GRAPHICAL ABSTRACT
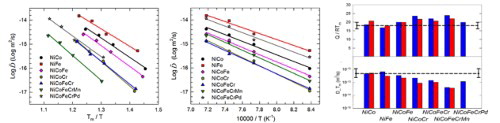
IMPACT STATEMENT
This work experimentally demonstrates that thermal-diffusion kinetics in concentrated solid-solution alloys depends not only on the number but also strongly on the species of the alloying elements.
The development of single-phase concentrated solid-solution alloys (SP-CSAs), including high entropy alloys (HEAs), in the past decade, has greatly broadened the scope of alloy design from focusing on the alloys containing one principle element to those containing multiple principle elements in near-equal concentration [Citation1–5]. The outstanding properties found in this new family of alloys, such as good thermal stability, high fracture toughness, good creep behavior, strong resistance to wear, oxidation and corrosion, and improved irradiation tolerance, have granted them promising application potential [Citation6–12]. Such excellent properties have been proposed correlated to four ‘core effects’, including high entropy, sluggish diffusion, severe lattice distortion, and cocktail effects [Citation6,Citation13]. Among them, the hypothesis that atomic diffusion is retarded in HEAs compared with in pure metals and traditional alloys has been considered crucial especially to the high-temperature behavior of HEAs, and has drawn extensive research interests [Citation14–16].
Although the concept of sluggish diffusion was proposed at the beginning of the HEA development [Citation2,Citation13], direct experimental evidence was not available until nearly a decade after [Citation14] and has still been limited till today [Citation6,Citation17]. Tsai et al. first compared the diffusion parameters in Ni–Co–Fe–Cr–Mn quinary alloys with pure metals and traditional alloys, and proposed a positive relation between the normalized activation energy and the number of alloying elements [Citation14]. This dataset has been re-analyzed by Beke and Erdélyi [Citation18] and Dabrowa et al. [Citation15] using different data analysis procedures, which both qualitatively supported the sluggish diffusion phenomenon. However, Miracle and Senkov re-plotted Tsai’s data with x-axis being absolute temperature, and argued that the diffusivity of NiCoFeCrMn0.5 alloy is not lower than the other reference materials at the same absolute temperatures [Citation6], which was supported by a tracer diffusion experiment by Vaidya et al. for Ni in equiatomic NiCoFeCr and NiCoFeCrMn [Citation16].
Existing diffusion experiments on investigating sluggish diffusion in HEAs have mainly focused on one or two diffusion systems. The target HEAs have been majorly NiCoFeCrMn (except one on NiCoFeCrAl with Al concentration below 10 at% [Citation15]), while the reference materials have been mostly pure metals and Fe–Cr–Ni (quasi-) ternary alloys from literatures. Increasing evidences have shown that specific alloying constituents can be more influential on the physical and mechanical properties compared with the number of alloying elements [Citation19–21]. Carefully checking the impact of various types of element is thus necessary before making final conclusions on the impact of the number of elements. Moreover, the absolute values of experimental diffusion coefficients are inevitably scattered from different sources, sometimes up to one order that is comparable to the extent of reported sluggish diffusion effects of HEAs [Citation6,Citation22]. To shed light on the controlling factors of sluggish diffusion, if exist, systematic experiments on a series of alloys containing various numbers and types of elements are highly desired, which have not been available.
In this study, a series of binary or pseudo-binary diffusion couples based on NiCo NiFe, NiCoFe, NiCoCr, NiCoFeCr, NiCoFeCrMn, and NiCoFeCrPd are designed and annealed at 920°C, 1020°C, 1070°C and 1120°C for 816, 192, 94.5, and 48 h, respectively, to systematically investigate the influence of the number and type of alloying elements on diffusion kinetics. The concentration profiles for all seven diffusion couples annealed at 1070°C are shown in Figure (a–g), as the representative cases for the other 3 temperatures. For convenient data analyses, only Ni and Co are designed to exhibit concentration gradient (except in Ni–NiFe), and the concentration fluctuation of the other non-target elements are small within experimental error and negligible. The details of alloy fabrication, couple design, and characterizations are in the Supplemental Materials.
Figure 1. (a–g) Concentration profiles of diffusion couples annealed at 1070°C; the solid lines are CALPHAD simulations; (h) backscattered electron image and EDX scan for (f).
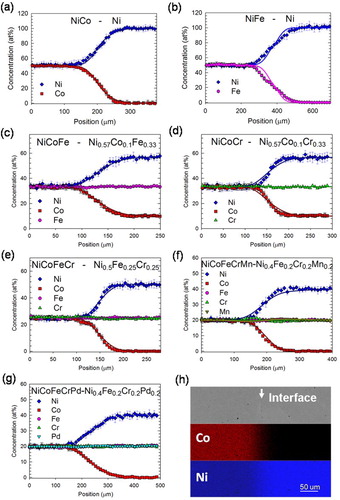
A typical scanning electron microscopy backscattered electron image and corresponding energy dispersive X-ray spectroscopy (EDX) mappings are shown in Figure (h). While a small amount of scattered oxides do form at the interface, all the diffusion interfaces are planar without noticeable Kirkendall porosity in the entire diffusion regime, similar to what seen in literatures for similar systems [Citation14,Citation23].
Computational thermodynamics (CALPHAD) simulations using a database based on literatures of binary and ternary alloys are performed to examine if the diffusion in HEAs can be understood or reasonably extrapolated from those containing less alloying elements. The simulation details can be found in Supplemental Materials. Here, we show the simulated concentration profiles at 1070°C in Figure (a–f) as solid lines as representative cases; their agreement with experiments serves as a complementary verification of the simulation database (no simulation on NiCoFeCrPd was performed due to the lack of validated database for Pd alloys).
The interdiffusion coefficients were derived using the Sauer–Freise (S–F) method [Citation24], which does not require a determined position of Matano interface, and has been widely applied for binary and quasi-binary interdiffusion couples [Citation14,Citation23]. Previous studies have shown that the S–F and another commonly used Boltzmann–Matano [Citation25,Citation26] methods derive consistent results for concentration-dependent diffusion coefficients [Citation23].
The interdiffusion coefficient from the S–F method can be expressed as
(1)
where C is the element concentration, for Ni in this study, C− and C+ are the concentrations at the infinite far ends from the interface, and C* is the concentration at a given position x* in the concentration profile, t is the diffusion time, and
. The cubic-spline fit was applied to evaluate the parameters in Equation (1).
The derived concentration-dependent interdiffusion coefficients are representatively shown in Figure for the case of NiCoCr couples. The values at the two ends of diffusion couples are intentionally omitted due to the inevitable artifacts by the numerical procedure of this method [Citation14,Citation23]. Mean interdiffusion coefficients for the range of concentration are estimated by definition [Citation23]:
(2)
Most literatures regarding the topic of sluggish diffusion compared the diffusion coefficients as a function of the homologous temperature (Tm/T, where Tm is the melting temperature), aiming to compensate the different melting temperature between alloys. Thus, we first show the correlation between
and Tm/T in Figure (a) for convenient comparison. At the same Tm/T, NiCoFeCrMn exhibits the lowest diffusivity among all the seven systems, which qualitatively agrees with its comparison with traditional alloys in literatures [Citation6]. In addition, the two binary alloys, with the lowest compositional complexity, exhibit the highest diffusion coefficients. These results may indicate a general trend of retarded diffusion with increasing compositional complexity. However, diffusion in quinary NiCoFeCrPd is shown faster than that in quaternary NiCoFeCr and even ternary NiCoCr. This observed exception suggests that diffusion does not necessarily become more sluggish with increasing number of alloying elements, but depends on specific constituents.
Ni tracer diffusivities from the CALPHAD simulation are displayed as solid lines in Figure (b) to compare with the experimental data. As discussed in Tsai et al. [Citation14], interdiffusion coefficients derived from the S–F method in NiCoFeCrMn can be approximated to the tracer diffusion coefficients, considering small Kirkendall effects and ideal solid solution [Citation27]. Detailed discussion on the accuracy of such approximation is beyond the scope of this work; here, we adapt such assumption and compare the results between all couples treated with consistent methods. The simulation results qualitatively agree well with the experiments: Ni diffuses the most slowly in NiCoFeCrMn, similarly in NiCoFeCr and NiCoCr, and fastest in other three systems at the same homologous temperatures. Relatively large quantitative discrepancies occur mainly in the Ni–Fe system, which has been observed in previous studies [Citation22] and does not affect the conclusions in the present study.
Figure 3. Diffusion coefficients as a function of homologous temperature. The lines in (a) are linear fits of experimental data; those in (b) are the Ni tracer diffusivities from CALPHAD simulations.
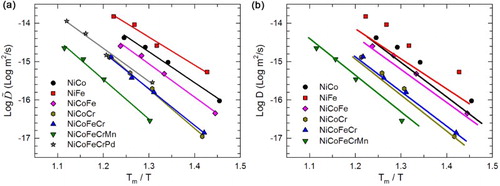
Good linear fittings in Figure (a) indicate that the diffusion coefficients in the studied alloys follow the Arrhenius equation:
(3)
where R is the gas constant. The activation energy Q and the frequency factor D0 are extracted and listed in Table . The activation energy does not necessarily increase with increasing number of alloying elements, especially that the value of NiCoFeCrPd is lower than all the other systems except for in NiFe. The value for NiCoFeCrMn is close to that for NiCoFeCr but lower than that for NiCoCr.
Table 1. Diffusion parameters for the alloys. The melting temperatures for NiCoFeCrPd are determined using differential scanning calorimeter measurements, and that for the others are from Wu et al. [Citation20].
It must be noted that the melting temperature of the two HEAs, NiCoFeCrMn, and NiCoFeCrPd are significantly lower than the others. The comparison against absolute temperatures, as shown in Figure , is thus necessary to have a more comprehensive picture. Apparently, sluggish diffusion of the two quinary alloys is no longer seen from this perspective: the diffusion rate of NiCoFeCrMn is greater than both NiCoFeCr and NiCoCr, while that of NiCoFeCrPd is even greater than all the other Ni–Co diffusion couples including the binary Ni–NiCo. The observation that the diffusion coefficient of NiCoFeCr is smaller than NiCoFeCrMn at the same absolute temperatures agrees with the results from a tracer diffusion experiments [Citation16].
The rationalization of plotting D over Tm/T may be traced back to an empirical variation form of Equation (3):
(4)
where K0 is a constant that depends on crystal structures, roughly 17 for FCC, and v is related to the bonding properties. Such form was first noticed in the 1930s [Citation28], and experimentally re-examined frequently over decades [Citation29,Citation30].
A constant diffusion coefficient at melting temperature, DTM, and a constant normalized activation energy Q/RTm have then been suggested for the alloys with the same structure and bonding type. To examine if our alloy system follows the same behavior, experimental and CALPHAD results of these two values are shown in Figure (a,b). The dash lines indicate the mean values of FCC metals and binary alloys summarized by Brown and Ashby [Citation30]. Our results for binary alloys are close to the mean values for both Q/RTm and DTM, and the alloys containing three or more elements seem to have slightly higher Q/RTm and lower DTM in general, which may support the proposed ‘sluggish diffusion’ concept [Citation14]. However, it needs to be noted that ternary NiCoCr has a similar Q/RTm value with NiCoFeCrMn, higher than NiCoFeCr and NiCoFeCrPd, suggesting that specific alloy constituents can play a significant role that overcome the effects of the number of elements. Furthermore, the differences among all the alloys are within one order for diffusivities and 30% for activation energies, which do not overpass the scatter range from the literature summary of the alloys with the same structure, and might not be considered very significant.
Figure 5. (a) Normalized activation energy and (b) melting temperature diffusivity of the tested alloys. The left bars are experimental data, and the right bars are CALPHAD results. The dash lines are the averaged values from Ref. [Citation30]. (c) Correlation between the pre-factor and the normalized activation energy; up triangle, round, and down triangle symbols are from the data in References [Citation14,Citation16,Citation22], respectively.
![Figure 5. (a) Normalized activation energy and (b) melting temperature diffusivity of the tested alloys. The left bars are experimental data, and the right bars are CALPHAD results. The dash lines are the averaged values from Ref. [Citation30]. (c) Correlation between the pre-factor and the normalized activation energy; up triangle, round, and down triangle symbols are from the data in References [Citation14,Citation16,Citation22], respectively.](/cms/asset/9803a8a0-b78c-413b-a0a2-43c28a1bd2b2/tmrl_a_1446466_f0005_c.jpg)
Similar to the case of diffusion coefficients, our CALPHAD calculations provide qualitatively the same comparison to the experiments in Figure (a,b). Note that the mobility database was obtained based on independent results of the alloys containing three or less elements. Thus, such a good agreement might suggest that the diffusion kinetics of HEAs are not qualitatively different from general CSAs containing lower number of elements.
Normalized activation energy is also scientifically meaningful for its correlation with the pre-factor. According to a thermodynamic treatment by Dienes [Citation31], D0 can be correlated to Q and Tm by
(5)
where ν is the Debye frequency, λ is the lattice parameter, and K is a constant. The correlation between Ln(D0) and Q/RTm is plot in Figure (c), which contains data from both this study and literatures for the subsystems of NiCoFeCrMn. In these alloys, the difference in lattice parameters of are within a few percent [Citation32]. In addition, although their Debye frequencies are not fully known, the available values for NiCoFe and NiCoCr are similar to that for the FCC Ni–Fe–Cr alloys and pure Ni [Citation33]. The discrepancies for both constants are thus considered small and treated as constants. A dashed line with a slope of 1 is drawn in Figure (c), and all data points fall approximately to this line as predicted by Equation (5), suggesting that the scheme of diffusion process may not be qualitatively changed with increasing number of elements.
The mechanisms of compositional effects on diffusion kinetics in concentrated alloys are complicated. The vacancy-mediated diffusion activation energy can be considered the sum of vacancy formation energy and migration barrier [Citation34]. Unlike pure metals, those defect energies are distributions rather than single values in concentrated alloys due to the unique atomic environments. First-principles calculations [Citation35] have revealed that, compared with pure Ni, NiCoCr and NiCoFeCr have lower migration barriers but higher vacancy formation energies. The physical origin has been proposed as the electronic charge deformation flexibility regarding eg to t2g state transition, while the accurately combined effects on diffusion activation energy remain unclear. It was also proposed that diffusion kinetics is correlated to the lattice potential energy fluctuation [Citation14], which might be affected by atomic size mismatch and electronic disorders [Citation9]. Addition of specific alloying elements, e.g. the 4-d element Pd, may modify the electronic structure [Citation19] and defect energy landscape of alloys, affect both formation and migration energies of vacancies, and eventually change the diffusion activation barriers and diffusivities. To further reveal the determinative compositional factors on diffusion kinetics, both interdiffusion and tracer diffusion experiments designed with specific variable controls on the number, type, and concentration of alloying elements are encouraged. Furthermore, theoretical studies from both first-principles calculations [Citation35] and CALPHAD modeling [Citation36] on this target are highly desired to understand the underlying physics.
In summary, interdiffusion in CSAs has been systematically studied with varying number and type of alloying elements. The results have shown that: (1) against homologous temperature, sluggish diffusion appears in general in the alloys with high compositional complexity, but an exception has been observed for NiCoFeCrPd, which exhibits higher diffusivity than NiCoCr and NiCoFeCr; (2) HEAs do not exhibit sluggish diffusion features against absolute temperature; and (3) the extrapolated diffusivities at melting temperatures of medium and HEAs are overall lower than the average values of pure metals and binary alloys, but the values also strongly depend on each specific constituent even with the same number of elements. CALPHAD calculations can qualitatively capture the above features using a database based on binary and ternary alloys, suggesting that the diffusion kinetics of HEAs may be reasonably extrapolated based on general CSAs containing less alloying elements.
Supplemental_materials_0222.docx
Download MS Word (49.5 KB)Acknowledgements
The authors acknowledge the insightful discussions with Drs Yanwen Zhang, Ying Yang, and Shijun Zhao.
Disclosure statement
No potential conflict of interest was reported by the authors.
ORCID
Hongbin Bei http://orcid.org/0000-0003-0283-7990
Additional information
Funding
References
- Cantor B, Chang ITH, Knight P, et al. Microstructural development in equiatomic multicomponent alloys. Mater Sci Eng A. 2004;375-377:213–218. doi: 10.1016/j.msea.2003.10.257
- Yeh JW, Chen SK, Lin SJ, et al. Nanostructured high-entropy alloys with multiple principal elements: novel alloy design concepts and outcomes. Adv Eng Mater. 2004;6(5):299–303. doi: 10.1002/adem.200300567
- Lucas MS, Wilks GB, Mauger L, et al. Absence of long-range chemical ordering in equimolar FeCoCrNi. Appl Phys Lett. 2012;100(25):251907. doi: 10.1063/1.4730327
- Senkov ON, Wilks GB, Scott JM, et al. Mechanical properties of Nb25Mo25Ta25W25 and V20Nb20Mo20Ta20W20 refractory high entropy alloys. Intermetallics. 2011;19(5):698–706. doi: 10.1016/j.intermet.2011.01.004
- Guo S, Liu CT. Phase stability in high entropy alloys: formation of solid-solution phase or amorphous phase. Prog Nat Sci. 2011;21(6):433–446. doi: 10.1016/S1002-0071(12)60080-X
- Miracle DB, Senkov ON. A critical review of high entropy alloys and related concepts. Acta Mater. 2017;122:448–511. doi: 10.1016/j.actamat.2016.08.081
- Zhang Y, Zuo T, Tang Z, et al. Microstructures and properties of high-entropy alloys. Prog Mater Sci. 2014;61:1–93. doi: 10.1016/j.pmatsci.2013.10.001
- Tsai M-H, Yeh J-W. High-entropy alloys: a critical review. Mater Res Lett. 2014;2(3):107–123. doi: 10.1080/21663831.2014.912690
- Zhang Y, Zhao S, Weber WJ, et al. Atomic-level heterogeneity and defect dynamics in concentrated solid-solution alloys. Curr Opin Solid State Mater Sci. 2017;21(5):221–237. doi: 10.1016/j.cossms.2017.02.002
- Gao MC, Zhang C, Gao P, et al. Thermodynamics of concentrated solid solution alloys. Curr Opin Solid St Mater Sci. 2017;21(5):238–251. doi: 10.1016/j.cossms.2017.08.001
- Zhang Y, Stocks GM, Jin K, et al. Influence of chemical disorder on energy dissipation and defect evolution in concentrated solid solution alloys. Nat Commun. 2015;6:1455.
- Lu C, Niu L, Chen N, et al. Enhancing radiation tolerance by controlling defect mobility and migration pathways in multicomponent single-phase alloys. Nat Commun. 2016;7:13564. doi: 10.1038/ncomms13564
- Yeh JW. Recent progress in high-entropy alloys. Ann Chim Sci Mat. 2006;31:633–648. doi: 10.3166/acsm.31.633-648
- Tsai KY, Tsai MH, Yeh JW. Sluggish diffusion in Co–Cr–Fe–Mn–Ni high-entropy alloys. Acta Mater. 2013;61(13):4887–4897. doi: 10.1016/j.actamat.2013.04.058
- Dąbrowa J, Kucza W, Cieślak G, et al. Interdiffusion in the FCC-structured Al-Co-Cr-Fe-Ni high entropy alloys: experimental studies and numerical simulations. J Alloy Comp. 2016;674:455–462. doi: 10.1016/j.jallcom.2016.03.046
- Vaidya M, Trubel S, Murty BS, et al. Ni tracer diffusion in CoCrFeNi and CoCrFeMnNi high entropy alloys. J Alloy Comp. 2016;688:994–1001. doi: 10.1016/j.jallcom.2016.07.239
- Zhang C, Zhang F, Jin K, et al. Understanding of the elemental diffusion behavior in concentrated solid solution alloys. J Phase Equilib Diffus. 2017;38(4):434–444. doi: 10.1007/s11669-017-0580-5
- Beke DL, Erdélyi G. On the diffusion in high-entropy alloys. Mater Lett. 2016;164:111–113. doi: 10.1016/j.matlet.2015.09.028
- Jin K, Sales BC, Stocks GM, et al. Tailoring the physical properties of Ni-based single-phase equiatomic alloys by modifying the chemical complexity. Sci Rep. 2016;6:213.
- Wu Z, Bei H, Pharr GM, et al. Temperature dependence of the mechanical properties of equiatomic solid solution alloys with face-centered cubic crystal structures. Acta Mater. 2014;81:428–441. doi: 10.1016/j.actamat.2014.08.026
- Gludovatz B, Hohenwarter A, Thurston KV, et al. Exceptional damage-tolerance of a medium-entropy alloy CrCoNi at cryogenic temperatures. Nat Commun. 2016;7:10602. doi: 10.1038/ncomms10602
- Million B, Růžičková J, Velíšek J, et al. Diffusion processes in the Fe-Ni system. Mater Sci Eng. 1981;50(1):43–52. doi: 10.1016/0025-5416(81)90084-7
- Karunaratne MSA, Reed RC. Interdiffusion of the platinum-group metals in nickel at elevated temperatures. Acta Mater. 2003;51(10):2905–2919. doi: 10.1016/S1359-6454(03)00105-8
- den Broeder FJA. A general simiplification and improvement of the Matano-Boltzmann method in the determination of the interdiffusion coefficients in binary systems. Scripta Metal. 1969;3:321–325. doi: 10.1016/0036-9748(69)90296-8
- Boltzmann L. Zur integration der diffusionsgleichung bei variabeln diffusionscoefficienten. Ann Phys. 1894;289:959. doi: 10.1002/andp.18942891315
- Matano C. On the relation between the diffusion-coefficients and concentrations of solid metals (the nickel-copper system). Jap J Phys. 1933;8(3):109–113.
- Otto F, Yang Y, Bei H, et al. Relative effects of enthalpy and entropy on the phase stability of equiatomic high-entropy alloys. Acta Mater. 2013;61(7):2628–2638. doi: 10.1016/j.actamat.2013.01.042
- Liempt J. Die Berechnung der Auflockerungswärme der Metalle aus Rekristallisationsdaten. Z Physik. 1935;96:534–541. doi: 10.1007/BF01337708
- Sherby OD, Simnad MT. Prediction of atomic mobility in metallic systems. Trans A S M. 1961;54:227–240.
- Brown AM, Ashby MF. Correlations for diffusion constants. Acta Metal. 1980;28(8):1085–1101. doi: 10.1016/0001-6160(80)90092-9
- Dienes GJ. Frequency factor and activation energy for the volume diffusion of metals. J Appl Phys. 1950;21(11):1189–1192. doi: 10.1063/1.1699563
- Jin K, Mu S, An K, et al. Thermophysical properties of Ni-containing single-phase concentrated solid solution alloys. Mater Des. 2017;117(5):185–192. doi: 10.1016/j.matdes.2016.12.079
- Jin K, Gao YF, Bei H. Intrinsic properties and strengthening mechanism of monocrystalline Ni-containing ternary concentrated solid solutions. Mater Sci Eng A. 2017;695:74–79. doi: 10.1016/j.msea.2017.04.003
- Janotti A, Krčmar M, Fu CL, et al. Solute diffusion in metals: larger atoms can move faster. Phys Rev Lett. 2004;92(8):085901. doi: 10.1103/PhysRevLett.92.085901
- Zhao S, Egami T, Stocks GM, et al. Effect of d electrons on defect properties in equiatomic NiCoCr and NiCoFeCr concentrated solid solution alloys. Phys Rev Mater. 2018;2(1):013602. doi: 10.1103/PhysRevMaterials.2.013602
- Kattner UR, Campbell CE. Invited review: modelling of thermodynamics and diffusion in multicomponent systems. Mater Sci Technol. 2009;25(4):443–459. doi: 10.1179/174328408X372001