Abstract
Triboelectric nanogenerator (TENG) is a new type of device that can convert mechanical energy in the environment into electrical energy. Since its wide range of energy sources, high energy conversion efficiency and wide application range, it has become a hot spot in the energy field. However, the shortcomings of the high internal resistance, the low output current and power density have seriously affected the further applications of TENGs. This review is aimed to introduce the recent advanced methods and applications of controlling the interface contact electrification of TENGs, and identify and discuss the key issues that deserve additional studies.
GRAPHICAL ABSTRACT
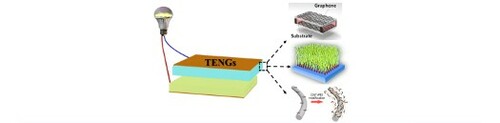
IMPACT STATEMENT
This paper delineates the advanced methods and applications of enhancing the electrical output of TENG and looks forward to the application prospects of TENG in other fields.
1. Introduction
Triboelectricity is also called contact electrification, which means that when two different substances come into contact, the charge will move from one side to the other [Citation1–4]. Since its inherent characteristics of causing extremely high voltages, it is considered a negative effect, causing accidents or discomfort. In daily life activities, triboelectricity will induce a voltage as high as 35,000 V [Citation5–7], and the electric sparks generated by electrostatic discharge will cause unpredictable losses in the industry, medical industry and electronics industry [Citation8–10]. Researchers have developed related technologies to eliminate triboelectricity, which can be summarized as three methods: reducing the generation of triboelectric charge, increasing the dissipation of triboelectric charge and the neutralization method. On the other hand, the technology of using static electricity has also produced many meaningful applications, such as electrospinning, electrostatic printing and triboelectric nanogenerators (TENGs) [Citation11–14].
By changing the way of thinking, TENGs invented by Zhonglin (ZL) Wang and others can use triboelectricity for energy harvesting [Citation15]. The TENG is a device that can use frictional energy to collect and convert static electricity generated by friction into electric energy [Citation16–20]. It can be used as an energy source, or combining this part of the electric current with microelectronics to produce miniature electronic systems promote the miniaturization and portability of electronic technology. As a device for collecting triboelectricity, the TENG's output largely depends on the number of electrostatic charges generated by triboelectricity [Citation20–22]. When two different materials come into contact due to external mechanical energy, the frictional electrification on the surface of each material will generate static charges. If the additional mechanical energy to separate the materials has been considered, a potential will be generated between the static charges, and the charge on the conductive material will move from one side to the other. It’s worth noting that the charge transfer between them is not immediately reversible, which means that the excess charge on one side is left behind, while the other side has insufficient charge [Citation23–27]. However, TENG's low current characteristics make it unsuitable for powering storage devices. In addition to the advantages of high output power of TENG, TENG also has advantages in selecting feasible materials, because frictional charging occurs in almost all material combinations: solid–solid, solid–liquid, solid–gas and liquid–gas [Citation28–32]. Thanks to these efforts and the aforementioned benefits, TENG has proven its potential to provide higher output power in the coming era of the Internet of Things. On the other hand, the current feasible application areas of TENGs are also rapidly expanding. In addition to wearable devices, implantable TENG is also being studied as a human-machine interface device. In addition, according to recent research, other mechanical energy sources, such as sound and magnetic fields, can also be harvested. Judging from these research results, the commercialization of TENG is likely to be completed in the near future [Citation33–36].
The main problem that TENGs face in the actual application process is low power density, which is determined by the characteristics of TENGs themselves [Citation37–39]. The reasons which TENGs can continuously supply power to the equipment is due to the storage of triboelectric charges and the generation of induced charges. However, since the moment the triboelectric charge is generated, it has been continuously dissipated into the environment, which reduces the amount of triboelectric charge stored on the surface of the tribolayers [Citation40]. As a result, the number of induced charges on the back electrode is reduced, which ultimately affects the electrical output. According to reports, the surface triboelectric charge of the PDMS film with a surface potential of 40 V after contact electrification will dissipate to the initial state within 2 min [Citation41]. It is a very serious problem, which determines whether TENGs can be used as a stable power supply module for equipment. On the other hand, the small capacitance of the tribolayer materials will also reduce the storage of triboelectric charges on the surface. Therefore, how to improve the contact electrification and storage performances of the tribolayers is very worthy of discussion.
In this review, as illustrated in Figure , the focus is primarily on recent advances in the methods of increasing electrical output of TENGs, including surface engineering, functional group modification or compounding of extreme electrode materials to improve the ability of tribolayer to gain/loss electrons, and improving the storage performance through chemical modification, the doping of dielectric materials or the introduction of storage intermediate-layer, then regulate the contact electrification of the friction interface. Subsequently, the applications of TENGs in energy harvesting, sensors, medicine, industry and other fields are introduced. Coinciding with the global pandemic of COVID-19, we look forward to the application of TENGs in flexible wearable devices, especially the potential application value of sensors such as body temperature and blood oxygen.
2. Influencing factors of interface contact electrification
2.1. Triboelectric polarity
There are many kinds of materials used as tribolayers, including polymers, metals and inorganic materials. The most commonly used materials are dielectric polymers such as PTFE, FEP, PDMS and Kapton. Green materials (such as cellulose-based materials) have recently received more and more attention, and the performance of TENG using cellulose materials has also been improved [Citation42–46]. The choice of material is very important for TENG, because the triboelectric effect of the material is the basis of TENG. To design TENG for a specific application, several parameters need to be considered, such as power density, stability, flexibility and sustainability [Citation47–51]. Since the triboelectric polarity of each material is determined, so, it is very important to choose the right tribolayer materials. It is precise because the triboelectric polarity of each material is inherent, it can be decided to choose the appropriate materials to assemble TENG [Citation52–56]. According to related reports, the triboelectric sequence of typical materials is shown in Figure . It can be seen from the figure that fluoropolymers (such as PTFE, polyvinylidene fluoride and polyimide and polydimethylsiloxane) are on the tribo-negative side and are more likely to be negatively charged. Nylon 11, Nylon 6, silk, aluminum foil and other materials are all on the positively charged side of the friction, which is more likely to become positively charged when rubbed against other materials [Citation57]. Therefore, in the early research on TENG, tribolayer materials are mainly concentrated in the two extremes shown in the figure below. With the deepening of research, tribolayer materials are no longer limited to simple materials, but begin to develop into composite materials.
Figure 2. Triboelectric series for some common materials [Citation57].
![Figure 2. Triboelectric series for some common materials [Citation57].](/cms/asset/1f69e92d-9313-4fa8-98d1-902cad83622d/tmrl_a_2026513_f0002_oc.jpg)
The compounding/doping of materials has a great influence on the contact electrification performance of the polymer. For example, a cost-effective TENG that uses polyvinylidene fluoride (PVDF) and microstructure polyvinyl alcohol (MS@PVA) polymers as the triboelectric materials for the anode and cathode polarization was prepared [Citation58], as shown in Figure (a). The PVDF film and its highly electroactive b-phase developed through a simple and cost-effective ultrasonic treatment process can be used to enhance the electric dipole–dipole interaction in the film and lead to an increase in its piezoelectric coefficient. In addition, the PVA film is produced by a simple curing method, and the microstructure on its surface is only developed by the corresponding reverse pattern on the commercially available sandpaper. The resulting TENG with PVDF and MS@PVA polymers showed the maximum peak-to-peak open-circuit voltage, short-circuit current and instantaneous output power density values, respectively, 230 V, 6 µA and 3.1 W/m2. Ultimately, the high electrical output produced by TENG is used to drive portable electronic devices such as displays and light-emitting diodes.
Figure 3. (a) Design of the PVDF and MS@PVA films-based TENG device [Citation58]. (b) Application of fur materials in TENG [Citation59]. (c) Schematic illustration of the fabrication of highly conductive ferroelectric BC composite paper [Citation60].
![Figure 3. (a) Design of the PVDF and MS@PVA films-based TENG device [Citation58]. (b) Application of fur materials in TENG [Citation59]. (c) Schematic illustration of the fabrication of highly conductive ferroelectric BC composite paper [Citation60].](/cms/asset/a7e7e567-426c-4b24-b5d2-9b2367cf1345/tmrl_a_2026513_f0003_oc.jpg)
In addition to the combination of materials, researchers are also actively looking for new triboelectrode materials to achieve greater electrical output performance of TENG. On this basis, some special materials were found, such as animal fur [Citation59]. As shown in Figure (b), an ultra-durable, low-wear TENG that uses animal fur as a triboelectric material for effective energy harvesting was developed. Due to its elasticity and softness, animal fur can maintain close contact and low friction with other tribolayer materials during long-term operation, thereby ensuring high output performance and low wear. By systematically measuring the output performance of the brush TENG (FB-TENG) in conventional rotating motions under various torque levels, the author discussed the influence of environmental humidity, material types and structural parameters. Even if the relative humidity is greatly increased from 40% to 90%, the performance of FB-TENG is almost unaffected by changes in humidity. A pair of meshing gears is also introduced to double the relative speed between the rotor and the stator to further increase the output power. Then, use the optimized FB-TENG to collect wind and water energy at different wind speeds and flows. Finally, by collecting energy from wind and water, a self-powered automatic irrigation, weather monitoring and wireless early warning system was constructed, demonstrating the potential applications of FB-TENG in agricultural big data and the ‘Internet of Things’.
While seeking the large electrical output of TENG, researchers are also exploring green and degradable materials as the tribolayer of TENG to prepare true ‘clean energy’ collection devices. As shown in Figure (c), the manufacture of high-conductivity ferroelectric cellulose composite paper and demonstrated their successful application in realizing large-area paper-based TENG with enhanced electrical output performance was reported [Citation60]. Cellulose-based composite paper contains silver (Ag) nanowires (NW) and BaTiO3 (BTO) NP in a bacterial cellulose (BC) matrix. Due to the percolation network of Ag NWs, it can be used not only as a positive triboelectric layer but also as a bottom electrode without integrating other electrodes. In addition, the ferroelectric properties derived from BTO NP facilitate charge transfer between the paper surface and the negative triboelectric layer, thereby achieving enhanced electrical output performance. Under the compression force of 5 kg, the output voltage and current of BC-TENG reached ≈170 V and ≈9.8 µA, respectively, and the optimized output power density was ≈180 µW cm−2, which exceeded the previous report, cellulose-based TENG. The author also demonstrated a large-area BC-TENG, which can directly operate a circuit containing 200 light-emitting diodes (LEDs) connected in series without the need for energy storage units. The results show that multifunctional cellulose-based paper has great potential in the manufacture of large-scale high-performance TENG devices.
2.2. Charge-storage property
In addition to the triboelectric polarity of the material, another internal factor that affects the contact charging behavior is the charge-storage capacity of the tribolayer materials. The difference between the charge-storage and triboelectric polarity of polymer materials is that the electrical property reflects the ability of the material to gain/loss electrons, while the charge-storage is a manifestation of the ability to capture and store charges [Citation61–65]. TENG is an energy supply device with large voltage and small current, which determines that the electrical energy it generates is difficult to store [Citation66–71]. However, the charge generated by friction can be temporarily stored in the surface (2 µm) of the bribolayer. These trapped charges will cause the back electrode to induce more charges, which are then converted into electrical output [Citation72–76]. Therefore, the electrical storage behavior of the material has a great influence on the electrical output of TENG. Generally speaking, the storage capacity of polymer materials depends on dielectric properties and capacitance. The greater the dielectric constant, the greater the capacitance, and the stronger the storage capacity of the material [Citation77–82]. For example, as shown in Figure (a), TENG was prepared using an electret composite film with adjustable dielectric constant [Citation83]. The composite film is composed of a thermoplastic polyurethane (TPU) matrix, polyethylene glycol (PEG) additives and polytetrafluoroethylene nanoparticles (PTFE-NPs) composition. By adjusting the PEG content, at a frequency of 100 Hz, the relative dielectric constant of the TPU matrix can be increased from 3.8 (PEG content is 0%) to 22.0 (PEG content is 20%). The results show that the increase in dielectric constant improves the injected charge density and internal capacitance of TENG, thereby increasing the output power and reducing the matching impedance. After systematically studying the influence of PEG content, the optimized TENG can increase the short-circuit current and output power by 7.5 and 17.3 times, respectively, and reduce the matching impedance by 90%.
Figure 4. (a) Structure of the TENG (i) and dielectric constant of TPU samples with different PEG content (ii, iii) [Citation81]. (b) Schematic diagrams of device fabrication and compatibility of graphene with an arbitrary substrate (i–iv), the device was neutral when no force was applied (v), and output voltage and current density from a Cu foil-grown 1L GTNG and randomly stacked 2L-, 3L-, and 4L-G-TENGs under a vertical compressive force of 1 kgf [Citation82]. (c) Schematic diagram of TENG structure (i) and comparison of charge dissipation between PP and PVA-based TENG under different humidity [Citation83].
![Figure 4. (a) Structure of the TENG (i) and dielectric constant of TPU samples with different PEG content (ii, iii) [Citation81]. (b) Schematic diagrams of device fabrication and compatibility of graphene with an arbitrary substrate (i–iv), the device was neutral when no force was applied (v), and output voltage and current density from a Cu foil-grown 1L GTNG and randomly stacked 2L-, 3L-, and 4L-G-TENGs under a vertical compressive force of 1 kgf [Citation82]. (c) Schematic diagram of TENG structure (i) and comparison of charge dissipation between PP and PVA-based TENG under different humidity [Citation83].](/cms/asset/f5ce3f67-76d9-4163-bced-08e000b1009f/tmrl_a_2026513_f0004_oc.jpg)
In addition to the influence of dielectric constant and capacitance, the doping of some nanomaterials with excellent charge trapping capabilities will also increase the charge storage capability of the friction layer. Common energy-storage materials include graphene, MXene and so on. Due to the existence of the interlayer voids of the 2D material of the sheet, the carriers are easily captured and stored. Furthermore, the excellent conductivity of the 2D material makes it easier for the trapped charges to be transferred to the surface of the friction layer and converted into electrical output, which reduces the internal resistance of the material and further increases the electrical output performance of TENG. As shown in Figure (b), graphene-based TENGs (G-TENGs) based on 1L, 2L, 3L, 4L, and few-layer graphene grown on Cu and Ni foils using chemical vapor deposition (CVD) have been fabricated, and their output voltage and output current density were measured under mechanical strains [Citation84]. The 1L-G-TENG exhibited a high output voltage and output current density of 5 V and 500 nA cm2, respectively. Additionally, the output voltage and output current density increased to 9 V and 1.2 A cm2, respectively, for the regularly stacked few-layer G-TENG. The ability of dielectric materials to store charges is not only reflected in the normal environment, but also in some extreme conditions such as high humidity environments. As shown in Figure (c), Compared with PP-based TENG, PVA-based TENG has greater electrical output performance in high humidity environments. In addition to the fact that PVA is rich in hydroxyl groups, it can form hydrogen bonds with water molecules in high humidity environments to fix water molecules to participate in triboelectricity. Another important reason is that PVA has better charge retention ability in high humidity environments. Reduce the dissipation of triboelectric charge to the environment. The results show that the current output of the PP- and PVA-based TENG both dropped to a stable value after 80 min at 35% RH, and the reduction rates were 26.5% and 21%. At 95% RH, the current output of the PP-based TENG decayed from 2.1 to 0.7 µA within 20 min with a reduction rate of 67%. However, the current output of the PVA-based TENG decayed to a stable value (35.1 µA) after 80 min with a reduction rate of 18%. These results show that PVA has a good charge retention ability under high environment, which effectively slows down the decay rate of triboelectricity [Citation85].
2.3. Environmental factor
In addition to internal factors, external environmental factors such as temperature, humidity and atmosphere will also affect the electrical output of TENG [Citation86–92]. For example, a new method to quantitatively study the relationship between real-time charge transfer and temperature in contact electrification through TENGs was reported [Citation74], which indicates that electron transfer is the main process of contact electrification between two inorganic solids, as shown in Figure (a). At different high temperatures, the evolution of surface charge density with time is consistent with the electron thermionic emission theory of friction pairs composed of Ti-SiO2 and Ti-Al2O3. In addition, it was found that there is a potential barrier on the surface, which can prevent the charge generated by contact electrification from flowing back into the solid that escapes from the surface after contact. This points out that the charge generated in contact electrification is easily kept at room temperature for several hours as the main reason why the electrostatic charge is retained by the material. In addition, based on the electron emission-based charge transfer mechanism, an electron-cloud-potential well model is proposed, which can generally be used to explain all types of contact electrification in conventional materials. In addition, AC-TENG and DC-TENG were used as test tools to systematically study the influence of various atmospheres on contact charging and electrostatic breakdown, as shown in Figure (b). The author theoretically analyzed the breakdown voltage of three common gases (air, O2, N2) and the output charge density of AC-TENG and DC-TENG in the three gas environments, and proposed strategies to improve the output performance of TENGs [Citation93]. The experiment confirmed the improvement of the charging of the oxygen contact with AC-TENG, and the obvious enhancement of the electrostatic breakdown in the oxygen with DC-TENG. The oxygen atmosphere is a good working atmosphere for DC-TENG. For practical applications, the author further manufactured a self-made high-performance DC-TENG stand-alone device in an oxygen atmosphere, and achieved a great increase in output power density. In addition to temperature and atmosphere, humidity also has a serious impact on the electrical output of TENG. Humidity will severely accelerate the generation and dissipation of triboelectricity on the surface, so the design of anti-humidity TENG is of great significance to extend the application of TENG. a bag-type TENG was prepared to significantly reduce the influence of relative humidity on its electrical output, and to obtain stable performance in a humid environment, as shown in Figure (c). First use nano-architecture polydimethylsiloxane (NA-PDMS) and multi-walled carbon nanotube/nylon composite layer as triboelectric materials with negative and positive trends to develop TENG (DD) based on dielectrics and dielectric materials). The NA-PDMS and nylon composite layer plays a key role in increasing the surface contact area and surface charge density between the dielectric/triboelectric materials and the output performance of DD-TENG. However, DD-TENG devices have stable and high output performance, and the effective output power density is about 25.35 W m−2. In addition, even if the relative humidity increases from 35% to 81%, the performance of the bag-type DD-TENG device is almost unaffected, while for non-bag-type devices, it drops significantly [Citation94].
Figure 5. (a) The influence of temperature on the electrical output of TENG. (i) Setup of the measurement platform. (ii) The total transferred changes QSC at room temperature and various high temperatures for three groups of experiments, respectively. (iii) The percentage of residual charges of the TENG at different temperatures. The residual charges are the QSC of the TENG after 5 min heat preservation at different temperatures. Inset is the diagram of the working model of the TENG. (iv) QSC evolution with time under high temperatures [Citation74]. (b) The influence of Air, N2 and O2 on TEN power supply output [Citation91]. (c) The influence of humidity on the electrical output of TENG. Photographic images of the (i) nonpouch- and (ii) pouch-type DD-TENG devices. Measured (iii) VOC and (iv) current curves of the pouch-type DD-TENG device under the relative humidity of 35%, which is observed in a normal room environment. (v) Photographic images and (vi) measured Voltage curves of the pouch-type DD-TENG device under various relative humidity conditions ranging from 47% to 81% [Citation92].
![Figure 5. (a) The influence of temperature on the electrical output of TENG. (i) Setup of the measurement platform. (ii) The total transferred changes QSC at room temperature and various high temperatures for three groups of experiments, respectively. (iii) The percentage of residual charges of the TENG at different temperatures. The residual charges are the QSC of the TENG after 5 min heat preservation at different temperatures. Inset is the diagram of the working model of the TENG. (iv) QSC evolution with time under high temperatures [Citation74]. (b) The influence of Air, N2 and O2 on TEN power supply output [Citation91]. (c) The influence of humidity on the electrical output of TENG. Photographic images of the (i) nonpouch- and (ii) pouch-type DD-TENG devices. Measured (iii) VOC and (iv) current curves of the pouch-type DD-TENG device under the relative humidity of 35%, which is observed in a normal room environment. (v) Photographic images and (vi) measured Voltage curves of the pouch-type DD-TENG device under various relative humidity conditions ranging from 47% to 81% [Citation92].](/cms/asset/e1c7cbdc-48ac-4daa-bb46-8782316ab571/tmrl_a_2026513_f0005_oc.jpg)
2.4. Others
In addition to the above-mentioned internal factors and environmental factors that affect the contact charging of the tribolayer, the surface structure [Citation95–98] and roughness of the friction layer [Citation99–103] will also affect the contact charging performance. Generally speaking, a material with a microstructure on the surface has a larger contact area during contact electrification, so the surface charge density is greater. Similarly, the greater the roughness of the interface, the stronger the contact charging performance. In the process of contact charging, the movement conditions and methods of the friction pair, such as impact force, contact area, movement speed, frequency, etc., will also affect the value of triboelectricity [Citation104–108]. Under the same control conditions, the greater the impact force, the greater the contact area, the faster the movement speed, and the higher the frequency, the greater the electrical output of TENG. Furthermore, the structural design and drive mode of TENG will also affect the size of the electrical output [Citation109–113].
3. Control methods of interface contact electrification
3.1. Improving triboelectric polarity
Since the energy generation in TENGs originates from the coupling effect of contact electrification and electrostatic induction, improving the contact charge generation on the dissimilar materials during the electrification process should be the intuitive and most effective approach to boost the energy output [Citation114–120]. As mentioned above, the main influencing factors of contact electrification are the electrical polarity and storage properties of the materials. Therefore, increasing the electrical polarity (electropositivity or electronegativity) of the tribolayer, or increasing the storage capacity of the triboelectric charge of the polymer material, can improve the contact electrification performance, thereby improving the electrical output performance of TENGs. There are many ways to improve triboelectric polarity, and the most commonly used method is surface engineering. By introducing functional groups that are easier to gain or lose electrons during contact electrification, it will be easier to generate triboelectric charge during electrification, which will directly increase the electrical output performance of TENGs [Citation121–126]. Generally speaking, according to the difficulty of gaining and losing electrons of the functional groups introduced on the polymer surface, these functional groups are divided into two categories, namely, positive functional groups (strong electron loss ability, positive after contact charging) and electronegative [Citation127–129]. The introduction of electronegative functional groups such as hydroxyl, aminomethoxy, etc. will make the tribolayer generate more positive charges (holes), while the introduction of electronegative functional groups such as carbonyl groups and halogens will make the tribolayer more negative charges (electrons) [Citation130–133]. As shown in Figure (a), two types of self-assembled monolayers thiols and silanes have been utilized to modify the surfaces of conductive and insulating materials, respectively [Citation122]. As a result, the triboelectric charges generated from the contact-electrification with a perfluorinated material fluorinated ethylene propylene (FEP) was improved effectively. By using different thiol molecules, the influence of head groups on the surface potential was systematically studied using scanning Kelvin probe microscopy (SKPM), which is in accordance with the influence on the surface charge density obtained from TENGs output. It can be found that amine (NH2) is the most triboelectrically-positive functional group that brings the largest enhancement to the generated charge density, while halogens (such as Cl) are the most triboelectrically-negative ones. The amine-functionalized surface is shown to be chemically stable during both a long period (over 90 days) and a huge number of continuous operating cycles (over 100,000) to generate a similar triboelectric charge density.
Figure 6. (a) Self-assembly surface functionalization for SiO2 as the triboelectric layer in TENG to enhance the output performance [Citation120]. (b) Molecular models with different amounts of –NH2 present (n1 < n2 < n3) [Citation132]. (c) The influence of the introduction of amino groups through molecular self-assembly on the dielectric and storage properties of PET films [Citation133]. (d) Synthesis and plausible mechanism of allicin grafting onto nanocellulose fiber, IR and comparison of the triboelectric output current (Isc) between various TENGs made of pristine cellulose, CNF-SH and Alc-S5-CNF film [Citation128].
![Figure 6. (a) Self-assembly surface functionalization for SiO2 as the triboelectric layer in TENG to enhance the output performance [Citation120]. (b) Molecular models with different amounts of –NH2 present (n1 < n2 < n3) [Citation132]. (c) The influence of the introduction of amino groups through molecular self-assembly on the dielectric and storage properties of PET films [Citation133]. (d) Synthesis and plausible mechanism of allicin grafting onto nanocellulose fiber, IR and comparison of the triboelectric output current (Isc) between various TENGs made of pristine cellulose, CNF-SH and Alc-S5-CNF film [Citation128].](/cms/asset/12e133fd-dd6e-4a52-a7aa-4f477b179c01/tmrl_a_2026513_f0006_oc.jpg)
In addition, to more intuitively prove the influence of the introduction of functional groups on contact charging, the microscopic surface potential of the modified polymer was tested to pass KPFM, as shown in Figure (b). The control of triboelectric charge density was studied with chemically tailored molecular surface modification, 3-aminopropyltriethoxysilane, 3-mercaptopropyltriethoxysilane, 3-cyanopropyltriethoxysilane, triethoxy-1 H,1H, 2H, 2H-tridecafluoro-n-octylsilane (PFOTES), N-Methylaminopropyltrimethoxysilane (NMAPS) and (N, N-dimethylaminopropyl) trimethoxysilane (NNMAPS) were selected to tailor the surface of cellulose nanofibrils (CNFs) [Citation128]. Kelvin probe force microscopy (KPFM) was used to measure the surface potential of CNFs before and after modification, that is to clarify the control of the triboelectric charge density of the materials by chemical customization from a microscopic point of view. The charge density of the CNFs was weakened by introducing electron-withdrawing groups, while the charge density was enhanced by introducing electron-donating groups. In addition, the number and density of functional groups were tailored on the surface of CNFs, and the range of charge density was adjusted more specifically. The introduction of functional groups can increase the power output of TENG by increasing the ability of the friction layer to gain/loss electrons during contact electrification. The increase in electrical storage is also an important factor. The introduction of certain functional groups such as amino groups can increase the dielectric constant and capacitance of the material, and improve the storage of triboelectric charges in the friction layer, as shown in Figure (c). The polyethylene terephthalate (PET) film has been modified by β-cyclodextrin to the surface through molecular self-assembly to introduce amidogen groups. The amidogen groups on the surface of the PET film can form hydrogen bonds with water molecules in the environment, making water molecules participate in triboelectricity as a more positive material, which will increase the electrical output of PET-based TENG in high-humidity environment. The results show that the circuit current (Isc) of the TENG increases by 3.3 times when the humidity increases from 15% to 95%. Moreover, the dielectric property of the PET film increases due to the introduction of amino groups in β-cyclodextrin, which causes the Isc of TENG to increase by 3.7 times at 15% RH [Citation134].
With the deepening of research, more and more functional groups are attempted to be modified on the surface of the polymer to prove the influence of the electronic effects on the contact electrification. Prior to this, the most studied functional groups were those containing oxygen, carbon, nitrogen and halogen, and the influence of the introduction of sulfur-containing functional groups on the electrical output of TENG has not been exploited. As shown in Figure (d), the researchers demonstrated for the first time the influence of the introduction of sulfur-containing functional groups on the contact electrification of the tribolayer. Allicin was grafted onto CNF using a thiol-ene click chemistry. It has been observed that after allicin modification, the CNF film became mechanically and thermally robust. The peak output voltage and current reached to 7.9 V and 5.13 µA, when modified film was used in a TENG, which was ∼6.5 times greater than the pristine cellulose-based TENG (1.23 V, 0.80 µA). Because of higher surface polarity, electron donating capacity and surface roughness of the modified film, the current density and power density increased from 0.2 to 1.28 A/cm2 and 0.25 to 10.13 W/cm2, i.e. 6 and 41 times greater respectively, than the pristine cellulose TENG. Such large electrical enhancements surpasseed many recent reports on cellulose TENG. Furthermore, the allicin grafted TENG showed outstanding stability against humidity, long-term cyclic load and environmental aging [Citation135].
Since contact electrification mainly occurs on the surface of the polymer, surface molecular assembly is the most direct and effective way to introduce functional groups. However, the functional groups introduced by molecular assembly usually have the disadvantage of weak bonding, making the surface charge density of the tribolayer during contact electrification become unstable, which seriously affects the electrical output of TENG [Citation136–138]. Although the chemical modification method will reduce the number of functional groups involved in contact electrification on the surface of the tribolayer, this method also has its unique advantages. First of all, the chemical grafting method usually improves the crystallinity of the polymer, which will make the hydroxyl and amino groups involved in the formation of intermolecular hydrogen bonds in the polymer leak to the maximum extent at the contact interface, directly increasing the electrical resistance of the friction layer [Citation139, Citation140]. Second, chemical modification occurs on the surface and inside of the material at the same time. The functional groups grafted on the surface can determine the electrical polarity of the material, while the internal functional groups will affect the electrical storage and electron transfer of the material, which will indirectly affect the contact electrification performance of the tribolayer [Citation123, Citation141]. Last but not least, the chemically grafted functional groups are very strong compared to molecular assembly, which makes the contact electrification properties of the modified polymer stable [Citation142–144].
Similar to the electronic effect of the functional group introduced by molecular assembly on the contact charging performance of the polymer, the functional group with the electron-withdrawing effect will make the modified polymer gain more negative charge during the triboelectric process, and have the electron-donating effect. Effective functional groups will allow the polymer to acquire more positive charges. Whether the polymer gets more negative charge or more positive charge, the electrical output of TENG will be greatly improved. Polyethyleneimine (PEI) is a highly branched polymer rich in amino groups. As an excellent electron-withdrawing group, the amino can be used to improve the triboelectricity of polymers. As shown in Figures (a and b), the uniformly sized Ag nanoparticles are prepared by the polyol method and uniformly coated on the surface of the CNF-PEI film [Citation145]. Consequently, a higher triboelectric positive polarity and a nanometer-scale surface are achieved; these significantly enhance the triboelectric output of TENG. The results show that the open-circuit voltage of the modified CNF film-based TENG is increased from 68 to 100 V, and the short-circuit current is increased from 0.6 to 1.1 µA. In addition, the introduction of functional groups with special functionalization can improve the hydrophilicity/hydrophobicity of the material, which can significantly improve the electrical output performance of TENG in a high-humidity environment. As shown in Figure (c), chemical functionalization is employed to control the surface polarizability and hydrophobicity of cellulose nanofibrils (CNFs) [Citation140]. Functional groups on the CNF surface are modified with triethoxy-1H,1H,2H,2H-tridecafluoro-n-octylsilane (PFOTES) in a straightforward and facile process. Fluorine-bearing silane chains are grafted to the surface of CNFs, which increases their triboelectric charge density and improves their hydrophobicity. Experimental results demonstrate that the surface polarity of CNFs is greatly improved after PFOTES modification. The PFOTES-CNF-based TENG exhibits good resistance to humidity and long-term cycle stability, and it retains 70% of the initial output performance at 70% ambient humidity. The short-circuit current of the PFOTES-CNF-based TENG reached 9.3 µA, which is about twice that of CNF-based TENG before modification.
Figure 7. (a) Real diagram and FE-SEM topography of CNF film before and after modification, peak distribution spectrum of C element in CNF film and peak distribution spectrum of C element in modified CNF film [Citation143]. (b) Influence mechanism of output performance, open-circuit voltage, short-circuit current and output charge of the CNF-based TENG before and after modification. (c) Preparation of the PFOTES-CNF film (i), short-circuit current (ii) and open circuit voltage under different RH (iii) [Citation144].
![Figure 7. (a) Real diagram and FE-SEM topography of CNF film before and after modification, peak distribution spectrum of C element in CNF film and peak distribution spectrum of C element in modified CNF film [Citation143]. (b) Influence mechanism of output performance, open-circuit voltage, short-circuit current and output charge of the CNF-based TENG before and after modification. (c) Preparation of the PFOTES-CNF film (i), short-circuit current (ii) and open circuit voltage under different RH (iii) [Citation144].](/cms/asset/d551c983-d1fd-41d3-8537-64ee8c7f1550/tmrl_a_2026513_f0007_oc.jpg)
The third method to increase the contact charging performance of the friction layer is to compound/dope materials with stronger electronegativity or electronegativity than the substrate. Generally speaking, materials with extreme frictional electrical polarity have strong ability to gain/loss electrons and electricity storage [Citation146–151], so the composite film will inherit the excellent contact charging performance of the compound material, especially for polymers with poor contact electrification properties. Furthermore, the combination of polymers can improve the mechanical properties of the substrate, making the modified polymer more suitable for the tribolayer of TENGs [Citation144, Citation152–156]. For example, a polymer electrolyte starch-based bio-TENG was fabricated using a low-cost processing method (Figures a and b). The films were electrically characterized at distinct loads, frequencies, thicknesses. Moreover, the starch polymer with CaCl2 was complexed to increase the triboelectrifying capacity and performance [Citation99]. Starch films at 0.5% of salt concentration reached the highest voltage output (1.2 V), exceeding threefold the initial output of the non-complexed pristine biopolymer (0.4 V). Furthermore, the electrical output performance varies positively at both thinner film thicknesses and elevated loads while moisture of films has been proved to be a critical parameter in the electrical performance of TENGs, showing that well-dried films performed a higher electrical output than moist samples. Furthermore, despite crack generation after fatigue, starch electrolyte films of TENGs showed an inalterable electrical performance suitable for a bunch of applications.
Figure 8. (a) Fabrication scheme of the TENGs based on nanostructured starch films [Citation155]. (b) Performance of TENG based on dielectric starch electrolyte. (c) Seawater degradable TENG (SD-TENG). (d–f) Photographs and SEM images of PLGA and PLA/PLGA thin films (100 µm thick) after degraded in 25°C seawater for different periods [Citation156]. (g) Configuration and working mechanism of skin-touch-actuated textile triboelectric nanogenerator [Citation99]. (h, i) Triboelectric performances of nanogenerators based on modified polyethylene terephthalate fabrics.
![Figure 8. (a) Fabrication scheme of the TENGs based on nanostructured starch films [Citation155]. (b) Performance of TENG based on dielectric starch electrolyte. (c) Seawater degradable TENG (SD-TENG). (d–f) Photographs and SEM images of PLGA and PLA/PLGA thin films (100 µm thick) after degraded in 25°C seawater for different periods [Citation156]. (g) Configuration and working mechanism of skin-touch-actuated textile triboelectric nanogenerator [Citation99]. (h, i) Triboelectric performances of nanogenerators based on modified polyethylene terephthalate fabrics.](/cms/asset/0fbaeebf-f6fc-4c05-a788-43dfc1b85396/tmrl_a_2026513_f0008_oc.jpg)
The tribolayer obtained by the compound modification of polymer materials may derive many interesting applications, such as degradable TENGs. As shown in Figures (c and d), a promising polymer blend (noted as PLA/PLGA), consisted from seawater-degradation-favorable poly (lactic-co-glycolic acid) (PLGA) and nonseawater-degradable polylatic acid (PLA), shows a mild rate degradation in seawater and complete weight loss within 9 months [Citation157]. Furthermore, the PLA/PLGA is found to be tribo-positive with excellent electrification properties and is mechanically robust. Then, a hollow-plate-shaped seawater degradable TENG (SD-TENG) is designed using PLA/PLGA as the electrification layer and Mg as the electrode, which demonstrates the ability to convert water wave vibration energy into electricity. In addition to the compounding between polymers, doped nanoparticles can also improve the electrical polarity of the friction layer, thereby improving the contact electrification performance. As shown in Figures (e and f), black phosphorus encapsulated with hydrophobic cellulose oleoyl ester nanoparticles serves as a synergetic electron-trapping coating, rendering a textile nanogenerator with long-term reliability and high triboelectricity regardless of various extreme deformations, severe washing, and extended environmental exposure [Citation158]. Considerably high output (∼250 880 V, ∼0.48 1.1 A cm 2) can be attained upon touching by hand with a small force (∼5 N) and low frequency (∼4 Hz), which can power light-emitting diodes and a digital watch. This conformable all-textile-nanogenerator is incorporable onto cloths/skin to capture the low output of 60 V from subtle involuntary friction with skin, well suited for user motion or daily operations.
In addition, some special methods can also achieve the purpose of regulating the triboelectricity of the polymer surface. Friction is a complex process of physical change. Therefore, various energy transfers occur during this process. The nature of triboelectricity is part of the transfer of many electrons in the friction process. From the energy point of view, friction is considered to be the process of transforming the kinetic energy of the friction pair into other forms of friction. Energy obtained by various means, including the excitation of phonons, photons and electrons. Theoretically, by controlling the energy and electron transfer in the friction process, the contact electrification can be adjusted. In addition to generating triboelectricity, other energy may also be generated during contact charging, such as friction light, friction heat, and chemical reactions. Therefore, by controlling the release of these energies, the mutual conversion between triboelectricity and light, heat or other energy is theoretically possible. On this basis, the concept of triboelectric control can be realized through the energy transfer and transformation in the friction process. As shown in Figures (a–c), a new method to control the release of triboelectric charges on polymer surfaces by interconversion between triboelectricity and mechanoluminescence (ML) has been proposed. In this method, the tribocharges are mitigated when ML is generated in the presence of a mediator, i.e. ZnS:Mn. Meanwhile, the presence of tribocharges increases ML intensity [Citation159]. Results show that when 10% ZnS:Mn is added and ML is generated, the current and voltage of ZnS:Mn@PDMS-based TENG are reduced by 96.4% and 94.9%, respectively. Meanwhile, when a charge of 11 nC is present on the surface of the film, the ML intensity increases by 84% compared with the uncharged film. Furthermore, based on the results of density functional theory calculations, triboelectrons on the polymer surface can easily enter the crystal lattice of ZnS to participate in ML, and the injection of electrons remarkably increases the ML intensity of ZnS:Mn.
Figure 9. (a) Schematic of the tribocharges and ML conversion on the surface of the ZnS: Mn @PDMS composite film during finger contact [Citation158]. (b) Short circuit current (Isc) of PDMS-based TENG with different contents of ZnS:Mn. (c) ML spectra of charged and uncharged ZnS: Mn @PDMS composite films with different ZnS:Mn content. (d) Schematic of near-infrared excitation of Fe3O4 electron emission [Citation41]. (e, f) The real-time current comparison between Fe3O4@PDMS-nylon 11-based TENG and Fe3O4@PDMS-PTFE-based TENG under repeated NIR irradiation. (g) Characterization of the new triboelectric series consisting of polydimethylsiloxane (PDMS), polypropylene (PP), polyethylene (PE), polycarbonate (PC), silica (SiO2), copper (Cu), glass, polyoxymethylene (POM), paper, polyamide (PA6), fabric nylon, and FOTS or APTS surfaces on these substrates, and molecular structure of FOTS and APTS and schematic diagram of possible antistatic surface coated with the mixture of FOTS and APTS at the molar ratio of λ [Citation159]. (h) Variation in electrostatic charges on coated PDMS surfaces with various l over time when the counter surface is copper. (i) Variation in electrostatic charges (left y axis) and coverage rate of PS spheres (right y axis) with the evolution of contact cycles on two kinds of surfaces, under a relative humidity of 60%.
![Figure 9. (a) Schematic of the tribocharges and ML conversion on the surface of the ZnS: Mn @PDMS composite film during finger contact [Citation158]. (b) Short circuit current (Isc) of PDMS-based TENG with different contents of ZnS:Mn. (c) ML spectra of charged and uncharged ZnS: Mn @PDMS composite films with different ZnS:Mn content. (d) Schematic of near-infrared excitation of Fe3O4 electron emission [Citation41]. (e, f) The real-time current comparison between Fe3O4@PDMS-nylon 11-based TENG and Fe3O4@PDMS-PTFE-based TENG under repeated NIR irradiation. (g) Characterization of the new triboelectric series consisting of polydimethylsiloxane (PDMS), polypropylene (PP), polyethylene (PE), polycarbonate (PC), silica (SiO2), copper (Cu), glass, polyoxymethylene (POM), paper, polyamide (PA6), fabric nylon, and FOTS or APTS surfaces on these substrates, and molecular structure of FOTS and APTS and schematic diagram of possible antistatic surface coated with the mixture of FOTS and APTS at the molar ratio of λ [Citation159]. (h) Variation in electrostatic charges on coated PDMS surfaces with various l over time when the counter surface is copper. (i) Variation in electrostatic charges (left y axis) and coverage rate of PS spheres (right y axis) with the evolution of contact cycles on two kinds of surfaces, under a relative humidity of 60%.](/cms/asset/0caaa89f-0a7b-4671-a998-c8d8f40bae58/tmrl_a_2026513_f0009_oc.jpg)
In addition to using the photoelectric conversion in the friction process to adjust the triboelectric charge on the polymer surface, external stimulation can also be used to adjust the triboelectric charge on the polymer surface. Based on the triboelectric electron transfer theory and thermionic emission theory, after friction, the triboelectric charge on the polymer surface can be neutralized by the electrons generated by infrared thermal excitation. Since the thermally excited electrons are generated instantaneously, the charge on the polymer surface will be quickly neutralized. In addition, thermally excited electrons can control the potential of the polymer surface. For example, for electropositive polymers, thermally excited electrons will neutralize the positive charge on the surface to achieve the purpose of anti-static; for electronegative polymers, thermally excited electrons will increase the surface potential, making the electric-negative property of the polymer enhancement. For example, the near-infrared (NIR) irradiation will cause the thermo electron emission of Fe3O4, thereby changing the charge amount on the PDMS surface (Figures d–f). Results show that the surface potential of Fe3O4@PDMS is reduced by 76.8% after NIR excitation when the PDMS surface is positively charged. In addition, the surface potential increases by 42.9% when the surface of Fe3O4 is negatively charged, showing the opposite trend. Moreover, the friction coefficient of the polymer can be adjusted by modifying the surface triboelectricity to change its motion state [Citation41]. In addition, by chemically polymerizing molecules with different electron gain/loss tendencies or physically spraying polymer coatings with different triboelectric properties, the distribution of triboelectricity on the polymer surface can be effectively controlled. As shown in Figures (g–i), a general toolbox was reported for designing antistatic coatings by leveraging on chemically heterogeneous components with electron-donating and electron-accepting functions, i.e. N-(2-aminoethyl)-3-aminopropyltrimethoxysilane and 1H, 1H, 2H, 2H-perfluorooctyl trimethoxysilane, to molecularly engineer the surface potential to achieve an electrostatic homogeneity [Citation160].
3.2. Improving charge-storage polarity
Another common method to control interface contact electrification is to increase the charge storage performance of the tribolayer. In the previous discussion, we mentioned that the mutual compounding of polymer materials can effectively improve the electron gain/loss ability of the friction layer; at the same time, the polymer with higher triboelectricity has excellent charge storage capacity can also improve the charge-storage property to a certain extent. Doping/compounding dielectric materials are the most direct and effective way to improve the storage performance of the tribolayer. In particular, lamellar 2D materials such as graphene, MXene and other common energy storage materials have become the first choice for improving the electrical storage performance of the friction layer due to the excellent charge trapping ability between the layers and the ultra-high capacitance. As shown in Figures (a and b), crumpled graphene (CG) layers and silicone films have been used to develop a smaller TENG with a better output performance [Citation161]. Owing to the stretchability of silicone and the unique structure of CG with continuously undulating 3D surfaces, the TENG could be operated in compressive, stretching, and hybridized modes, respectively. Intriguingly, it can generate higher output voltages with smaller areas, which is particularly suitable to develop smaller and more powerful TENG devices. In the traditional compressive mode, its output voltage is larger than that of the TENG composed of planar graphene (PG) layers in the control experiment by one magnitude. At the stretching mode, the TENG is capable of withstanding a maximum strain as large as 200% without evident degradation of the electrical properties. In addition, based on the superior stretchability of the device, it could be conformably attached to human skins to monitor the joint motions of fingers and wrists. In addition to increasing the charge storage performance of the friction layer to increase the electrical output of TENG, the introduction of two-dimensional inorganic materials such as graphene can also improve the mechanical strength and tribological properties of the polymer, making the friction layer material more suitable for real working conditions.
Figure 10. (a) Fabrication of stretchable TENG devices [Citation160]. (b) Output performance of TENGs with different crumple degrees. (c) Preparation and characterization of Ti3C2 MXene dispersions [Citation161]. (d) Electrochemical performance of MXene-coated cotton yarns with 78 wt% (2.5 mg cm−1) MXene loading using a three-electrode cell in 1 M H2SO4.
![Figure 10. (a) Fabrication of stretchable TENG devices [Citation160]. (b) Output performance of TENGs with different crumple degrees. (c) Preparation and characterization of Ti3C2 MXene dispersions [Citation161]. (d) Electrochemical performance of MXene-coated cotton yarns with 78 wt% (2.5 mg cm−1) MXene loading using a three-electrode cell in 1 M H2SO4.](/cms/asset/37b313b4-4151-4d59-a769-762697d3d3b0/tmrl_a_2026513_f0010_oc.jpg)
MXene is another commonly used filler to increase the storage capacity of the tribolayer. Similar to graphene, the lamellar structure allows it to effectively capture and store charges. More importantly, both graphene and MXene are nanomaterials with excellent conductivity, which can be used to prepare highly conductive yarns, which have great application potential in the field of flexible electronics. By coating cellulose yarns with Ti3C2Tx MXene, highly conductive and electroactive yarns are produced, which can be knitted into textiles using an industrial knitting machine (Figure c). It is shown that yarns with MXene loading of 77 wt% (≈2.2 mg cm−1) have conductivity of up to 440 S cm−1 (Figure d). After washing for 45 cycles at temperatures ranging from 30 to 80oC, MXene-coated cotton yarns exhibit a minimal increase in resistance while maintaining constant MXene loading [Citation162].
The method of introducing functional groups on the surface and inside of the tribolayer by means of surface molecular assembly or chemical grafting can also increase the electricity storage capacity of the polymer. As shown above, the main effect of the introduction of functional groups is to improve the ability of the tribolayer to gain/loss electrons. In fact, in many cases, changes in the electrical polarity and storage properties of polymers often occur at the same time, that is, while the ability to gain or lose electrons increases, charges are simultaneously captured and stored on the surface of the polymer. Different from the electrical storage mechanism of doped dielectric materials or 2D materials, the mechanism of improving the electrical storage capacity of polymers modified by introducing functional groups is the increase in dielectric properties or capacitance, which makes more triboelectric charge storage. Furthermore, chemical modification has stronger chemical bonds than doped/compounded dielectric materials, which can ensure that the electrical output of TENG is more stable during actual work. As mentioned above, by introducing a black phosphorus layer and a hydrophobic cellulose oleoyl ester nanoparticles (HCOENPs) coating on a PET fabric, a TENG with an improved output performance has been developed. However, a great amount of modifier is typically required to alter the tribopolarity of commercial fabrics, and the stability of the modified fabric would be a major concern that was not investigated in detail in previous works, which is the main difference between physical doping and chemical modification. So, how to create tough chemical linkages between pristine fabric fibers and triboelectric modifiers and generate a hierarchical topography on the fiber surface without sacrificing long-term stability are grand challenges for further improving the output performance of fabric-based TENGs. As shown in Figures (a and b), chemically grafting poly (ether imide) (PEI) with cellulose nanofibrils (CNFs) significantly improved the tribopositivity of CNF since amide bonds are electron-donating groups that are easy to be positively charged. CNTs and branched PEI was used to achieve simultaneous manipulation of the surface topography and surface chemical composition of a typical commercial velvet fabric [Citation163]. With a very low optimized modifier content (less than 1 wt%), the modified velvet fabric could generate a 16.3 V voltage when paired with pristine velvet, which was improved by 23.3 times. When paired with PTFE, a high voltage of 119 V and a current of 12.6 µA were reached. A power density of 3.2 W/m2 was achieved on a 5 106 Ω resistor, which allows the fabric-based TENG to be able to power various electronics.
Figure 11. (a) Schematic of the grafting mechanism of CNT−COOH and PEI, and illustration modification process of the velvet fabric using CNT and PEI [Citation162]. (b) Output voltage results of TENGs assembled using different modified fabrics as electropositive layer. (c) The schematic illustration of the fabrication process of leaf and leaf powder-based TENGs [Citation163]. (d) Schematic illustration of the PLL modify process on dry leaf and leaf powder and output performance of leaf and leaf powder-based TENGs before and after PLL modification.
![Figure 11. (a) Schematic of the grafting mechanism of CNT−COOH and PEI, and illustration modification process of the velvet fabric using CNT and PEI [Citation162]. (b) Output voltage results of TENGs assembled using different modified fabrics as electropositive layer. (c) The schematic illustration of the fabrication process of leaf and leaf powder-based TENGs [Citation163]. (d) Schematic illustration of the PLL modify process on dry leaf and leaf powder and output performance of leaf and leaf powder-based TENGs before and after PLL modification.](/cms/asset/0acbb75a-f902-427f-bd8f-04b8878ac1b4/tmrl_a_2026513_f0011_oc.jpg)
Similar to chemical modification, the introduction of macromolecular groups into the surface of the polymer by means of surface molecular assembly can also significantly improve the dielectric or capacitance of the tribolayer, thereby improving the contact electrification performance. Commonly used molecular assembly materials are macromolecules containing oxygen, nitrogen, sulfur or fluorine groups. Generally speaking, the introduction of oxygen and nitrogen-containing groups can not only significantly increase the electrical positiveness of the material but also significantly improve the storage capacity. In addition to increasing the electronegativity of the tribolayer, the introduction of fluorine-containing groups can also significantly improve the hydrophobic properties of the material surface, which will be more conducive to the enhancement of the electrical output of solid–liquid TENG (not discussed in detail in this article). As shown in Figures (c and d), TENGs based on biodegradable plant leaf and leaf powder is fabricated through a simple and cost-effective method. The short-circuit current (Isc) and output voltage (Vo) of fresh leaf can reach 15 µA and 430 V. Dry leaf is grinded into powder to make solve problem of frangibility when contact and make full use of the leaf [Citation164]. Poly-L-Lysine is used to modify leaf powder and enhance the output performance of leaf powder-based TENG. After surface modification, the Isc and Vo can reach high as 60 µA and 1000 V, respectively, which can easily power a commercial electric watch and 868 LEDs. To extend the drive mode, wind-driven TENG (WTENG) based on PLL modified leaf powder is designed for harvesting wind energy and the maximum Isc can reach 150 µA under 7 m/s wind speed.
As mentioned above, when preparing the friction layer of TENG with leaves, polyimide (PI) is needed as the middle layer of the electrode. Indeed, PI is a dielectric material with excellent charge storage capabilities. At a frequency of 1000 HZ, the dielectric constant of PI is 4.0, but the dielectric loss is only 0.004–0.007, indicating that more charge is stored rather than dissipated to the environment. Different from the mechanism mentioned above by mixing 2D materials and chemical/physical methods to introduce functional groups to increase the electric storage capacity of the friction layer, the mechanism of PI as an intermediate layer to improve the electric storage capacity is the contact electrification of the friction layer. In the coupling process of the induction electrification of the back electrode, the PI intermediate layer relies on its excellent storage capacity to increase the amount of charge of the induction electrification of the back electrode, thereby increasing the electrical output of the TENG. In other words, the ability to gain/loss electrons and storage capacity of the friction layer is not directly enhanced, but the transition through the intermediate layer, the induced charge is amplified, thereby improving the electrical output of the entire TENG.
The research mentioned in Figure is a typical representative work of PI as an intermediate transition layer to increase the electrical output of TENG [Citation99]. A new structured TENG was designed by adding a transition layer between the friction layer and the conducting layer, which could significantly improve the output performance by one order of magnitude. The material of transition layer, such as polyimide, has high ability to store the triboelectrification charges, resulting in more induced charges and higher external current. After adding a polyimide charge storage layer with a thickness of 25 µm, the short-circuit current and output voltage of polyvinylidene fluoride (PVDF) and nylon (NY) based TENG (Cu-PIPVDF@NY-Cu) increased from 9.2 µA to 65 µA, and 110 to 1010 V, respectively. The maximum charge density can reach approximately 105 µC/m2 with the maximum value of the output power of 5.87 mW under 4 MΩ loading resistance, which can instantaneous light up 992 commercial LEDs and charge a capacitor with the speed increased by 10 times. Moreover, the mechanism and influence factors including the surface structure, composition and thickness of the charge-keeping layer to enhance the output of TENGs were discussed in detail. The charge decay tests of the transition layers showed that polyimide layer has very good charge keeping ability with a decay rate of only about 20% in 4 h, while the charge of PVDF decreases about 97% in 4 h, which is a key factor for its lower output.
Figure 12. (a) Schematic illustration of fabrication processes of triboelectric nanogenerator without and with PI as transition layer. (b, c) Short-circuit current (Isc) and output voltage (Vo) performance of TENGs with and without PI as charge. (d, e) Output performance of PI and PVDF-based TENGs Isc and charge. (f, g) Isc and charge decay of PVDF, PI, PI-PVDF and PVDF-PI. (h, i) Isc of the first 3s when PVDF and NY get into contact and the previous parts when TENGs reach the maximum value. (j, h) Charge decay of PVDF, PI, PI-PVDF and PVDF-PI [Citation155].
![Figure 12. (a) Schematic illustration of fabrication processes of triboelectric nanogenerator without and with PI as transition layer. (b, c) Short-circuit current (Isc) and output voltage (Vo) performance of TENGs with and without PI as charge. (d, e) Output performance of PI and PVDF-based TENGs Isc and charge. (f, g) Isc and charge decay of PVDF, PI, PI-PVDF and PVDF-PI. (h, i) Isc of the first 3s when PVDF and NY get into contact and the previous parts when TENGs reach the maximum value. (j, h) Charge decay of PVDF, PI, PI-PVDF and PVDF-PI [Citation155].](/cms/asset/4463058c-0158-4141-8290-29fced05904d/tmrl_a_2026513_f0012_oc.jpg)
Subsequently, different methods used to increase the output of TENGs in recent years were compared, as shown in Table . The results show that any method is of great help to the electrical output of TENG. There is no distinction between these methods because they have their advantages and disadvantages. For example, after adding PI storage layer, the electrical output of TENG will increase, but the flexibility and transparency will decrease. Meanwhile, the bonding force between the layers of this method is weaker due to the van der Waals force compared with the chemical modification method. We should decide which method to choose to increase the electrical output of TENG based on the actual situation.
Table 1. Summary of different methods on the charge density of TENGs.
4. Applications of TENGs
As a smart device, TENGs, using the coupling effect of the well-known contact charging and electrostatic induction to transform environmental energy into electrical energy, for instance, water wave energy, wind energy, mechanical energy generated through body movement and some other mechanical movements [Citation165–170]. Furthermore, TENGs have also exhibited promising potential applications as a power supply to drive some small electronic products, and also shown great potential in sensors, lighting, anticorrosion and some other fields [Citation29, Citation171–174]. The most common application of TENGs is as an energy harvesting device to power small devices such as commercial light-emitting diodes, electronic watches, and wireless transmitters (Figure ). Also, as a power module, the main difference between TENGs and batteries is that TENGs are self-powered components, which essentially convert mechanical energy in the environment into electrical energy, achieving the purpose of continuous power supply and clean energy [Citation175–177]. In the actual application process, TENG has a variety of driving methods. For example, the driving of TENG attached to the sole is based on the contact-separation between the sole and the ground; the coated electrode applied on the wall can be rubbed by hand. Achieve single-electrode TENG driving; TENG sewn on clothes can be powered by TENG through the friction between the sleeve and the electrode; the friction layer can be contacted and separated by wind blowing to generate triboelectricity; the ship can be driven by waves hitting the hull Anti-corrosion coating TENG; the beating of raindrops can realize the drive of solid–liquid TENG and so on [Citation7,Citation178–180]. All driving methods have a common feature: the friction layers must meet the contact and separation at the same time, which is a necessary condition for TENG to generate triboelectricity.
Figure 13. Applications of TENGs in energy harvesting, sensor, medicine, coating, anticorrosion other fields.
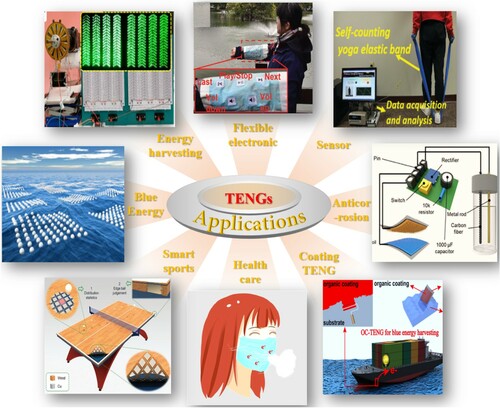
Based on this, the application range of TENG has been unlimitedly expanded. Therefore, in addition to energy harvesting, flexible electronics has become a new hot spot in TENG applications. As a flexible wearable self-powered energy module, TENG can power small sensors to replace traditional batteries. Moreover, the special tribolayer materials of TENGs can also rely on changes in temperature, humidity, atmosphere or pressure to achieve self-powered sensing. In the medical field, the application of TENGs is also ubiquitous [Citation181–183]. For example, based on PVA's excellent charge storage capacity and combined water molecules to participate in contact charging to increase triboelectric performance in a high-humidity environment, PVA is electrospun to prepare the electrostatic adsorption layer of medical masks that has self-charging performance and stronger electrostatic adsorption capacity, which has great application value during the virus pandemic period. In addition, TENG attached to the back can monitor various postural changes of the human body and has potential applications in medical rehabilitation; flexible TENG attached to the skin can monitor changes in blood oxygen and body temperature, which is of great significance in the detection of new coronaviruses.
Under actual working conditions, the wear problem of the tribolayer of TENG is unavoidable. Abrasion will directly cause the decrease or instability of the electrical output of TENG, and even damage the device. In addition, the metal friction layer will also face problems such as corrosion and pollution. Therefore, the coating TENG came into being. Coating TENG is committed to solving the problems of wear, corrosion and pollution of the friction layer while enhancing the electrical output. The materials used to prepare the coating TENG are mostly resin polymers, such as epoxy resin, phenolic resin, methyl acrylate, etc., which have strong electronegativity and are ideal electronegative tribolayer in solid–liquid TENG [Citation184, Citation185]. For example, fluorine-containing macromolecules are introduced into acrylic resin to increase the electronegativity of the material, and then the modified resin is coated on the hull of a model cruise ship. This packaged organic coating triboelectric nanogenerator provides good stability and high-output performance, which can easily light several commercial LEDs on a model ship by collecting the wave energy during the voyage. This new type of triboelectric nanogenerator based on the coating material has the advantages of simple process, low cost and large-area preparation, which combines the performance of the coating itself with the power generation function, and have potential promising practical applications in ocean energy collection and utilization, self-powered sensing, and other fields [Citation186–190]. In the field of anti-corrosion, TENGs can effectively protect the cathode (metallic material) from salt water corrosion. In the self-powered anticorrosion system, a capacitor was introduced to stabilize the voltage and storage the charges during the TENG’s reciprocating running process. The TENG was connected to a rectifier to convert the alternating-current (AC) to direct-current (DC), which is essential for cathodic protection [Citation191–193]. Although the short-circuit current is not direct-current, the current is still in one direction, which is probably able to prevent iron from corrosion through cathodic protection system.
In addition to the conventional applications mentioned above, TENG also has potential applications in other fields, such as methane mitigation thermoelectric generators and magneto-mechano-triboelectric nanogenerators (MMTENG)[Citation194–199]. As shown in Figure , a thermoelectric (TE) module is a solid-state device that can transform the temperature difference between the two sides of the module into an electrical potential difference by means of the see beck effect. TE modules are noiseless and have good reliability. Though currently commercialized TE modules have relatively low efficiency, a thermoelectric generator (TEG) comprising TE modules and heat exchangers has been consistently considered as an alternative for waste heat recovery, where efficiency is less important. The methane mitigation thermoelectric generators have been fabricated by employing a magnet-attached cantilever structure and triboelectric materials to initially transduce an AC magnetic field into mechanical oscillations by magnetic force, which is subsequently converted to electricity by the triboelectric effect. With the advent of magneto-mechano-triboelectric conversion, lighting up a biomedical f-µLED under an ambient magnetic field could be beneficial to realize battery-free phototherapeutic devices.
Figure 14. Applications of TENGs in thermoelectric generators and magneto-mechano-triboelectric nanogenerators.
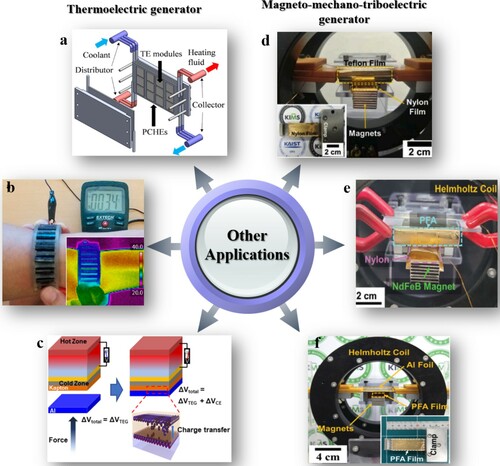
With the unremitting efforts of researchers, the application fields of TENGs have been continuously expanded, and the intersection between disciplines has provided unlimited possibilities for the future development of TENG applications. We believe that with the deepening of research, more meaningful applications of TENGs will be reported, and we will wait and see.
5. Conclusion and future perspective
In this paper, we primarily reviewed the recent advances in TENG's interface contact electrification influencing factors, control methods and applications. We first covered the details of the factors that affect charge density of the polymer contact electrification process, including internal factors (triboelectric polarity, storage performance) and external factors (environmental factors). Then, we introduced how to increase the friction layer's ability to gain/loss electrons and increase the friction layer's charge storage capacity by improving the triboelectricity of the polymer to increase and control the contact charging performance of the friction layer of TENG. Finally, we introduced the application of TENG in energy harvesting, sensing, medicine, coating, anti-corrosion and other fields.
In view of the difficulties faced in the development of TENG, we think there are three directions for TENG's future development. First of all, TENG is an energy supply device with high voltage but low current, which causes it to only supply power for some small devices at present, which severely limits practical applications. Although there are many meaningful works to solve the problem of small current breakthroughs that have been made, but the current known electrical output of TENG still has not exceeded the ampere (A) level. To realize the real industrialization of TENG, how to achieve the balance between voltage and current still needs to be solved urgently. Second, how to realize the energy storage of TENG is also an obstacle in the development of TENG. At present, to realize TENG as a self-powered energy module, it is necessary to maintain the continuous contact-separation state of the friction layer. Once the contact or separation stops, TENG will stop working. Therefore, how to realize the storage of triboelectricity in the moving state and supply power to the equipment in the static state is also a problem that needs to be solved in the future development of TENG. Last but not least, ‘blue energy’, the collection of ocean energy, will be an important way out for TENG's large-scale application in the future. On this basis, combining TENG with anti-corrosion and anti-fouling coatings may be a feasible way for the development of TENG in the future.
Acknowledgements
Thanks for the financial support of the Program for Taishan Scholars of Shandong Province (No. ts20190965), the National Key Research and Development Program of China (2020YFF0304600), the National Natural Science Foundation of China (No. 51905518), the National Natural Science Foundation of China (No. U21A2046, 51905518), the Key Research Program of the Chinese Academy of Sciences (grant number XDPB24) and the Innovation Leading Talents Program of Qingdao (19-3-2-23-zhc) in China. N. Wang and Z. Li would also like to thank the financial support from the Agency for Science, Technology and Research (A*STAR), Science and Engineering Research Council (SERC) Central Research Fund (Use-inspired Basic Research) for this work.
Disclosure statement
No potential conflict of interest was reported by the author(s).
Additional information
Funding
References
- Xu C, Zhang B, Wang AC, et al. Contact-electrification between two identical materials: curvature effect. ACS Nano. 2019;13:2034–2041.
- Chen L, Shi Q, Sun Y, et al. Controlling surface charge generated by contact electrification: strategies and applications. Adv Mater. 2018;30:e1802405.
- Lin S, Xu L, Xu C, et al. Electron transfer in nanoscale contact electrification: effect of temperature in the metal-dielectric case. Adv Mater. 2019;31:e1808197.
- Wang ZL, Wang AC. On the origin of contact-electrification. Mater Today. 2019;30:34–51.
- Cezan SD, Nalbant AA, Buyuktemiz M, et al. Control of triboelectric charges on common polymers by photoexcitation of organic dyes. Nat Commun. 2019;10:276.
- He W, Liu W, Chen J, et al. Boosting output performance of sliding mode triboelectric nanogenerator by charge space-accumulation effect. Nat Commun. 2020;11:4277.
- Wang H, Xu L, Bai Y, et al. Pumping up the charge density of a triboelectric nanogenerator by charge-shuttling. Nat Commun. 2020;11:4203.
- Chen L, Chen C, Jin L. Stretchable negative Poisson’s ratio yarn for triboelectric nanogenerator for environmental energy harvesting and self-powered sensor. Energy Environ Sci. 2021;14:955.
- Wong P-Y, Phang S-W, Baharum A. Effects of synthesised polyaniline (PAni) contents on the anti-static properties of PAni-based polylactic acid (PLA) films. RSC Adv. 2020;10:39693–39699.
- Wang J, Bao L, Zhao H, et al. Preparation and characterization of permanently anti-static packaging composites composed of high impact polystyrene and ion-conductive polyamide elastomer. Compos Sci Technol. 2012;72:976–981.
- Wang W, Yu A, Liu X, et al. Large-scale fabrication of robust textile triboelectric nanogenerators. Nano Energy. 2020;71:104605.
- Deng W, Zhou Y, Zhao X, et al. Ternary electrification layered architecture for high-performance triboelectric nanogenerators. ACS Nano. 2020;14:9050–9058.
- Liu Z, Li H, Shi B, et al. Wearable and implantable triboelectric nanogenerators. Adv Funct Mater. 2019;29:1808820.
- Zou Y, Raveendran V, Chen J. Wearable triboelectric nanogenerators for biomechanical energy harvesting. Nano Energy. 2020;77:105303.
- Fan F-R, Tian Z-Q, Lin Wang Z. Flexible triboelectric generator. Nano Energy. 2012;1:328–334.
- Bai Y, Xu L, Lin S, et al. Charge pumping strategy for rotation and sliding type triboelectric nanogenerators. Adv Energy Mater. 2020;10:2000605.
- Wang H, Han M, Song Y, et al. Design, manufacturing and applications of wearable triboelectric nanogenerators. Nano Energy. 2020: 105627.
- Zhao Z, Huang Q, Yan C, et al. Machine-washable and breathable pressure sensors based on triboelectric nanogenerators enabled by textile technologies. Nano Energy. 2020;70:104528.
- Luo J, Wang ZL. Recent progress of triboelectric nanogenerators: from fundamental theory to practical applications. EcoMat. 2020;2:e12059.
- Luo J, Wang Z, Xu L, et al. Flexible and durable wood-based triboelectric nanogenerators for self-powered sensing in athletic big data analytics. Nat Commun. 2019;10:5147.
- Song Y, Wang N, Wang Y, et al. Direct current triboelectric nanogenerators. Adv Energy Mater. 2020;10:2002756.
- Cheng R, Dong K, Liu L, et al. Flame-retardant textile-based triboelectric nanogenerators for fire protection applications. ACS Nano. 2020;14:15853–15863.
- Rodrigues C, Nunes D, Clemente D, et al. Emerging triboelectric nanogenerators for ocean wave energy harvesting: state of the art and future perspectives. Energy Environ Sci. 2020;13:2657–2683.
- Dong K, Peng X, Wang ZL. Fiber/fabric-based piezoelectric and triboelectric nanogenerators for flexible/stretchable and wearable electronics and artificial intelligence. Adv Mater. 2020;32:1902549.
- Ahmed A, Hassan I, El-Kady MF, et al. Integrated triboelectric nanogenerators in the era of the internet of things. Adv Sci. 2019;6:1802230.
- Wang H, Zhu J, He T, et al. Programmed-triboelectric nanogenerators—a multi-switch regulation methodology for energy manipulation. Nano Energy. 2020;78:105241.
- Conta G, Libanori A, Tat T, et al. Triboelectric nanogenerators for therapeutic electrical stimulation. Adv Mater. 2021: 2007502.
- Peng X, Dong K, Ye C, et al. A breathable, biodegradable, antibacterial, and self-powered electronic skin based on all-nanofiber triboelectric nanogenerators. Sci Adv. 2020;6: eaba9624.
- Cheng T, Gao Q, Wang ZL. The current development and future outlook of triboelectric nanogenerators: a survey of literature. Adv Mater Tech. 2019;4:1800588.
- Harmon W, Bamgboje D, Guo H, et al. Self-driven power management system for triboelectric nanogenerators. Nano Energy. 2020;71:104642.
- Dong K, Peng X, An J, et al. Shape adaptable and highly resilient 3D braided triboelectric nanogenerators as e-textiles for power and sensing. Nat Commun. 2020;11:1–11.
- Dharmasena R, Silva S. Towards optimized triboelectric nanogenerators. Nano Energy. 2019;62:530–549.
- Lin Z, Zhang B, Zou H, et al. Rationally designed rotation triboelectric nanogenerators with much extended lifetime and durability. Nano Energy. 2020;68:104378.
- Paosangthong W, Torah R, Beeby S. Recent progress on textile-based triboelectric nanogenerators. Nano Energy. 2019;55:401–423.
- Ryu H, Park H-m, Kim M-K, et al. Self-rechargeable cardiac pacemaker system with triboelectric nanogenerators. Nat Commun. 2021;12:1–9.
- Lai Y, Hsiao Y, Wu H. Waterproof fabric-based multifunctional triboelectric nanogenerator for universally harvesting energy from raindrops, wind, and human motions and as self-powered sensors. Adv Sci. 2019;6:1801883.
- Xu L, Xu L, Luo J, et al. Hybrid all-in-one power source based on high-performance spherical triboelectric nanogenerators for harvesting environmental energy. Adv Energy Mater. 2020;10:2001669.
- Chen A, Zhang C, Zhu G, et al. Polymer materials for high-performance triboelectric nanogenerators. Adv Sci. 2020;7:2000186.
- Zhang Z, Jiang D, Zhao J, et al. Tribovoltaic effect on metal–semiconductor interface for direct-current low-impedance triboelectric nanogenerators. Adv Energy Mater. 2020;10:1903713.
- Li S, Liu D, Zhao Z, et al. A fully self-powered vibration monitoring system driven by dual-mode triboelectric nanogenerators. Acs Nano. 2020;14:2475–2482.
- Wang N, Feng Y, Zheng Y, et al. Triboelectrification of interface controlled by photothermal materials based on electron transfer. Nano Energy. 2021;89:106336.
- Qian C, Li L, Gao M, et al. All-printed 3D hierarchically structured cellulose aerogel based triboelectric nanogenerator for multi-functional sensors. Nano Energy. 2019;63:103885.
- Zhang R, Dahlstrom C, Zou H, et al. Cellulose-based fully Green triboelectric nanogenerators with output power density of 300 W m(-2). Adv Mater. 2020;32:e2002824.
- Nie S, Cai C, Lin X, et al. Chemically functionalized cellulose nanofibrils for improving triboelectric charge density of a triboelectric nanogenerator. ACS Sustain Chem Eng. 2020;8:18678–18685.
- Yao C, Yin X, Yu Y, et al. Chemically functionalized natural cellulose materials for effective triboelectric nanogenerator development. Adv Funct Mater. 2017;27:201700794.
- He X, Zou H, Geng Z, et al. A Hierarchically nanostructured cellulose fiber-based triboelectric nanogenerator for self-powered healthcare products. Adv Funct Mater. 2018;28:201805540.
- Zhao Z, Zhou L, Li S, et al. Selection rules of triboelectric materials for direct-current triboelectric nanogenerator. Nat Commun. 2021;12:1–8.
- Wei XY, Zhu G, Wang ZL. Surface-charge engineering for high-performance triboelectric nanogenerator based on identical electrification materials. Nano Energy. 2014;10:83–89.
- Wang ZL. Triboelectric nanogenerator (TENG)—sparking an energy and sensor revolution. Adv Energy Mater. 2020;10:2000137.
- Sun J, Li W, Liu G, et al. Triboelectric nanogenerator based on biocompatible polymer materials. J Phys Chem C. 2015;119:9061–9068.
- Wu C, Wang AC, Ding W, et al. Triboelectric nanogenerator: a foundation of the energy for the new era. Adv Energy Mater. 2019;9:1802906.
- Kim H-J, Yim E-C, Kim J-H, et al. Bacterial nano-cellulose triboelectric nanogenerator. Nano Energy. 2017;33:130–137.
- Liu X, Zhao K, Yang Y. Effective polarization of ferroelectric materials by using a triboelectric nanogenerator to scavenge wind energy. Nano Energy. 2018;53:622–629.
- Li Y, Zhao Z, Gao Y, et al. Low-cost, environmentally friendly, and high-performance triboelectric nanogenerator based on a common waste material. ACS Appl Mater Interfaces. 2021;13:30776–30784.
- Khandelwal G, Chandrasekhar A, Maria Joseph Raj NP, et al. Metal–organic framework: A novel material for triboelectric nanogenerator–based self-powered sensors and systems. Adv Energy Mater. 2019;9:1803581.
- Yu Z, Wang Y, Zheng J, et al. Rapidly fabricated triboelectric nanogenerator employing insoluble and infusible biomass materials by fused deposition modeling. Nano Energy. 2020;68:104382.
- Zou H, Zhang Y, Guo L, et al. Quantifying the triboelectric series. Nat Commun. 2019;10:1427.
- Patnam H, Dudem B, Graham SA, et al. High-performance and robust triboelectric nanogenerators based on optimal microstructured poly(vinyl alcohol) and poly(vinylidene fluoride) polymers for self-powered electronic applications. Energy. 2021;223:120031.
- Chen P, An J, Shu S, et al. Super-Durable, Low-wear, and high-performance fur-brush triboelectric nanogenerator for wind and water energy harvesting for smart agriculture. Adv Energy Mater. 2021;11:02003066.
- Oh H, Kwak SS, Kim B, et al. Highly conductive ferroelectric cellulose composite papers for efficient triboelectric nanogenerators. Adva Funct Mater. 2019;29:201904066.
- Yao Y, Jiang T, Zhang L, et al. Charging system optimization of triboelectric nanogenerator for water wave energy harvesting and storage. ACS Appl Mater Interfaces. 2016;8:21398–21406.
- Wang X, Yang Y. Effective energy storage from a hybridized electromagnetic-triboelectric nanogenerator. Nano Energy. 2017;32:36–41.
- Choi J H, Ra Y, Cho S, et al. Electrical charge storage effect in carbon based polymer composite for long-term performance enhancement of the triboelectric nanogenerator. Compos Sci Technol. 2021;207:108680.
- Li Z, Zhu M, Qiu Q, et al. Multilayered fiber-based triboelectric nanogenerator with high performance for biomechanical energy harvesting. Nano Energy. 2018;53:726–733.
- Luo J, Wang ZL. Recent advances in triboelectric nanogenerator based self-charging power systems. Energy Storage Materials. 2019;23:617–628.
- Kwak S S, Kim S M, Ryu H, et al. Butylated melamine formaldehyde as a durable and highly positive friction layer for stable, high output triboelectric nanogenerators. Energy Environ Sci. 2019;12:3156–3163.
- Jiang H, Lei H, Wen Z, et al. Charge-trapping-blocking layer for enhanced triboelectric nanogenerators. Nano Energy. 2020;75:105011.
- Bai Y, Xu L, He C, et al. High-performance triboelectric nanogenerators for self-powered, in-situ and real-time water quality mapping. Nano Energy. 2019;66:104117.
- Hu Y, Zheng Z. Progress in textile-based triboelectric nanogenerators for smart fabrics. Nano Energy. 2019;56:16–24.
- Yang D, Ni Y, Kong X, et al. Self-healing and elastic triboelectric nanogenerators for muscle motion monitoring and photothermal treatment. ACS Nano. 2021;15:14653–14661.
- Wang Y, Duan J, Yang X, et al. The unique dielectricity of inorganic perovskites toward high-performance triboelectric nanogenerators. Nano Energy. 2020;69:104418.
- Lin S, Zheng M, Luo J, et al. Effects of surface functional groups on electron transfer at liquid-solid interfacial contact electrification. ACS Nano. 2020;14:10733–10741.
- Li Y, Zheng W, Zhang H, et al. Electron transfer mechanism of graphene/Cu heterostructure for improving the stability of triboelectric nanogenerators. Nano Energy. 2020;70:104540.
- Xu C, Zi Y, Wang AC, et al. On the electron-transfer mechanism in the contact-electrification effect. Adv Mater. 2018;30:e1706790.
- Lee H, Lee HE, Wang HS, et al. Hierarchically surface-textured ultrastable hybrid film for large-scale triboelectric nanogenerators. Adv Funct Mater. 2020;30:2005610.
- Kang S-M, Lee HE, Wang HS, et al. Self-Powered flexible full-color display via dielectric-tuned hybrimer triboelectric nanogenerators. ACS Energy Lett. 2021;6:4097–4107.
- Cui N, Gu L, Lei Y, et al. Dynamic behavior of the triboelectric charges and structural optimization of the friction layer for a triboelectric nanogenerator. ACS Nano. 2016;10:6131–6138.
- Zi Y, Wang J, Wang S, et al. Effective energy storage from a triboelectric nanogenerator. Nat Commun. 2016;7:1–8.
- Chen J, Guo H, He X, et al. Enhancing performance of triboelectric nanogenerator by filling high dielectric nanoparticles into sponge PDMS film. ACS Appl Mater Interfaces. 2016;8:736–744.
- Cheng X, Miao L, Song Y, et al. High efficiency power management and charge boosting strategy for a triboelectric nanogenerator. Nano Energy. 2017;38:438–446.
- Niu S, Liu Y, Zhou YS, et al. Optimization of triboelectric nanogenerator charging systems for efficient energy harvesting and storage. IEEE Trans Electron Devices. 2014;62:641–647.
- Cheng X, Tang W, Song Y, et al. Power management and effective energy storage of pulsed output from triboelectric nanogenerator. Nano Energy. 2019;61:517–532.
- Wang H L, Guo ZH, Zhu G, et al. Boosting the power and lowering the impedance of triboelectric nanogenerators through manipulating the permittivity for wearable energy harvesting. ACS Nano. 2021;15:7513–7521.
- Kim S, Gupta MK, Lee KY, et al. Transparent flexible graphene triboelectric nanogenerators. Adv Mater. 2014;26:3918–3925.
- Wang N, Feng Y, Zheng Y, et al. New hydrogen bonding enhanced polyvinyl alcohol based self-charged medical mask with superior charge retention and moisture resistance performances. Adv Funct Mater. 2021;31:202009172.
- Wang H, Wang J, He T, et al. Direct muscle stimulation using diode-amplified triboelectric nanogenerators (TENGs). Nano Energy. 2019;63:103844.
- Zhong W, Xu L, Zhan F, et al. Dripping channel based liquid triboelectric nanogenerators for energy harvesting and sensing. ACS Nano. 2020;14:10510–10517.
- He X, Guo H, Yue X, et al. Improving energy conversion efficiency for triboelectric nanogenerator with capacitor structure by maximizing surface charge density. Nanoscale. 2015;7:1896–1903.
- Song Y, Cheng X, Chen H, et al. Integrated self-charging power unit with flexible supercapacitor and triboelectric nanogenerator. J Mater Chem A. 2016;4:14298–14306.
- Han S A, Lee J, Lin J, et al. Piezo/triboelectric nanogenerators based on 2-dimensional layered structure materials. Nano Energy. 2019;57:680–691.
- Zhao Z, Dai Y, Liu D, et al. Rationally patterned electrode of direct-current triboelectric nanogenerators for ultrahigh effective surface charge density. Nat Commun. 2020;11:1–9.
- Kwak SS, Yoon HJ, Kim SW. Textile-based triboelectric nanogenerators for self-powered wearable electronics. Adv Funct Mater. 2019;29:1804533.
- Yi Z, Liu D, Zhou L, et al. Enhancing output performance of direct-current triboelectric nanogenerator under controlled atmosphere. Nano Energy. 2021;84:105864.
- Mule A R, Dudem B, Graham SA, et al. Humidity sustained wearable pouch-type triboelectric nanogenerator for harvesting mechanical energy from human activities. Adv Funct Mater. 2019;29:201807779.
- Wang N, Zheng Y, Feng Y, et al. Biofilm material based triboelectric nanogenerator with high output performance in 95% humidity environment. Nano Energy. 2020;77:105088.
- Xu C, Liu Y, Liu Y, et al. New inorganic coating-based triboelectric nanogenerators with anti-wear and self-healing properties for efficient wave energy harvesting. Appl Mater. Today. 2020;20:100645.
- Zhang J, Zheng Y, Xu L, et al. Oleic-acid enhanced triboelectric nanogenerator with high output performance and wear resistance. Nano Energy. 2020;69:104435.
- Zhang L, Li X, Zhang Y, et al. Regulation and influence factors of triboelectricity at the solid-liquid interface. Nano Energy. 2020;78:105370.
- Feng Y, Zheng Y, Zhang G, et al. A new protocol toward high output TENG with polyimide as charge storage layer. Nano Energy. 2017;38:467–476.
- Luo N, Feng Y, Wang D, et al. New Self-Healing triboelectric nanogenerator based on simultaneous repair friction layer and conductive layer. ACS Appl Mater Interfaces. 2020;12:30390–30398.
- Cui S, Zheng Y, Zhang T, et al. Self-powered ammonia nanosensor based on the integration of the gas sensor and triboelectric nanogenerator. Nano Energy. 2018;49:31–39.
- Li X, Zhang L, Feng Y, et al. Solid–liquid triboelectrification control and antistatic materials design based on interface wettability control. Adv Funct Mater. 2019;29:201903587.
- Cui S, Zheng Y, Liang J, et al. Triboelectrification based on double-layered polyaniline nanofibers for self-powered cathodic protection driven by wind. Nano Res. 2018;11:1873–1882.
- Xu S, Feng Y, Liu Y, et al. Gas-solid two-phase flow-driven triboelectric nanogenerator for wind-sand energy harvesting and self-powered monitoring sensor. Nano Energy. 2021;85:106023.
- Sun W, Wang N, Li J, et al. Humidity-resistant triboelectric nanogenerator and its applications in wind energy harvesting and self-powered cathodic protection. Electrochim Acta. 2021;391:138994.
- Luo N, Feng Y, Li X, et al. Manipulating electrical properties of silica-based materials via atomic oxygen irradiation. ACS Appl Mater Interfaces. 2021;13:15344–15352.
- Wang N, Zheng Y, Feng Y, et al. New starch capsules with antistatic, anti-wear and superlubricity properties. Front Mater Sci. 2021;15:266–279.
- Xu G, Zheng Y, Feng Y, et al. A triboelectric/electromagnetic hybrid generator for efficient wind energy collection and power supply for electronic devices. Science China Technol Sci. 2021;64:2003–2011.
- Li T, Dong C, Liu Y, et al. An anodized titanium/sol-gel composite coating with self-healable superhydrophobic and oleophobic property. Front Mater. 2021;8:618674.
- Feng Y, Benassi E, Zhang L, et al. Concealed wireless warning sensor based on triboelectrification and human-plant interactive induction. Research (Wash D C. 2021: 9870936.
- Liu Y, Zheng Y, Wu Z, et al. Conductive elastic sponge-based triboelectric nanogenerator (TENG) for effective random mechanical energy harvesting and ammonia sensing. Nano Energy. 2021;79:105422.
- Sun X, Feng Y, Wang B, et al. A new method for the electrostatic manipulation of droplet movement by triboelectric nanogenerator. Nano Energy. 2021;86:106115.
- Li X, Zhang L, Feng Y, et al. Reversible temperature-sensitive liquid–solid triboelectrification with polycaprolactone material for wetting monitoring and temperature sensing. Adv Funct Mater. 2021;31:202010220.
- Wen J, Chen B, Tang W, et al. Harsh-environmental-resistant triboelectric nanogenerator and its applications in autodrive safety warning. Adv Energy Mater. 2018;8:1801898.
- Jie Y, Jia X, Zou J, et al. Natural leaf made triboelectric nanogenerator for harvesting environmental mechanical energy. Adv Energy Mater. 2018;8:1703133.
- He S, Yu Z, Zhou H, et al. Polymer tubes as carrier boats of thermosetting and powder materials based on 3D printing for triboelectric nanogenerator with microstructure. Nano Energy. 2018;52:134–141.
- Parida K, Xiong J, Zhou X, et al. Progress on triboelectric nanogenerator with stretchability, self-healability and bio-compatibility. Nano Energy. 2019;59:237–257.
- Chen B, Tang W, Jiang T, et al. Three-dimensional ultraflexible triboelectric nanogenerator made by 3D printing. Nano Energy. 2018;45:380–389.
- Gao S, Chen Y, Su J, et al. Triboelectric nanogenerator powered electrochemical degradation of organic pollutant using Pt-free carbon materials. ACS Nano. 2017;11:3965–3972.
- Hajra S, Vivekananthan V, Sahu M, et al. Triboelectric nanogenerator using multiferroic materials: an approach for energy harvesting and self-powered magnetic field detection. Nano Energy. 2021;85:105964.
- Yang J-R, Lee C-J, Chang C-Y. An electrostatically self-assembled fluorinated molecule as a surface modification layer for a high-performance and stable triboelectric nanogenerator. J Mater Chem A. 2021;9:4230–4239.
- Wang S, Zi Y, Zhou YS, et al. Molecular surface functionalization to enhance the power output of triboelectric nanogenerators. J Mater Chem A. 2016;4:3728–3734.
- Song G, Kim Y, Yu S, et al. Molecularly engineered surface triboelectric nanogenerator by self-assembled monolayers (METS). Chem Mater. 2015;27:4749–4755.
- Park C, Song G, Cho SM, et al. Supramolecular-assembled nanoporous film with switchable metal salts for a triboelectric nanogenerator. Adv Funct Mater. 2017;27:1701367.
- Kim KN, Jung YK, Chun J, et al. Surface dipole enhanced instantaneous charge pair generation in triboelectric nanogenerator. Nano Energy. 2016;26:360–370.
- Jeong CK, Baek KM, Niu S, et al. Topographically-designed triboelectric nanogenerator via block copolymer self-assembly. Nano Lett. 2014;14:7031–7038.
- Dang C, Shao C, Liu H, et al. Cellulose melt processing assisted by small biomass molecule to fabricate recyclable ionogels for versatile stretchable triboelectric nanogenerators. Nano Energy. 2021: 106619.
- Liu Y, Fu Q, Mo J, et al. Chemically tailored molecular surface modification of cellulose nanofibrils for manipulating the charge density of triboelectric nanogenerators. Nano Energy. 2021;89:106369.
- Kim Y, Lee D, Seong J, et al. Ionic liquid-based molecular design for transparent, flexible, and fire-retardant triboelectric nanogenerator (TENG) for wearable energy solutions. Nano Energy. 2021;84:105925.
- Charoonsuk T, Pongampai S, Pakawanit P, et al. Achieving a highly efficient chitosan-based triboelectric nanogenerator via adding organic proteins: influence of morphology and molecular structure. Nano Energy. 2021;89:106430.
- Zhang J-H, Li Y, Du J, et al. Bio-inspired hydrophobic/cancellous/hydrophilic trimurti PVDF mat-based wearable triboelectric nanogenerator designed by self-assembly of electro-pore-creating. Nano Energy. 2019;61:486–495.
- Saadatnia Z, Esmailzadeh E, Naguib HE. High performance triboelectric nanogenerator by hot embossing on self-assembled micro-particles. Adv Eng Mater. 2019;21:1700957.
- Zhang H, Li Y, Du J, et al. A high-power wearable triboelectric nanogenerator prepared from self-assembled electrospun poly (vinylidene fluoride) fibers with a heart-like structure. J Mater Chem A. 2019;7:11724–11733.
- Wang N, Liu Y, Wu Y, et al. A β-cyclodextrin enhanced polyethylene terephthalate film with improved contact charging ability in a high humidity environment. Nanoscale Advances. 2021;3:6063–6073.
- Roy S, Ko H-U, Maji PK, et al. Large amplification of triboelectric property by allicin to develop high performance cellulosic triboelectric nanogenerator. Chem Eng J. 2020;385:123723.
- Yao C, Yin X, Yu Y, et al. Chemically functionalized natural cellulose materials for effective triboelectric nanogenerator development. Adv Funct Mater. 2017;27:1700794.
- Shang W, Gu GQ, Yang F, et al. A sliding-mode triboelectric nanogenerator with chemical group grated structure by shadow mask reactive ion etching. ACS Nano. 2017;11:8796–8803.
- Zhou Q, Pan J, Deng S, et al. Triboelectric nanogenerator-based sensor systems for chemical or biological detection. Adv Mater. 2021;33:2008276.
- Nie S, Fu Q, Lin X, et al. Enhanced performance of a cellulose nanofibrils-based triboelectric nanogenerator by tuning the surface polarizability and hydrophobicity. Chem Eng J. 2021;404:126512.
- Jiang P, Zhang L, Guo H, et al. Signal output of triboelectric nanogenerator at oil–water–solid multiphase interfaces and its application for dual-signal chemical sensing. Adv Mater. 2019;31:1902793.
- Guo H, Li T, Cao X, et al. Self-sterilized flexible single-electrode triboelectric nanogenerator for energy harvesting and dynamic force sensing. ACS Nano. 2017;11:856–864.
- Gao S, Wang M, Chen Y, et al. An advanced electro-fenton degradation system with triboelectric nanogenerator as electric supply and biomass-derived carbon materials as cathode catalyst. Nano Energy. 2018;45:21–27.
- Harnchana V, Ngoc HV, He W, et al. Enhanced power output of a triboelectric nanogenerator using poly (dimethylsiloxane) modified with graphene oxide and sodium dodecyl sulfate. ACS Appl Mater Interfaces. 2018;10:25263–25272.
- Cui X, Zhao T, Yang S, et al. A spongy electrode-brush-structured dual-mode triboelectric nanogenerator for harvesting mechanical energy and self-powered trajectory tracking. Nano Energy. 2020;78:105381.
- Zhang C, Lin X, Zhang N, et al. Chemically functionalized cellulose nanofibrils-based gear-like triboelectric nanogenerator for energy harvesting and sensing. Nano Energy. 2019;66:104126.
- Jiang Q, Chen B, Zhang K, et al. Ag nanoparticle-based triboelectric nanogenerator to scavenge wind energy for a self-charging power unit. ACS Appl Mater Interfaces. 2017;9:43716–43723.
- Wang H, Sakamoto H, Asai H, et al. An all-fibrous triboelectric nanogenerator with enhanced outputs depended on the polystyrene charge storage layer. Nano Energy. 2021;90:106515.
- Du J, Duan J, Yang X, et al. Charge boosting and storage by tailoring rhombus all-inorganic perovskite nanoarrays for robust triboelectric nanogenerators. Nano Energy. 2020;74:104845.
- Xia K, Tang H, Fu J, et al. A high strength triboelectric nanogenerator based on rigid-flexible coupling design for energy storage system. Nano Energy. 2020;67:104259.
- Pu X, Li L, Song H, et al. A self-charging power unit by integration of a textile triboelectric nanogenerator and a flexible lithium-ion battery for wearable electronics. Adv Mater. 2015;27:2472–2478.
- Xia K, Tian Y, Fu J, et al. Transparent and stretchable high-output triboelectric nanogenerator for high-efficiency self-charging energy storage systems. Nano Energy. 2021;87:106210.
- Wu H, Wang S, Wang Z, et al. Achieving ultrahigh instantaneous power density of 10 MW/m2 by leveraging the opposite-charge-enhanced transistor-like triboelectric nanogenerator (OCT-TENG). Nat Commun. 2021;12:1–8.
- Li S, Zhang D, Meng X, et al. A flexible lithium-ion battery with quasi-solid gel electrolyte for storing pulsed energy generated by triboelectric nanogenerator. Energy Storage Materials. 2018;12:17–22.
- Jin L, Xiao X, Deng W, et al. Manipulating relative permittivity for high-performance wearable triboelectric nanogenerators. Nano Lett. 2020;20:6404–6411.
- Han Y, Wang W, Zou J, et al. Self-powered energy conversion and energy storage system based on triboelectric nanogenerator. Nano Energy. 2020;76:105008.
- Fu S, He W, Tang Q, et al. Ultrarobust and high-performance rotational hydrodynamic triboelectric nanogenerator enabled by automatic mode switch and charge excitation. Adv Mater. 2021: 2105882.
- Chen G, Xu L, Zhang P, et al. Seawater degradable triboelectric nanogenerators for blue energy. Adv Mater Tech. 2020: 202000455.
- Xiong J, Cui P, Chen X, et al. Skin-touch-actuated textile-based triboelectric nanogenerator with black phosphorus for durable biomechanical energy harvesting. Nat Commun. 2018;9:4280.
- Wang N, Pu M, Ma Z, et al. Control of triboelectricity by mechanoluminescence in ZnS/Mn-containing polymer films. Nano Energy. 2021;90:106646.
- Jin Y, Xu W, Zhang H, et al. Complete prevention of contact electrification by molecular engineering. Matte. 2021;4:290–301.
- Chen H, Xu Y, Bai L, et al. Crumpled graphene triboelectric nanogenerators: smaller devices with higher output performance. Adv Mater Tech. 2017;2:201700044.
- Uzun S, Seyedin S, Stoltzfus AL, et al. Knittable and washable Multifunctional MXene-coated cellulose yarns. Adv Funct Mater. 2019;29:201905015.
- Feng P Y, Xia Z, Sun B, et al. Enhancing the performance of fabric-based triboelectric nanogenerators by structural and chemical modification. ACS Appl Mater Interfaces. 2021;13:16916–16927.
- Feng Y, Zhang L, Zheng Y, et al. Leaves based triboelectric nanogenerator (TENG) and TENG tree for wind energy harvesting. Nano Energy. 2019;55:260–268.
- Liu F, Liu Y, Lu Y, et al. Electrical analysis of triboelectric nanogenerator for high voltage applications exampled by DBD microplasma. Nano Energy. 2019;56:482–493.
- Wan J, Wang H, Miao L, et al. A flexible hybridized electromagnetic-triboelectric nanogenerator and its application for 3D trajectory sensing. Nano Energy. 2020;74:104878.
- Zhang X-S, Han M-D, Wang R-X, et al. Frequency-multiplication high-output triboelectric nanogenerator for sustainably powering biomedical microsystems. Nano Lett. 2013;13:1168–1172.
- Sun J-G, Yang TN, Kuo I-S, et al. A leaf-molded transparent triboelectric nanogenerator for smart multifunctional applications. Nano Energy. 2017;32:180–186.
- Zhang B, Zhang L, Deng W, et al. Self-powered acceleration sensor based on liquid metal triboelectric nanogenerator for vibration monitoring. ACS Nano. 2017;11:7440–7446.
- Liu W, Wang Z, Wang G, et al. Switched-capacitor-convertors based on fractal design for output power management of triboelectric nanogenerator. Nat Commun. 2020;11:1–10.
- Ma M, Kang Z, Liao Q, et al. Development, applications, and future directions of triboelectric nanogenerators. Nano Res. 2018;11:2951–2969.
- Zi Y, Guo H, Wen Z, et al. Harvesting low-frequency (< 5 Hz) irregular mechanical energy: a possible killer application of triboelectric nanogenerator. ACS Nano. 2016;10:4797–4805.
- Kim W-G, Kim D-W, Tcho I-W, et al. Triboelectric nanogenerator: structure, mechanism, and applications. ACS Nano. 2021;15:258–287.
- Zhu G, Peng B, Chen J, et al. Triboelectric nanogenerators as a new energy technology: from fundamentals, devices, to applications. Nano Energy. 2015;14:126–138.
- Ma M, Liao Q, Zhang G, et al. Self-recovering triboelectric nanogenerator as active Multifunctional sensors. Adv Funct Mater. 2015;25:6489–6494.
- Niu S, Zhou YS, Wang S, et al. Simulation method for optimizing the performance of an integrated triboelectric nanogenerator energy harvesting system. Nano Energy. 2014;8:150–156.
- Khandelwal G, Raj NPMJ, Kim S-J. Triboelectric nanogenerator for healthcare and biomedical applications. Nano Today. 2020;33:100882.
- Rasel M S, Maharjan P, Salauddin M, et al. An impedance tunable and highly efficient triboelectric nanogenerator for large-scale, ultra-sensitive pressure sensing applications. Nano Energy. 2018;49:603–613.
- Liu W, Wang Z, Wang G, et al. Integrated charge excitation triboelectric nanogenerator. Nat Commun. 2019;10:1–9.
- Bera B. Literature review on triboelectric nanogenerator. Imperial J Interdiscipl Res (IJIR). 2016; 2: 1263-1271.
- Qiu C, Wu F, Shi Q, et al. Sensors and control interface methods based on triboelectric nanogenerator in IoT applications. IEEE Access. 2019;7:92745–92757.
- Jiang B, Long Y, Pu X, et al. A stretchable, harsh condition-resistant and ambient-stable hydrogel and its applications in triboelectric nanogenerator. Nano Energy. 2021;86:106086.
- Wang Y, Yang Y, Wang ZL. Triboelectric nanogenerators as flexible power sources. Flexible Electron. 2017;1:1–10.
- Wang S, Niu S, Yang J, et al. Quantitative measurements of vibration amplitude using a contact-mode freestanding triboelectric nanogenerator. ACS Nano. 2014;8:12004–12013.
- Xu S, Zhang L, Ding W, et al. Self-doubled-rectification of triboelectric nanogenerator. Nano Energy. 2019;66:104165.
- Chen X, Xie X, Liu Y, et al. Advances in healthcare electronics enabled by triboelectric nanogenerators. Adv Funct Mater. 2020;30:2004673.
- Zhang S, Bick M, Xiao X, et al. Leveraging triboelectric nanogenerators for bioengineering. Matter. 2021;4:845–887.
- Xia X, Liu Q, Zhu Y, et al. Recent advances of triboelectric nanogenerator based applications in biomedical systems. EcoMat. 2020;2:e12049.
- Zhang B, Chen J, Jin L, et al. Rotating-disk-based hybridized electromagnetic–triboelectric nanogenerator for sustainably powering wireless traffic volume sensors. ACS Nano. 2016;10:6241–6247.
- Jao Y-T, Yang P-K, Chiu C-M, et al. A textile-based triboelectric nanogenerator with humidity-resistant output characteristic and its applications in self-powered healthcare sensors. Nano Energy. 2018;50:513–520.
- Yu A, Pu X, Wen R, et al. Core–shell-yarn-based triboelectric nanogenerator textiles as power cloths. ACS Nano. 2017;11:12764–12771.
- Lee K Y, Chun J, Lee J H, et al. Hydrophobic sponge structure-based triboelectric nanogenerator. Adv Mater. 2014;26:5037–5042.
- Xing F, Jie Y, Cao X, et al. Natural triboelectric nanogenerator based on soles for harvesting low-frequency walking energy. Nano Energy. 2017;42:138–142.
- Lee W, Lee J. Development of a compact thermoelectric generator consisting of printed circuit heat exchangers. Energy Convers Manage. 2018;171:1302–1310.
- Lim K-W, Peddigari M, Park CH, et al. A high output magneto-mechano-triboelectric generator enabled by accelerated water-soluble nano-bullets for powering a wireless indoor positioning system. Energy Environ Sci. 2019;12:666–674.
- Choi J, Jung Y, Dun C, et al. High-performance, wearable thermoelectric generator based on a highly aligned carbon nanotube sheet. ACS Appl Energy Mater. 2019;3:1199–1206.
- Kwak M S, Lim K W, Lee HY, et al. Multiscale surface modified magneto-mechano-triboelectric nanogenerator enabled by eco-friendly NaCl imprinting stamp for self-powered IoT applications. Nanoscale. 2021;13:8418–8424.
- Lee HE, Park JH, Jang D, et al. Optogenetic brain neuromodulation by stray magnetic field via flash-enhanced magneto-mechano-triboelectric nanogenerator. Nano Energy. 2020;75:104951.
- Kim S-W, Yang UJ, Lee JW, et al. Triboelectric charge-driven enhancement of the output voltage of BiSbTe-based thermoelectric generators. ACS Energy Lett. 2021;6:1095–1103.