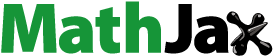
Abstract
We found boron doping can substantially reduce the ductility-loss in the CrCoNi medium-entropy alloy after gas-hydrogen charging, from ∼71% to ∼46%, while the fracture mode transfers from predominantly intergranular to ductile transgranular dominated. The two alloys have no difference in phase-structures (single face-center-cubic), grain sizes, and grain-boundary (GB) characters. However, atom probe tomography identified apparent GB decoration of boron up to 1.5 at.% and nanometer-scaled scattered borides in boron-doped CrCoNi. Such local chemical difference leads to enhanced GB cohesion and reduced hydrogen diffusivity along GBs, resulting in improved immunity against hydrogen-embrittlement and suppressed mechanical degradation in the boron-doped CrCoNi alloy.
GRAPHICAL ABSTRACT
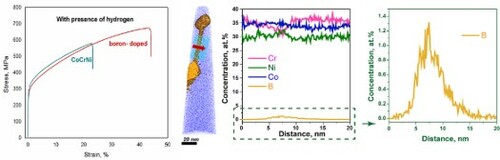
IMPACT STATEMENT
We found grain-boundary decoration of boron can substantially enhanced resistance to hydrogen embrittlement in a CrCoNi-based medium-entropy alloy when exposing to high-pressure gas-hydrogen charging.
1. Introduction
Hydrogen has long been recognized as a clean and mobile energy carrier, potential to be an alternative to fossil fuels. However, hydrogen is known to markedly damage the ductility, fracture strength, fracture toughness, and/or fatigue durability of most metallic materials. This effect is known as hydrogen embrittlement (HE) [Citation1,Citation2] and has hindered the advancement of hydrogen-based community. Recently, a new philosophy of alloy design has emerged, namely high-entropy alloys (HEAs) [Citation3–5] or compositional-complex alloys [Citation6,Citation7], which are likely to pave a new way to develop hydrogen-bearing alloys with economic efficiency due to their outstanding mechanical performance and structural stability. Relative studies show that face-centered-cubic (fcc) HEAs are highly resistant to HE [Citation8–14]. For instance, the CrMnFeCoNi HEA exhibits a negligible ductility loss of ∼5% after gas-hydrogen charging (300°C, 15 MPa, 72 h), which is much lower than that of ∼61% for 304 and of ∼27% for 316 L austenitic steels [Citation10]. More interestingly, after reduction or removal of Mn from the CrMnFeCoNi HEA, the resulted (CrFeCoNi)87Mn13 [Citation13] and CrFeCoNi [Citation11] alloys exhibit even better HE resistance. This suggests that the chemistry of HEAs strongly influences the grain boundary (GB) cohesive strength, which is essential for resistance to hydrogen-induced boundary cracking and associated mechanical degradation.
Compared to the above CrMnFeCoNi and CrFeCoNi HEAs, the equiatomic prototype CrCoNi medium-entropy alloy (MEA) shows superior mechanical properties in atmospheric environment [Citation5], but to date studies on its resistance to HE remain very limited. Soundararajan et al. [Citation15] reported that it has good resistance to HE in the case of electrochemical hydrogen charging, but it is unknown for the case of high-pressure hydrogen gas-charging, which is more critical for practical situations. In this study, we firstly investigate the mechanical properties of the ternary CrCoNi MEA after gas-charging of hydrogen at 300°C under 18 MPa for 336 hs, resulting in a hydrogen concentration of 28 wt. ppm. Unfortunately, we found a catastrophic ductility loss of up to ∼71% and a hydrogen-induced intergranular fracture. Inspiring from previous studies on Ni3Al where moisture-induced hydrogen-based boundary embrittlement can be suppressed by adding small amounts of boron [Citation16], we designed a boron-doped (400 at. ppm) CrCoNi MEA to overcome hydrogen-induced intergranular brittleness by controllable segregation of boron at GBs. The phase components, GB chemistries and characters, as well as tensile properties and fracture mechanisms with and without hydrogen presence were thoroughly investigated.
2. Experimental methods
The equiatomic CrCoNi and boron-doped (400 at. ppm or 80 wt. ppm) CrCoNi alloys were prepared by arc melting under high-purity argon atmosphere, and then casted into a copper mold with dimensions of 55 × 10 × 10 mm3. All the cast ingots were homogenized at 1200°C for 2 hs, then cold rolled with 73% thickness reduction, and subsequently recrystallized at 1200°C, followed by water quenching. The CrCoNi and boron-doped CrCoNi alloys were annealed for 2 and 2.5 mins, respectively, to obtain similar grain sizes. Detailed information about grain sizes and GB characters were examined by electron backscatter diffraction (EBSD) measurements operated at 20 kV. Specimens for EBSD measurements were mechanically polished, followed by electrochemical polishing with a methanol solution containing 25% nitric acid. Tensile samples were prepared along the rolling direction using electrical discharge machining, with gauge dimensions of 12.5 × 3.25 × 1.5 mm3. All tensile samples were initially ground with fine SiC papers up to 2000# before being gassed with hydrogen at 300°C under a constant pressure of 18 MPa for 336 hs. This charging temperature was selectively chosen to promote hydrogen diffusivity and meanwhile to preclude any phase transformation in the boron-doped and undoped CrCoNi alloys. After hydrogen-charging, all the samples were immediately stored in liquid nitrogen to retain the hydrogen until further experiments as tensile tests or/and hydrogen concentration detections. The hydrogen concentration was measured by a hydrogen nitrogen oxygen meter. Uniaxial tensile tests were conducted at room temperature operating at a strain rate of 5 × 10−5 s−1. This relatively low displacement rate was employed here to allow enough time for diffusion-controlled hydrogen redistribution to fracture sites, and to precisely evaluate the hydrogen embrittlement effect [Citation17]. Two tests were performed for each sample for the repeatable and reliable of data. The fracture surfaces were examined by using Zeiss scanning electronic microscope. Site-specific specimens containing a GB for three-dimensional atom probe tomography (3D-APT) observations were prepared by a dual-beam scanning electronic microscope/focused-ion-beam (SEM-FIB) instrument (FEI Helios Nanolab 600i) equipped with the transmission Kikuchi diffraction (TKD) technique. Subsequently APT measurements were performed in a Cameca Instrument Local Electrode Atom Probe (LEAP) 5000 XR operated at a specimen setpoint temperature of 60 K and a laser pulse energy of 30 pJ at a pulse repetition rate of 200 kHz for a detection rate of 0.5% atoms per pulse. Notice for the identification of B atoms during the APT reconstruction process [Citation18], we selected four peaks where Da = 5, 5.5, 10 and 11 in the mass spectrum that cover both B++ and B+ ions, as exampled in Appendix A4.
3. Results and discussion
Figure a presented the engineering strain–stress curves of the CrCoNi and boron-doped CrCoNi alloys with and without the presence of hydrogen. Before hydrogen-charging, the yield strength, fracture strength, and elongation to failure of CrCoNi is 250.0, 727.4 MPa and 78.4%, respectively. After 18 MPa hydrogen-charging, the yield strength increased slightly to 292 MPa, but the tensile -strength and -elongation noticeably decreased to 579.0 MPa and 22.8%, respectively. From the uniaxial tensile test results, it is apparent that hydrogen induced a severe ductility loss, which reaches as high as ∼70.9%. While for the boron-doped alloy, the yield strength, fracture strength, and elongation to failure is 241.5, 717.6 MPa and 81.3% in the uncharged state. After 18 MPa hydrogen-charging, the yield strength and tensile strength increased to 275.6 and 673.4 MPa, respectively, whereas elongation to failure decreased to 44.1%. The hydrogen-induced ductility loss in the boron-doped alloy was measured to be 45.8%, which is approximate half of that (70.9 %) in the undoped alloy, indicating a much lower susceptibility to HE. It should be noted that the hydrogen concentrations in the boron-doped and undoped CrCoNi alloys were measured at similar levels of 27 and 28 wt. ppm, respectively, which precludes effects of hydrogen concentration on their distinctive HE resistance.
Figure 1. (a) Engineering strain-stress curves of the CrCoNi and boron-doped CrCoNi alloys in the hydrogen- uncharged and charged states. Fractured surfaces of (b and d) CrCoNi and (c and e) boron-doped CrCoNi with and without presence of hydrogen. Insets of Figure d and e are corresponding high-resolution fractographs from selected intergranular facets.
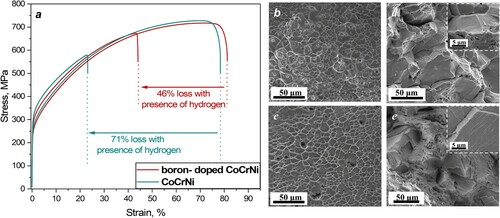
Figures b–e compare the typical fractographies of the undoped and boron-doped CrCoNi alloys with and without the presence of hydrogen. As shown in Figure b,c, both the boron-doped and undoped CrCoNi alloys exhibit ductile transgranular failure of microvoid coalescence in the uncharged state, which is consistent with previous studies [Citation19]. After 18 MPa hydrogen-charging, however, their failure modes changed completely and differed significantly. More specifically, the CrCoNi alloy transferred to an intergranular dominated failure mode only with traces of ductile features (Figure d). Inspection of faceted surfaces at high magnification readily identified evidence of slip traces on individual facets (see inset of Figure d), which are common features on a hydrogen-induced intergranular facet [Citation20,Citation21]. Secondary cracks formed between faceted regions, while ductile fracture features were also observed on ridges between intergranular facets. These interesting observations possibly suggest variant resistance to HE in different GB types. The boron-doped CrCoNi alloy, on the other hand, surprisingly shows a ductile transgranular dominated failure mode even in the hydrogen charged state. This suggests that the hydrogen-induced intergranular fracture observed in the hydrogen-charged CrCoNi alloy was greatly suppressed by boron doping at the ppm level, despite few intergranular fractured facets probed in Figure e. Actually, these facets are completely different from those in the CrCoNi alloy, as they are not so ‘flat’ and have more slip traces (see inset of Figure e), evidencing the enhanced ductility. In addition, with charging of hydrogen, the pronounced necking behavior almost disappeared in both alloys (see Appendix A1), with rupture taking place immediately after reaching the ultimate tensile strength [Citation19]. Besides, we should note that Soundararajan et al. [Citation15] has studied the hydrogen resistance behavior of the CrCoNi MEA by electrochemical hydrogen charging, and it exhibits good immunity to HE. The different responses to HE were ascribed to the different hydrogen distribution caused by different hydrogen charging methods, i.e. gaseous hydrogen charging vs. electrochemical hydrogen charging. The former guarantees a homogeneous and saturated hydrogen distribution in the boron -doped and -undoped CoCrNi alloys. For the later, however, most hydrogen is concentrated IN the surface, and the interior is still free of hydrogen. Therefore, they only observed hydrogen-induced intergranular brittle fracture nearby the surfaces and the interior region still dominated by ductile fracture, which suppressed the mechanical property degradation in the electrochemical hydrogen-charged CoCrNi alloy.
The main goal of the present work is to suppress hydrogen-induced GB embrittlement and associated mechanical degradation in the CrCoNi MEA. The reduction in ductility loss as well as the difference in the fracture surface morphologies evidently suggest that boron doping has greatly enhanced the resistance to hydrogen-induced boundary cracking, which is significantly affected by intrinsic GB cohesive strength. The failure mode transitions from ductile transgranular fracture to brittle intergranular fracture with the presence of hydrogen have been previously reported in Ni [Citation21], Ni-based alloys [Citation1], and Fe [Citation22,Citation23], known as hydrogen-induced GB embrittlement. The transition in failure mode could be explained by hydrogen segregating to, and accumulating at GBs through dislocation transport and stress-induced diffusion, which reduces the cohesive strength of GBs. Consequently, crack initiation and propagation along the boundary was favored over ductile failure processes, leading to brittle intergranular fracture. Accordingly, it is not hard to draw a conclusion that the GB cohesive strength is a key factor to control the resistance to HE in metallic materials, which can indeed be tuned by GB characters and chemistries (including GB phases). We first use EBSD measurements to examine the boron-doped and -undoped CrCoNi alloys’ detailed microstructures, including grain sizes and GB characters, as presented in Figure . Both alloys have fully recrystallized single-phase microstructures with the fcc structure. Because of the relative shorter annealing time at 1200°C, the CrCoNi alloy has an averaged grain size of 19.8 µm, which is a little smaller than 24.1 µm of the boron-doped alloy. See the detailed grain size distribution in Appendix A2. It have been reported that grain refinement can increase resistance to HE [Citation13,Citation24], but the larger-grained boron-doped CrCoNi alloy has stronger resistance to HE and there should be other cause for the improved performance. We further analyzed their GB characters, since random high-angle GBs were suggested to be more vulnerable to hydrogen-induced boundary cracking than special-angle GBs. As presented in Figure a,b, a large number of twin boundaries and high-angle GBs were readily identified. Quantitative analysis in Figure c clearly shows that the two alloys have a similar number density of twin boundaries and high-angle GBs. This suggests that boron alloying did not alter GB characters and thus they are not responsible for the improved resistance to HE. We further examined the deformed microstructures of the hydrogen-charged CrCoNi and boron-doped CrCoNi alloys after tensile fracture. As shown in Figure d,e, both alloys were dominated by dislocations, deformation twins, and stacking faults, which have no distinctive difference with the uncharged alloys [Citation19]. Such results indicate that the deformation mechanism was not significantly affected by hydrogen.
Figure 2. EBSD measurements on grain sizes and GB characters of the fully recrystallized (a) CrCoNi and (b) boron-doped CrCoNi alloys as well as (c) their comparison on GB characters. The deformed microstructures of the hydrogen-charged (d) CrCoNi and (e) boron-doped CrCoNi alloys after fracture.
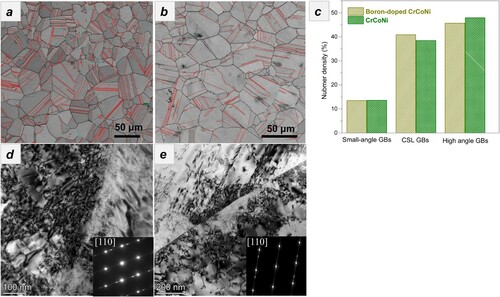
In the following, we investigated the boundary chemistry using 3D-APT, which was suitable for performing quantitative chemical analyses across the GBs. The APT tips with a high-angle 20.5° GB were prepared using the site-specific FIB technique (see Appendix A3) [Citation25]. The GB misorientation was determined by the TKD orientation map (see Appendix A3). Two tip samples were field-evaporated and then reconstructed following the stand protocol [Citation26]. Figures a,b present the elemental distribution maps, where the B atoms are colored in yellow. The two figures show clear segregation of boron and precipitation of borides along the boundary. To quantitatively analyze the degree of segregation, we further outlined the GB by 0.4 at.% B and 1.0 at.% B iso-compositional surfaces (Figure a,c), respectively. One-dimensional (1D) composition analysis profiles across the GB, as indicated by a red arrow in Figure a, reveal segregations of boron up to 1.5 at.% (Figure b), which is 37.5 times as much as that of the matrix (0.04 at.%). Besides, Ni and Co atoms are randomly distributed across the boundary but a slight depletion of Cr was observed (marked by a red arrow in Figure b), with a Cr concentration around 31 at.%, compared to 37.5 at.% in the matrix. Precipitates of borides with a size being 20–50 nm were also identified along the boundary. 1D-composition analysis profiles across borides shown in Figure d reveal that they are Cr-enriched and Co- and Ni-depleted, resulting in a chemical composition of Cr57-B34-Co7-Ni2 (at.%), the stoichiometry of which corresponds to Cr5B3. See the mass spectrum from the region of the CrB precipitate in Appendix A4. The depletion of Cr along the boundary may be due to the precipitation of Cr-enriched borides. From the perspective of mixing enthalpy of B with the constituent elements of Cr, Co, and Ni, Cr-B (−61 kJ/mol) is much smaller than Co-B (−42 kJ/mol) and Ni-B (−39 kJ/mol) [Citation27], and thus it combines preferentially with Cr to form borides. Based on these results, we know that Cr, Co, and Ni atoms are randomly distributed in the undoped CrCoNi MEA, but ppm level boron doping resulted in boron decorated GBs, likely leading to enhanced resistance to HE in the boron-doped CrCoNi MEA.
Figure 3. (a and b) Two atom probe reconstructions of the boron-doped (400 at. ppm) CrCoNi alloy from the specific region containing a high-angel GB being 20.5°.
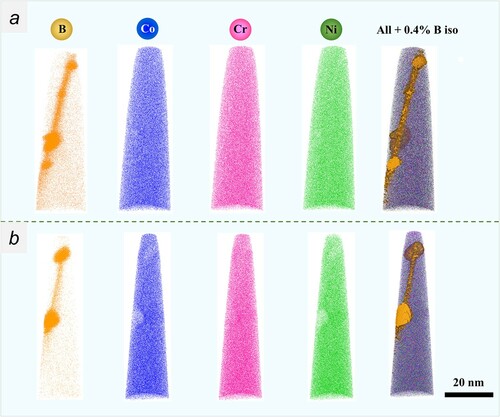
Figure 4. (a and c) Detailed atom probe reconstruction of the boron-doped (400 at. ppm) CrCoNi alloy using 0.4% B iso outlining the GB and 1.0% B iso outlining borides along the GB. 1D composition profiles across (b) a GB and (d) three borides.
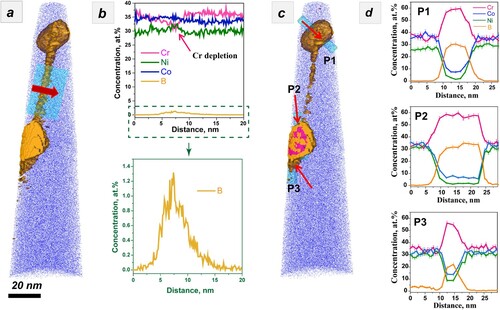
As noted above, the key finding in this work is that GB decoration of boron can greatly suppress hydrogen-induced GB embrittlement and thereby optimize the mechanical properties of the CrCoNi alloy when exposed to hydrogen. The beneficial effect of boron possibly comes from two aspects: a reduction of hydrogen diffusivity along GBs and an enhancement of boundary-cohesive strength. Firstly, adoption of 400 at. ppm boron hardly changes the fcc lattice in the CrCoNi alloy, so that the diffusivities of hydrogen in the grain interior remain constant. Diffusivities of hydrogen along GBs, however, can be greatly suppressed. It has shown in Ni3Al that an increased boron concentration from 100 to 1000 wt. ppm induces a substantial increase in the level of boron segregated to GBs [Citation28], which significantly reduces the diffusion of hydrogen along GBs at room temperature. Specifically, the apparent diffusion coefficient of hydrogen in the Ni3Al (23 at.% Al) alloy doped with 1000 wt. ppm boron is less than one-twentieth that of the Ni3Al (21 at.% Al) alloy doped with 120 wt. ppm boron [Citation29]. It has been suggested that segregations of boron may fill vacancies at GBs [Citation30], and thus decreases hydrogen diffusivity along GBs, which hindered hydrogen accumulation at GBs. On the other hand, it has been suggested theoretically and shown experimentally that segregation of boron at GBs changes the local atom bonding and enhanced the boundary-cohesive strength of materials. For instance, first-principles calculations on iron boundary-cohesion strength show that boron is a GB cohesion enhancer [Citation31]. This study demonstrates that iron–boron hybridization permits covalent bonding normal to the boundary, which contributes to the cohesion enhancement of (111) [Citation31]. A more recent study on the iron Σ5(310) symmetrical tilt GB suggests that boron occupations of GBs decreased the boundary energy and caused the boundary strengthening, which effectively inhibits hydrogen-induced GB embrittlement [Citation32]. Similar theoretical calculations were also conducted on a ∑5(210)
nickel GB, which shows that boron does not draw charge from nickel atoms but rather form covalent bonds with Ni, these covalent Ni-B bonds building boron as a GB cohesion enhancer [Citation33]. Besides these theoretical calculations, extensive experimental results demonstrated that boron is a GB strengthener. For instance, Jung et al. [Citation34] shows that adding minor boron to Ni prevents hydrogen-related intergranular failure, and Wan et al. [Citation35] demonstrated that the hydrogen-induced ductility loss in an ordered Fe3Ni compound decreases from 75% to 14% after a minor boron alloying.
It should be noted that segregation of boron at GBs in the boron-doped CrCoNi alloy caused the precipitation of a few borides, which are incoherent with matrix. These incoherent interfaces are strong hydrogen trapping sites and may trigger cracks as a result of hydrogen accumulation. Fortunately, these ellipsoidal borides are isolated. Even cracks formed at these interfaces, they could be prevented from propagating without causing fatal damage. Based on these facts, it is reasonable for us to speculate that in the boron-doped CrCoNi MEA, the segregation of boron to GBs substantially enhanced the boundary-cohesive strength and reduced hydrogen diffusivity along the GBs, which together improves the resistance to HE.
4. Conclusions
In summary, the present work explores mechanical properties and failure modes of the classic CrCoNi MEA before and after 18 MPa-hydrogen charging. We found that hydrogen induced a failure mode transition from ductile transgranular to brittle intergranular and severe mechanical degradation. In contrast, minor doping with boron (400 at. ppm) reduced the hydrogen-induced ductility loss from ∼71% to ∼46% and simultaneously suppressed hydrogen-induced intergranular failure. Microstructural examinations reveal two alloys have no major microstructural differences in phase constitutions, grain sizes, and GB characters. However, apparent boron segregation to GBs was readily identified by 3D-APT. The GB decoration of boron was suggested to significantly enhance the cohesive strength of GBs and simultaneously reduced hydrogen diffusivity along GBs, resulting in the improved resistance to hydrogen-induced intergranular fracture in the boron-doped CrCoNi alloy.
Acknowledgements
We thank Dr. Baptiste Gault for the initial discussion. U. Tezins, C. Broß and A. Sturm are acknowledged for their technical support of the FIB & APT facilities at MPIE.
Disclosure statement
No potential conflict of interest was reported by the author(s).
Additional information
Funding
References
- Kimura A, Birnbaum H. Hydrogen induced grain boundary fracture in high purity nickel and its alloys—enhanced hydrogen diffusion along grain boundaries. Acta Metall. 1988;36(3):757–766.
- Lassila D, Birnbaum H. Intergranular fracture of nickel: the effect of hydrogen-sulfur co-segregation. Acta Metall. 1987;35(7):1815–1822.
- Yeh JW, Chen SK, Lin SJ, et al. Nanostructured high-entropy alloys with multiple principal elements: novel alloy design concepts and outcomes. Adv Eng Mater. 2004;6(5):299–303.
- George EP, Raabe D, Ritchie RO. High-entropy alloys. Nat Rev Mater. 2019;4(8):515–534.
- Gludovatz B, Hohenwarter A, Catoor D, et al. A fracture-resistant high-entropy alloy for cryogenic applications. Science. 2014;345(6201):1153–1158.
- Cantor B, Chang I, Knight P, et al. Microstructural development in equiatomic multicomponent alloys. Mater Sci Eng A. 2004;375:213–218.
- Seol JB, Bae JW, Li Z, et al. Boron doped ultrastrong and ductile high-entropy alloys. Acta Mater. 2018;151:366–376.
- Nygren KE, Bertsch KM, Wang S, et al. Hydrogen embrittlement in compositionally complex FeNiCoCrMn FCC solid solution alloy. Curr Opin Solid State Mater Sci. 2018;22(1):1–7.
- Pu Z, Chen Y, Dai LH. Strong resistance to hydrogen embrittlement of high-entropy alloy. Mater Sci Eng A. 2018;736:156–166.
- Zhao Y, Lee D-H, Seok M-Y, et al. Resistance of CoCrFeMnNi high-entropy alloy to gaseous hydrogen embrittlement. Scr Mater. 2017;135:54–58.
- Nygren KE, Wang S, Bertsch KM, et al. Hydrogen embrittlement of the equi-molar FeNiCoCr alloy. Acta Mater. 2018;157:218–227.
- Luo H, Li Z, Raabe D. Hydrogen enhances strength and ductility of an equiatomic high-entropy alloy. Sci Rep. 2017 Aug 29;7(1):9892.
- Koyama M, Wang H, Verma VK, et al. Effects of Mn content and grain size on hydrogen embrittlement susceptibility of face-centered cubic high-entropy alloys. Metall Mater Trans A. 2020;51(11):5612–5616.
- Ichii K, Koyama M, Tasan CC, et al. Localized plasticity and associated cracking in stable and metastable high-entropy alloys pre-charged with hydrogen. Procedia Struct Integ. 2018;13:716–721.
- Soundararajan CK, Luo H, Raabe D, et al. Hydrogen resistance of a 1 GPa strong equiatomic CoCrNi medium entropy alloy. Corros Sci. 2020;167:108510.
- Liu CT, White C, Horton J. Effect of boron on grain-boundaries in Ni3Al. Acta Metall. 1985;33(2):213–229.
- Boniszewski T, Smith G. The influence of hydrogen on the plastic deformation ductility, and fracture of nickel in tension. Acta Metall. 1963;11(3):165–178.
- Seol JB, Gu GH, Lim NS, et al. Atomic scale investigation on the distribution of boron in medium carbon steels by atom probe tomography and EELS. Ultramicroscopy. 2010;110(7):783–788.
- Shi Y, Wang Y-D, Li S, et al. Mechanical behavior in boron-microalloyed CoCrNi medium-entropy alloy studied by in situ high-energy X-ray diffraction. Mater Sci Eng A. 2020;788:139600.
- Bechtle S, Kumar M, Somerday BP, et al. Grain-boundary engineering markedly reduces susceptibility to intergranular hydrogen embrittlement in metallic materials. Acta Mater. 2009;57(14):4148–4157.
- Martin M, Somerday B, Ritchie R, et al. Hydrogen-induced intergranular failure in nickel revisited. Acta Mater. 2012;60(6–7):2739–2745.
- Kimura A, Kimura H. Effect of carbon on the hydrogen induced grain boundary fracture in iron. Nippon Kinzoku Gakkaishi (1952). 1983;47(10):807–813.
- Shin K, Meshii M. Effect of sulfur segregation and hydrogen charging on intergranular fracture of iron. Acta Metall. 1983;31(10):1559–1566.
- Koyama M, Ichii K, Tsuzaki K. Grain refinement effect on hydrogen embrittlement resistance of an equiatomic CoCrFeMnNi high-entropy alloy. Int J Hydrogen Energy. 2019;44(31):17163–17167.
- Thompson K, Lawrence D, Larson D, et al. In situ site-specific specimen preparation for atom probe tomography. Ultramicroscopy. 2007;107(2-3):131–139.
- Gault B, Moody MP, De Geuser F, et al. Advances in the calibration of atom probe tomographic reconstruction. J Appl Phys. 2009;105(3):034913.
- Takeuchi A, Inoue A. Classification of bulk metallic glasses by atomic size difference, heat of mixing and period of constituent elements and its application to characterization of the main alloying element. Mater Trans. 2005;46(12):2817–2829.
- Choudhury A, White C, Brooks C. The intergranular segregation of boron in Ni3Al: equilibrium segregation and segregation kinetics. Acta Metall Mater. 1992;40(1):57–68.
- Swart PJ, Snoeij P. Hydrogen diffusivity in boron-doped polycrystalline Ni3Al. IEEE Trans Geosci Remote Sens. 1994;32(2):296–306.
- Kontis P, Yusof HM, Pedrazzini S, et al. On the effect of boron on grain boundary character in a new polycrystalline superalloy. Acta Mater. 2016;103:688–699.
- Wu R, Freeman AJ, Olson GB. First principles determination of the effects of phosphorus and boron on iron grain boundary cohesion. Science. 1994;265(5170):376–380.
- Kulkov SS, Bakulin AV, Kulkova SE. Effect of boron on the hydrogen-induced grain boundary embrittlement in α-Fe. Int J Hydrogen Energy. 2018;43(3):1909–1925.
- Geng W, Freeman AJ, Wu R, et al. Embrittling and strengthening effects of hydrogen, boron, and phosphorus on a Σ5 nickel grain boundary. Phys Rev B. 1999;60(10):7149.
- Jung CB, Lee KS. The effects of the addition of B and Fe and a prior deformation on the hydrogen embrittlement of nickel. Scr Mater. 1996;35(2):267–271.
- Wan X, Chen Y, Shi D, et al. Effect of alloy stoichiometry and boron doping on the H2-induced environmental embrittlement of Ni3Fe intermetallics. Intermetallics. 2008;16(4):550–553.
Appendices
Figure A1. Overall fracture surfaces of the (a and b) CrCoNi and (c and d) boron-doped CrCoNi alloys with and without the presence of hydrogen.
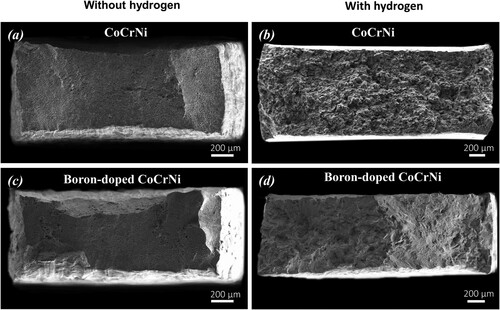