ABSTRACT
We report the synthesis of eight new members of the i-MAX family, of the formula (Mo2/3RE1/3)2GaC, where RE = Gd, Tb, Dy, Ho, Er, Tm, Lu, and Yb, the latter not previously incorporated in a MAX phase. The structure and composition of powder samples were investigated by X-ray diffraction, scanning transmission electron microscopy, and energy dispersive X-ray analysis combined with scanning electron microscopy. All phases showed evidence of an orthorhombic (Cmcm) structure, and the phases based on Er and Yb also crystallized in a monoclinic (C2/c) arrangement. The chemical order of the magnetic elements suggests interesting magnetic characteristics, with a high tuning potential through the range of attainable lanthanide elements.
GRAPHICAL ABSTRACT
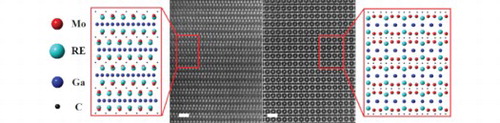
IMPACT STATEMENT
We present eight new in-plane ordered i-MAX phases based on rare earth elements, including Yb (new MAX phase element), with high magnetic characteristics tuning potential.
Introduction
Chemical ordering of magnetic elements in a material sits at the crossroad of fascinating condensed matter physics, giving rise to phenomena such as the anomalous Hall effect and multiferroicity [Citation1,Citation2]. Furthermore, rare earth (RE) elements are known to exhibit various magnetic and electronic ground states, owing to hybridization of 4f and conduction electrons [Citation3–5]. Heavy fermion systems being complex are excellent systems to study interactions between magnetic and valence instabilities [Citation6]. These are also useful systems to study quantum phase transitions [Citation7] and complex collective quantum states [Citation8]. When the ions are surrounded by the free electrons, the interaction among the f shells is dominated by indirect exchange coupling between the localized spins, the Ruderman–Kittel–Kasuya–Yosida (RKKY) interaction [Citation9–11], giving rise to long-range magnetic order. Heavy fermion states in some lanthanides can lead to interesting magnetic states, including metamagnetic-like transitions [Citation5,Citation8,Citation12].
Recently, a family of quaternary atomic laminates was discovered, coined i-MAX phases [Citation13,Citation14]. They stem from so called MAX phases, which are a family of atomic nanolaminates with general formula Mn+1AXn, where M is a transition metal, A is an A-group element and X is C or N, n = 1–3 [Citation15]. First discovered in the 1960s [Citation16] and further extensively studied in the 1990s [Citation17] and onwards, MAX phases have been attractive study topic due to their combination of metallic and ceramic properties [Citation18] with remarkable mechanical properties [Citation19]. These properties make the materials suitable for various applications, such as nuclear reactor applications [Citation20], ohmic contacts [Citation21], and precursors for the synthesis of their 2D counterparts—MXenes [Citation22,Citation23].
Different to MAX phases, the i-MAX phases display in-plane chemical ordering of two different M-elements, as described by the general formula (M12/3M21/3)2AC, wherein the M1 atoms form a honeycomb lattice and the M2 atoms a triangular lattice. Furthermore, the A-layer is arranged in a Kagomé-like structure. For a more detailed description, see Refs. [Citation14,Citation24]. To date, i-MAX phases with A = Al and Ga have been reported, where X is C. Notably, the discovery of i-MAX phases has significantly expanded the number of M elements used in MAX-phases, for example through M = Y and W [Citation25,Citation26]. Furthermore, the i-MAX phases have been shown to realize a new type of MXenes with either in-plane chemical order or vacancy-ordering [Citation13,Citation25,Citation27], with shown high potential for energy storage.
Most recently, the discovery of 11 new i-MAX phases was reported, including rare-earth (RE) elements and being of the general formula (Mo2/3RE1/3)2AlC [Citation28], where RE = Ce, Pr, Nd, Sm, Gd, Tb, Dy, Ho, Er, Tm, and Lu. It was shown that these phases can display three different but similar symmetries; monoclinic (C2/c), monoclinic (C2/m), and orthorombic (Cmcm). Furthermore, analysis of RE-i-MAX phases with Nd, Gd, Tb, and Er indicated complex magnetic ordering, being sensitive to temperature and applied a magnetic field, which is characteristics of frustrated systems. This recent study has served as a motivation for the present work, and herein we report the synthesis and structural characterization of eight new i-MAX compounds of the general formula (Mo2/3RE1/3)2GaC, for which RE = Gd, Tb, Dy, Ho, Er, Tm, Yb, and Lu. The realized phases add a new element to the MAX phase family (Yb), and serve as inspiration for continued systematic studies on the effect of RE element on the magnetic properties. The compounds also allow detailed studies on the effect of A-element on the overall magnetic characteristics.
Methods
Materials synthesis
Polycrystalline samples of (Mo2/3RE1/3)2GaC, where RE = Gd, Tb, Dy, Ho, Er, Tm, Yb, and Lu, were synthesized by solid–state reaction from elemental constituents. Elemental powders of Mo (99.99%) and graphite (99.999%), from Sigma Aldrich, were used together with the RE elements (99.5%), the latter from Stanford Advanced Materials. For Ga, metal pellets (99.99999%), from Mining and Chemical Products Limited, of about 7 mm diameter and of mass ∼1 g were used, after being mechanically cleaved into smaller pieces of about 1 mm in diameter. Mo:RE:Ga:C were mixed in a stoichiometric ratio of 4:2:3:3. First, Mo, RE and C powders were mechanically mixed in an agate mortar. The powder mixture was then placed in an alumina crucible, Ga pellets were added, and the pellet/powder mixture was stirred. The alumina crucible with its content was thereafter heated under five standard cubic centimeter per minute (sccm) Ar flow to 1400°C at a rate of 10°C/min and then held at 1400°C for 5 h. The loosely sintered powders were crushed into a fine powder that was directly used for further analysis.
Structural characterization
X-ray diffraction (XRD) analysis of powder samples was performed using a Panalytical X’pert powder diffractometer using Cu Kα radiation (λ = 1.54 Å), equipped with Bragg–Brentano HD on the incident side with 1/4° divergence slit and 1/2° anti-scatter slit. On the diffracted beam side, a 5-mm anti-scatter slit together with a Soller slit (with an opening radius of 0.04) was used. A continuous scan from 5° to 120° was performed on the sample with a step size of 0.008° and counting time of 60 s per step. XRD Rietveld refinement using FullProf Suite [Citation29,Citation30] was used to evaluate the sample’s phase purity.
Specimens for scanning transmission electron microscopy (STEM) analysis were prepared by dispersing powder on Cu grid with C film. STEM combined with high angle annular dark field imaging (STEM-HAADF) and selected area electron diffraction (SAED) was performed in the double-corrected Linköping FEI Titan3, operated at 300 kV. Scanning electron microscopy (SEM) analysis was performed using an LEO 1550 SEM operated at 20 kV accelerating voltage, combined with energy dispersive X-ray spectroscopy (EDS) analysis with an Oxford Instruments X-Max 80 mm2 SDD detector.
Results and discussion
All of the synthesized RE i-MAX phases were found to crystallize in a structure of orthorhombic (Cmcm) symmetry, however presence of monoclinic (C2/c) phase was also found in the Er and Yb samples. For details of these structures, see Figure S1 in the supplementary information. The (Mo2/3Tm1/3)2GaC i-MAX phase was found to be one of the highest purity and was thus chosen as a model system for space group #63 (Cmcm), shown in Figure . There, STEM images of the i-MAX phase along the (a) [100] and (b) [010] zone axes are shown along with schematic atomic arrangements of the structure based on an orthorombic structure of space group Cmcm. The Tm atoms, having the highest atomic number, are the brightest ones due to the Z-contrast in STEM, with Ga and Mo appearing less bright (C is not visible). The view of the i-MAX along the [100] zone axis is identical to the classical MAX phase when viewed along the [Citation11–20] zone axis. Along the [010], the chemical ordering characteristic of the i-MAX phase is evident, where the Tm atoms extend from the Mo layer towards the Ga layer. The Ga layer is arranged in a Kagomé structure, concluded from the alternating contrast between neighboring Ga atoms in the [010] orientation, as seen in Figure (b). The SEM-EDX analysis of the (Mo2/3Tm1/3)2GaC sample gives the relative composition of 44, 22 and 34 at% for Mo, Tm and Ga, respectively. The XRD data together with Rietveld refinement is shown in Figure (c), and it supports the orthorhombic symmetry (Cmcm) as determined from STEM and SAED. The goodness of fit (χ2) and RF-factor for the i-MAX phase values are 22.1 and 7.7, respectively. The goodness of fit and RF-factors for all other samples can be found in Table S1 in the supplementary information. The quantitative analysis of the sample shows that i-MAX is the main phase, with a small amount of Mo2C, Tm2O3, and TmGa3 impurities. The RE oxide is present in all samples of the present paper, likely originating from a reaction between the alumina crucible and the constituent powder at the sintering temperature. The summary and estimated phase composition of all i-MAX containing samples can be found in Table , except for the sample for RE = Gd (due to low sample purity). Attempts were also made to synthesize also (Mo2/3RE1/3)2GaC i-MAX phase where RE = La, Ce, Pr, Nd, and Sm, however, no i-MAX phase could be identified for these elements.
Figure 1. STEM micrographs of (Mo2/3Tm1/3)2GaC i-MAX phase along (a) [100] and (b) [010] zone axis. Insets in panels of (a) and (b) show SAED from respective orientation. Schematics next to each panel represent the corresponding atomic arrangements assuming a structure with orthorhombic symmetry (Cmcm). The scale bars in (a) and (b) correspond to 1 nm. (c) XRD pattern and Rietveld refinement (calculated) of (Mo2/3Tm1/3)2GaC, including identified impurity phases.
![Figure 1. STEM micrographs of (Mo2/3Tm1/3)2GaC i-MAX phase along (a) [100] and (b) [010] zone axis. Insets in panels of (a) and (b) show SAED from respective orientation. Schematics next to each panel represent the corresponding atomic arrangements assuming a structure with orthorhombic symmetry (Cmcm). The scale bars in (a) and (b) correspond to 1 nm. (c) XRD pattern and Rietveld refinement (calculated) of (Mo2/3Tm1/3)2GaC, including identified impurity phases.](/cms/asset/4fd4d7f8-0d6d-423a-8812-0406221bd36f/tmrl_a_1644684_f0001_oc.jpg)
Table 1. Phase composition of different samples as quantitatively estimated from Rietveld refinement.
Table shows the lattice parameters of the i-MAX phases determined from Rietveld refinement, see XRD patterns and, where possible, also the refined data in Figure S2. A clear trend emerges, where all lattice parameters, a, b, and c decrease with the increasing atomic number going from Gd to Lu, with (Mo2/3Gd1/3)2GaC having the largest unit cell and (Mo2/3Lu1/3)2GaC the smallest one out of the materials presented herein. The structural parameters were determined based on a (Cmcm) structure.
Figure shows STEM micrographs of (Mo2/3Yb1/3)2GaC in the (C2/c) structure. This atomic arrangement was also found in the sample where RE = Er, though only as a minority phase. Both the (Cmcm) and the (C2/c) structures consist of alternating layers of (Mo2/3RE1/3)2C and Ga building blocks where (Mo2/3RE1/3)2C can have three different orientations; A, B (rotated +60° relative A), and C (rotated +180° relative to A). Different stacking sequences of (Mo2/3RE1/3)2C result in a (C2/c) structure with ABAB stacking, and a (Cmcm) structure with ACAC stacking. Nonetheless, also Figure shows the characteristic i-MAX features of both the M-element ordering and the indicated rearrangement of the A-layer.
Figure 2. STEM micrographs of (Mo2/3Yb1/3)2GaC sample along the (a) [100], and in (b) [110] zone axis, showing the monoclinic space group #15 structure (C2/c). Schematics next to each panel represent the corresponding atomic arrangements assuming a structure with monoclinic symmetry (C2/c). The scale bars in (a) and (b) are 1 nm.
![Figure 2. STEM micrographs of (Mo2/3Yb1/3)2GaC sample along the (a) [100], and in (b) [110] zone axis, showing the monoclinic space group #15 structure (C2/c). Schematics next to each panel represent the corresponding atomic arrangements assuming a structure with monoclinic symmetry (C2/c). The scale bars in (a) and (b) are 1 nm.](/cms/asset/88dfaa81-e30a-4557-b6fa-40b4ddddf4cd/tmrl_a_1644684_f0002_oc.jpg)
Figure shows the STEM micrographs of all synthesized (Mo2/3RE1/3)2GaC i-MAX phases in the present study, with the chemical order of the i-MAX being evident when viewed along the [010] or [110] zone axis. Both (C2/c) and (Cmcm) symmetries are identified. Further STEM and SAED analyses are presented in Figures S3–S9. Furthermore, the relative composition of the phases, as obtained by SEM-EDX, can be found in Table S2 in the supplemental information. Comparing the structures of all RE-based i-MAX phases identified to date, it can be noted that a majority of those with A = Al are monoclinic, while those with A = Ga are primarily orthorhombic. The origin thereof is not clear, even though we find 3.5% higher values of b and c lattice parameters of the Al-based phases, compared to the Ga counterparts, while a lattice parameters are larger for the Ga-based phases. The resulting difference in volume is about 1%, with larger values for the Al-based phases, consistent with the larger radius of Al compared to Ga.
Figure 3. STEM micrographs of (Mo2/3RE1/3)2GaC i-MAX phases showing the characteristic chemical order, as described primarily by a space group #63 (Cmcm) symmetry. The samples (a) RE = Gd and (b) RE = Tb are viewed along the [110] zone axis, showing a zig-zag-like pattern along with the chemical order. The samples (c) RE = Dy, (d) RE = Ho, (f) RE = Tm and (h) RE = Lu are viewed along the [010] zone axis. In (e) and (g), for the Er and Yb samples, respectively, both #63 (Cmcm) and #15 (C2/c) symmetries are present in a single grain (segments marked with arrows), thus this orientation corresponds to the [010] zone axis for (Cmcm) and the [110] zone axis for (C2/c). The scale bars in (a)–(h) are 1 nm.
![Figure 3. STEM micrographs of (Mo2/3RE1/3)2GaC i-MAX phases showing the characteristic chemical order, as described primarily by a space group #63 (Cmcm) symmetry. The samples (a) RE = Gd and (b) RE = Tb are viewed along the [110] zone axis, showing a zig-zag-like pattern along with the chemical order. The samples (c) RE = Dy, (d) RE = Ho, (f) RE = Tm and (h) RE = Lu are viewed along the [010] zone axis. In (e) and (g), for the Er and Yb samples, respectively, both #63 (Cmcm) and #15 (C2/c) symmetries are present in a single grain (segments marked with arrows), thus this orientation corresponds to the [010] zone axis for (Cmcm) and the [110] zone axis for (C2/c). The scale bars in (a)–(h) are 1 nm.](/cms/asset/148c781c-946e-4ef1-b7e4-8bdda5bedc21/tmrl_a_1644684_f0003_ob.jpg)
It is also noted that there are no Ga-based i-MAX phases with light RE-elements, as opposed to the Al-based counterpart that can accommodate both light and heavy RE-elements. The reason for this observation can likely not be explained simply by elemental characteristics or preferred crystal structure, though it should be noted that the light RE elements of the Al phases crystallize in a monoclinic C2/m structure, whereas the heavier ones form the monoclinic C2/c structure. The former structure has not been identified for the here presented Ga-based phases.
Since the only difference between these eight new i-MAX phases is the change in RE element, our discovery allows systematic, fundamental studies of the effect of the RE element on materials properties. Furthermore, the presence of RE elements with 4f electrons might provide interesting magnetic properties, in line with the plethora of magnetic characteristics found in previous RE-containing i-MAX phases [Citation28], including magnetically frustrated systems due to inter- and intralayer interaction. Most of the RE-i-MAX phases reported herein, with A = Ga, have a counterpart in the previously reported corresponding compound (Mo2/3RE1/3)2AlC, with A = Al. Thus, the tuning potential of these materials is facilitated by both changes in the RE elements and the A layer. The latter is exemplified for MAX phases through previously observed altered magnetic transition temperature when going from (Cr1−xMnx)2GaC [Citation31] to (Cr1−xMnx)2AlC [Citation32]. Similar effects might be expected for the magnetic i-MAX phases. Furthermore, Ga is known to enhance the magnetostrictive properties of Fe [Citation33], and also significant magnetostriction was found for the Mn2GaC MAX phase [Citation34]. Similar investigations of magnetic properties of the novel Ga-based materials of the present paper are therefore suggested.
Finally, Yb has previously been used in attempts to synthesize (Mo2/3Yb1/3)2AlC [Citation28], though not successful to date. The suggested explanation included the divalent nature of the Yb. Through the here presented synthesis of (Mo2/3Yb1/3)2GaC i-MAX phase, Yb is a new M-element in the MAX phase family. The mechanism behind the formation of a Yb-based i-MAX phase for A = Ga, but not for A = Al, remains to be investigated, however, the interaction resulting from the divalent characteristics may be at least part of the explanation. This would suggest that also (Mo2/3Eu1/3)2GaC exists, which was, however, not attempted in the present study.
Conclusions
Eight new i-MAX phases were realized based on RE as minority M element, of the formula (Mo2/3RE1/3)2GaC for RE = Gd, Tb, Dy, Ho, Er, Tm, Yb, and Lu. The compounds were found to crystallize in an orthorombic (Cmcm) structure, with the exception for RE = Ho and Yb, where also (C2/c) was found present in a small amount. The lattice parameters trendwise decrease by increasing atomic number going from Gd to Lu.
Acknowledgements
A. P. acknowledges Quanzheng Tao for provided help and support in Rietveld refinement.
Disclosure statement
No potential conflict of interest was reported by the authors.
Additional information
Funding
References
- Nakatsuji S, Kiyohara N, Higo T. Large anomalous Hall effect in a non-collinear antiferromagnet at room temperature. Nature. 2015;527:212–215. doi: 10.1038/nature15723
- Essafi K, Benton O, Jaubert LDC. A kagome map of spin liquids from XXZ to Dzyaloshinskii–Moriya ferromagnet. Nat Commun. 2016;7:10297. doi: 10.1038/ncomms10297
- Riseborough PS. Heavy fermion semiconductors. Adv Phys. 2000;49(3):257–320. doi: 10.1080/000187300243345
- Dzero M, Xia J, Galitski V, et al. Topological Kondo insulators. Annu Rev Condens Matter Phys. 2016;7(1):249–280. doi: 10.1146/annurev-conmatphys-031214-014749
- Xu Y, Yue C, Weng H, et al. Heavy Weyl fermion state in CeRu4Sn6. Phys Rev X. 2017;7(1):011027. doi: 10.1103/PhysRevX.7.011027
- Flouquet J, Harima H. 2009. Heavy fermion material: Ce versus Yb case. https://arxiv.org/abs/0910.3110
- Brando M, Belitz D, Grosche FM, et al. Metallic quantum ferromagnets. Rev Mod Phys. 2016;88(2):025006. doi: 10.1103/RevModPhys.88.025006
- Wirth S, Steglich F. Exploring heavy fermions from macroscopic to microscopic length scales. Nat Rev Mater. 2016;1:16051. doi: 10.1038/natrevmats.2016.51
- Ruderman MA, Kittel C. Indirect exchange coupling of nuclear magnetic moments by conduction electrons. Phys Rev. 1954;96(1):99–102. doi: 10.1103/PhysRev.96.99
- Kasuya T. A theory of metallic ferro- and antiferromagnetism on Zener’s model. Prog Theor Phys. 1956;16(1):45–57. doi: 10.1143/PTP.16.45
- Yosida K. Magnetic properties of Cu-Mn alloys. Phys Rev. 1957;106(5):893–898. doi: 10.1103/PhysRev.106.893
- Gertrud Z. Field-induced suppression of the heavy-fermion state in YbRh2Si2. J Phys: Condens Matter. 2011;23(9):094215.
- Tao Q, Dahlqvist M, Lu J, et al. Two-dimensional Mo1.33C MXene with divacancy ordering prepared from parent 3D laminate with in-plane chemical ordering. Nat Commun. 2017;8:14949. doi: 10.1038/ncomms14949
- Dahlqvist M, Lu J, Meshkian R, et al. Prediction and synthesis of a family of atomic laminate phases with Kagomé-like and in-plane chemical ordering. Sci Adv. 2017;3(7):e1700642. doi: 10.1126/sciadv.1700642
- Barsoum MW. MAX phases: properties of machinable ternary carbides and nitrides. 2013.
- Jeitschko W, Nowotny H, Benesovsky F. Kohlenstoffhaltige ternäre Verbindungen (V-Ge-C, Nb-Ga-C, Ta-Ga-C, Ta-Ge-C, Cr-Ga-C und Cr-Ge-C). Monatsh Chem. 1963;94(5):844–850. doi: 10.1007/bf00902358
- Barsoum MW. The MN+1AXN phases: a new class of solids: thermodynamically stable nanolaminates. Prog Solid State Chem. 2000;28(1–4):201–281. doi: 10.1016/S0079-6786(00)00006-6
- Barsoum MW, El-Raghy T. The MAX phases: unique new carbide and nitride materials: ternary ceramics turn out to be surprisingly soft and machinable, yet also heat-tolerant, strong and lightweight. Am Sci. 2001;89(4):334–343. doi: 10.1511/2001.28.736
- Barsoum MW, Radovic M. Elastic and mechanical properties of the MAX phases. Annu Rev Mater Res. 2011;41(1):195–227. doi: 10.1146/annurev-matsci-062910-100448
- Hoffman EN, Vinson DW, Sindelar RL, et al. MAX phase carbides and nitrides: properties for future nuclear power plant in-core applications and neutron transmutation analysis. Nucl Eng Des. 2012;244(Supplement C):17–24. doi: 10.1016/j.nucengdes.2011.12.009
- Fashandi H, Dahlqvist M, Lu J, et al. Synthesis of Ti3AuC2, Ti3Au2C2 and Ti3IrC2 by noble metal substitution reaction in Ti3SiC2 for high-temperature-stable Ohmic contacts to SiC. Nat Mater. 2017;16:814–818. doi: 10.1038/nmat4896
- Naguib M, Mashtalir O, Carle J, et al. Two-dimensional transition metal carbides. ACS Nano. 2012;6(2):1322–1331. doi: 10.1021/nn204153h
- Anasori B, Lukatskaya MR, Gogotsi Y. 2D metal carbides and nitrides (MXenes) for energy storage. Nat Rev Mater. 2017;2:16098. doi: 10.1038/natrevmats.2016.98
- Dahlqvist M, Petruhins A, Lu J, et al. Origin of chemically ordered atomic laminates (i-MAX): expanding the elemental space by a theoretical/experimental approach. ACS Nano. 2018;12(8):7761–7770. doi: 10.1021/acsnano.8b01774
- Meshkian R, Dahlqvist M, Lu J, et al. W-based atomic laminates and their 2D derivative W1.33C MXene with vacancy ordering. Adv Mater. 2018;30(21):1706409. doi: 10.1002/adma.201706409
- Lu J, Thore A, Meshkian R, et al. Theoretical and experimental exploration of a novel in-plane chemically ordered (Cr2/3M1/3)2AlC i-MAX phase with M=Sc and Y. Cryst Growth Des. 2017;17(11):5704–5711. doi: 10.1021/acs.cgd.7b00642
- Persson I, el Ghazaly A, Tao Q, et al. Tailoring structure, composition, and energy storage properties of MXenes from selective etching of in-plane, chemically ordered MAX phases. Small. 2018;14(17):1703676. doi: 10.1002/smll.201703676
- Tao Q, Lu J, Dahlqvist M, et al. Atomically layered and ordered rare-earth i-MAX phases: a new class of magnetic quaternary compounds. Chem Mater. 2019;31(7):2476–2485. doi: 10.1021/acs.chemmater.8b05298
- Rodríguez-Carvajal J. Recent advances in magnetic structure determination by neutron powder diffraction. Phys B: Condens Matter. 1993;192(1):55–69. doi: 10.1016/0921-4526(93)90108-I
- Rodríguez-Carvajal J. Recent developments of the program FULLPROF. Commission on powder diffraction (IUCr). Newsletter. 2001;26:12–19.
- Salikhov R, Semisalova AS, Petruhins A, et al. Magnetic anisotropy in the (Cr0.5Mn0.5)2GaC MAX phase. Mater Res Lett. 2015;3(3):156–160. doi: 10.1080/21663831.2015.1036324
- Mockute A, Persson POÅ, Magnus F, et al. Synthesis and characterization of arc deposited magnetic (Cr,Mn)2AlC MAX phase films. Phys Status Solidi RRL. 2014;8(5):420–423. doi: 10.1002/pssr.201409087
- Atulasimha J, Flatau AB. A review of magnetostrictive iron–gallium alloys. Smart Mater Struct. 2011;20(4):043001. doi: 10.1088/0964-1726/20/4/043001
- Novoselova IP, Petruhins A, Wiedwald U, et al. Large uniaxial magnetostriction with sign inversion at the first order phase transition in the nanolaminated Mn2GaC MAX phase. Sci Rep. 2018;8(1):2637. doi: 10.1038/s41598-018-20903-2