ABSTRACT
There is emerging evidence that glial-derived neurotrophic factor (GDNF) is a potent inducer of restrictive barrier function in tight junction-forming microvascular endothelium and epithelium, including the human blood-nerve barrier (BNB) in vitro. We sought to determine the role of GDNF in restoring BNB function in vivo by evaluating sciatic nerve horseradish peroxidase (HRP) permeability in tamoxifen-inducible GDNF conditional knockout (CKO) adult mice following non-transecting crush injury via electron microscopy, with appropriate wildtype (WT) and heterozygous (HET) littermate controls. A total of 24 age-, genotype- and sex-matched mice >12 weeks of age were injected with 30 mg/kg HRP via tail vein injection 7 or 14 days following unilateral sciatic nerve crush, and both sciatic nerves were harvested 30 minutes later for morphometric assessment by light and electron microscopy. The number and percentage of HRP-permeable endoneurial microvessels were ascertained to determine the effect of GDNF in restoring barrier function in vivo. Following sciatic nerve crush, there was significant upregulation in GDNF protein expression in WT and HET mice that was abrogated in CKO mice. GDNF significantly restored sciatic nerve BNB HRP impermeability to near normal levels by day 7, with complete restoration seen by day 14 in WT and HET mice. A significant recovery lag was observed in CKO mice. This effect was independent on VE-Cadherin or claudin-5 expression on endoneurial microvessels. These results imply an important role of GDNF in restoring restrictive BNB function in vivo, suggesting a potential strategy to re-establish the restrictive endoneurial microenvironment following traumatic peripheral neuropathies.
Introduction
Peripheral neuropathies are a very common group of disorders characterized by axonal degeneration, demyelination or both in peripheral nerves. Over 20 million Americans are affected by peripheral neuropathies (www.neuropathy.org), with >100 million patients affected worldwide. The prevalence of peripheral neuropathy is increasing. Peripheral neuropathies can be acquired or inherited. Acquired causes are commonly due to trauma, metabolic derangements, endocrine disorders (such as diabetes mellitus, the most common cause of peripheral neuropathy worldwide), infections (such as leprosy and human immunodeficiency virus), drugs and toxins, nutritional deficiencies, cancer, primary or secondary autoimmune inflammatory disorders (e.g. Guillain-Barré syndrome), to name a few.
The total annual cost of diabetic peripheral neuropathy and its complications in the United States of America (U.S.A.) alone was estimated to be ∼$66.2 billion in 2012, with significantly increased total direct costs in patients with peripheral neuropathy compared to patients without neuropathy.Citation1,Citation2 A common consequence of peripheral neuropathies is chronic neuropathic pain, a condition that is estimated to affect about 1–10% of the general population worldwide.Citation3-Citation7 Diabetic patients with severe peripheral neuropathy had ∼5-fold higher direct medical costs in the U.S.A. compared to unaffected diabetics.Citation2 Peripheral neuropathy and its consequential chronic neuropathic pain cause significant reduction in productivity and burden on health care resources worldwide.Citation6,Citation8-Citation10 Current medical management of peripheral neuropathies and neuropathic pain aims to identify and specifically treat its cause. However, most treatments are non-specific and are partly efficacious.Citation11,Citation12
Severe traumatic peripheral neuropathies may occur in up to 2.8% of polytrauma patients,Citation13 and are commonly associated with residual motor and sensory deficits, chronic neuropathic pain and disability despite significant microsurgical advances (neurorrhapy, auto- or allograft use and application of nerve conduits), which are primarily geared towards reconnecting the connective tissue layers in peripheral nerves to provide a permissive scaffold for growth, and strategies geared towards enhancing axonal regeneration.Citation14,Citation15 Schwann cells are unique glial cells restricted to the endoneurium of peripheral nerves. Schwann cells secrete GDNF in vitro and in vivo, with higher levels expressed during peripheral nerve injury.Citation16-Citation26 GDNF is structurally related to transforming growth factor β-1 and supports the survival of peripheral autonomic and sensory, spinal cord motor and midbrain dopaminergic neurons.Citation17,Citation21,Citation27-Citation29 GDNF typically signals via its receptor GFRα1 complexed with RET-Tyrosine kinase, and utilizes Ras-mitogen activated protein kinase (MAPK), phosphatidylinositol 3-kinase (PI3-K)/AKT, p38 MAPK and c-Jun N-terminal kinase signaling pathways for its biological effects.Citation29-Citation34 GDNF has been shown to promote mammalian peripheral nerve or nerve root axonal regeneration after injury in vivo,Citation20,Citation22,Citation35,Citation36 with decline in expression associated with impaired regeneration following chronic Schwann cell denervation.Citation37
Peripheral nerves are vascularized by extrinsic nutrient arteries that form the vasa nervorum from which epineurial macrovessels and perineurial and endoneurial microvessels are derived. These vessels form anastomotic plexi, with tight junction specialization restricted to the endoneurial endothelial cells. These cells lack fenestrations and form the blood-nerve barrier (BNB), as they are in direct contact with circulating blood (unlike innermost layer perineurial myofibroblasts that also form tight junctions but separate the endoneurial and epineurial interstitial fluid compartments).Citation38-Citation41 Although the importance of viable revascularization for achieving functional recovery following surgical repair of traumatic peripheral neuropathy has been suggested,Citation14,Citation15 incorporation of tissue-specific revascularization paradigms in peripheral nerve repair procedures is lacking. It is important to recognize that there is phenotypic and functional heterogeneity between macrovascular and microvascular endothelial cells from the same tissue, and between endothelial cells from different tissues and species, with selective adaptation occurring depending on the tissue microenvironment.Citation42-Citation45
Primary human endoneurial endothelial cells that form the BNB express GFRα1 in vitro and in situ.Citation46-Citation49 Our prior work demonstrated that GDNF is a sufficient paracrine regulator of the human BNB in vitro following diffuse injury mediated by serum withdrawal (which causes endothelial cell detachment in vitro). GDNF (in a dose-dependent manner) restored human BNB biophysical properties dependent on RET-Tyrosine kinase signaling pathways. There was observable cytoskeletal reorganization (translocation of F-actin filaments from the cytoplasm to cell membranes) that resulted in more continuous intercellular junctions, without significantly enhancing known adherens and tight junction associated protein expression, or modulating claudin-5 phosphorylation in vitro.Citation47 A role for GDNF in regulating microvascular endothelial and epithelial tight junction barrier function had also been described in mammalian blood-brain, blood-retina, blood-testis and intestinal barriers in vitro or in vivo,Citation49-Citation56 in addition to the in vitro BNB. In order to ascertain whether GDNF regulates mammalian BNB function in vivo, we performed macromolecular permeability assessments of endoneurial microvessels using a validated conditional knockout murine model following non-transecting sciatic nerve crush injury, with appropriate controls. We also sought to determine whether changes in VE-Cadherin and claudin-5 expression by endoneurial microvessels (as markers of adherens and tight junctions respectively) were associated with GDNF-mediated BNB macromolecular permeability regulation following sciatic nerve crush injury.
Results
Tamoxifen-induced conditional GDNF gene knockdown inhibits gene transcription
Four days following intraperitoneal tamoxifen injection, there was 71.7% mean reduction in GDNF mRNA levels in the sciatic nerves of CKO mice (mean GDNF ΔCt 14.88) compared to WT mice (mean GDNF ΔCt 13.06). HET mice (mean GDNF ΔCt 13.75) demonstrated an average 37.4% reduction in GDNF mRNA levels compared to WT mice. There was no significant difference in GDNF mRNA expression in CKO mice without tamoxifen injection compared to HET mice with a mean 32.5% reduction in transcript expression (mean GDNF ΔCt 13.63) compared to WT mice. These data imply partial GDNF gene disruption by the inserted LacZ construct, and effective, but incomplete inhibition of floxed allele transcription following tamoxifen-induced estrogen receptor-dependent Cre recombinase activation in murine sciatic nerves, as previously observed with this model.Citation57
Basal mouse sciatic nerve GDNF expression is not modulated by conditional knockout
There was no significant difference in basal GDNF protein levels in mouse sciatic nerves between the different genotypes. The cohort of GDNF WT mice studied had a mean protein level of 43.4 (± 35.1) pg/µg total protein, while HET and CKO mice had mean protein levels of 54.8 (± 44.6) pg/µg total protein and 62.3 (± 29.3) pg/µg total protein respectively (p-values: WT vs. HET = 0.423, WT vs. CKO = 0.347 and HET vs. CKO = 0.445). These data suggest that partial or conditional knockout does not influence basal endogenous GDNF protein expression, implying no significant gene transcription and translation occurs in physiologically normal adult murine sciatic nerves due to low GDNF turnover post-embryonically.
Induced GDNF expression following sciatic nerve crush injury is abrogated by conditional knockout
Following sciatic nerve crush injury, the expected upregulation in GDNF protein expression was observed in WT and HET mice on day 7 (mean 7.5-fold increase in both genotypes, p = 0.5), as shown in . This upregulation was abrogated in tamoxifen-induced GDNF CKO mice (1.6-fold increase, p values: WT vs. CKO = 0.0028, HET vs. CKO = 0.046, ), implying suitability of this model to investigate the effect of endogenous GDNF in mouse sciatic nerve BNB function in vivo following traumatic peripheral nerve injury. These data also demonstrate that one functional GDNF allele is sufficient for maximal GDNF protein upregulation in the mouse sciatic nerve in vivo 7 days following crush injury.
Figure 1. Effect of crush injury on sciatic nerve GDNF protein expression. Tamoxifen-induced conditional GDNF knockout (CKO) was associated with truncated sciatic nerve GDNF protein expression upregulation as measured by ELISA on day 7 following crush injury relative to its Sham surgery control. This is in contrast to the robust upregulation observed in GDNF wildtype (WT) and heterozygote (HET) mice. No differences were seen between the latter two genotypes. N = 3-4 mice per genotype in duplicate experiments. N.S. = not significant, **indicates p < 0.01.
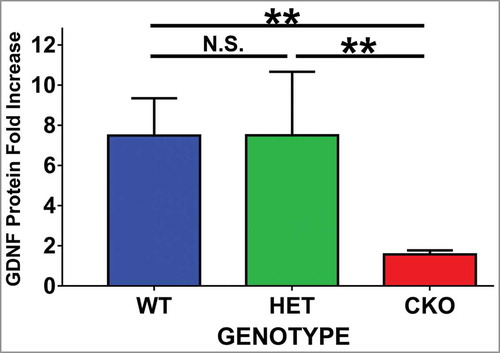
Endoneurial microvessel HRP permeability is modulated by GDNF following crush injury
By two hours following sciatic nerve crush injury, increased endoneurial microvessel permeability to HRP was observed in all microvessels, associated with endothelial cell swelling and loss of distinct cell membranes between endothelial cells and pericytes (), in contrast to Sham control nerves (). Quantitative morphometric evaluation of BNB HRP permeability performed on ultra-thin plastic-embedded sciatic nerve sections by electron microscopy (total of 302 microvessels, mean 6–7 microvessels/mouse) demonstrated significant differences in mean % permeable endoneurial microvessels per mouse genotype at day 7 compared to its sham control nerves (WT 21.4 (± 5.6)% vs. 4.2 (± 4.2)%, p = 0.01; HET 24.4 (± 5.2)% vs 7.7 (± 4.5)%, p = 0.05 and CKO 68.1 (± 10.9)% vs. 15.1 (± 8.3)%, p = 0.01, as shown in . There was no difference in the mean % permeable Sham-treated endoneurial microvessels between the genotypes (p-values 0.13-0.29). Upregulation in sciatic nerve GDNF expression (as shown by ELISA) was significantly associated with reduced % of permeable endoneurial microvessels compared to conditional knockdown (WT vs. CKO p = 0.004, HET vs. CKO p = 0.006) with no significant difference between WT and HET mice (p = 0.35), as shown in .
Figure 2. Early effect of crush injury on endoneurial microvascular permeability to horseradish peroxidase. Representative high magnification digital photomicrographs from the sciatic nerves of a GDNF WT mouse 2 hours following crush injury shows a horseradish peroxidase (HRP)-permeable microvessel with loss of distinct borders between endoneurial endothelial cells (EC) and pericytes (P) due to extravasation of electron-dense HRP from the lumen with a red blood cell (RBC) present (A). This is in contrast to a HRP-impermeable endoneurial microvessel from the contralateral Sham surgery control nerve with distinct borders between EC and P (white arrows) with luminal HRP and a RBC also observed. Scale bars = 500 nm.
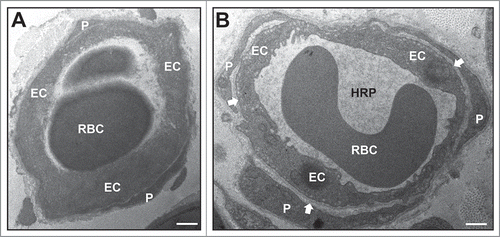
Figure 3. Quantitative effect of GDNF on sciatic nerve endoneurial microvessel HRP permeability on day 7 following crush injury. Bar histograms of mean % HRP-permeable endoneurial microvessels day 7 following sciatic nerve crush injury show significantly increased permeability in GDNF WT (blue histograms), HET (green histograms) and CKO (red histograms) mice compared to the contralateral Sham surgery controls (purple histograms) for each genotype (A). Significant reductions in permeability were seen in WT and HET mice relative to CKO mice, with no differences between the former two genotypes (B). These data imply GDNF significantly contributes to near complete recovery in restrictive BNB characteristics 7 days after nerve injury. N = 12 mice, with a total of 151 microvessels evaluated. *indicates p < 0.05, **indicates p < 0.01, ****indicates p < 0.0001 and N.S. indicates not significant. Upper indicators in the comparison bar in B depict significance relative to Sham controls, while lower indicators indicate significance relative to CKO.
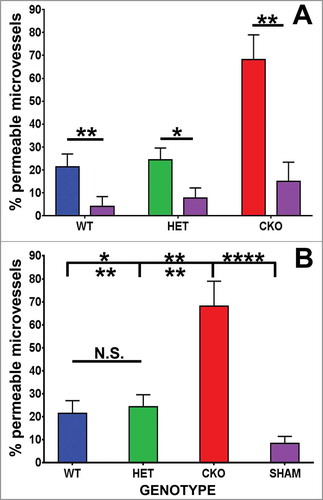
Mean % permeable endoneurial microvessels in all the genotypes following crush injury were significantly different from all Sham surgery control nerves [SHAM 8.4 (± 3.1)% vs. WT, p = 0.02; vs. HET, p = 0.01; vs. CKO, p = 2.7 × 10–6, ]. These data indicate an important role of GDNF in facilitating recovery of restrictive BNB function towards unaffected nerves 7 days after crush injury. Representative light microscopy digital photomicrographs demonstrate differences in axonal degeneration and myelinated axon density in WT and HET mice compared to CKO mice (), with digital ultramicrographs demonstrating HRP-permeable and impermeable endoneurial microvessels in the crush-injured sciatic nerves of WT, HET and CKO mice, compared to Sham surgery controls ().
Figure 4. Qualitative effect of GDNF on sciatic nerve morphology on day 7 following crush injury. Representative digital light photomicrographs from the sciatic nerves of experimental mice 7 days following sciatic nerve crush injury at low (A, D, G) and high magnification (B, E, H) show increased axonal density in GDNF WT (A, B) and HET (D, E) mice compared to CKO mice (G, H) which demonstrates more myelin debris with evidence of active Wallerian degeneration (black arrows), consistent with previous reports demonstrating a protective role for GDNF following axonal injury. Uninjured sham surgery control nerves (C, F, I) from the same mice are also shown. Scale bars = 25 µm (C), 50 µm (B, E, F, H, I) and 200 µm (A, D, G).
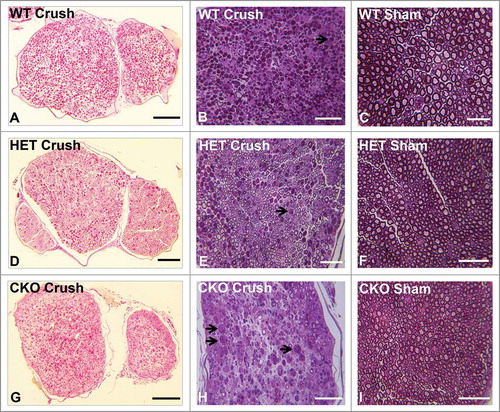
Figure 5. Qualitative effect of GDNF on sciatic nerve endoneurial microvessel HRP permeability on day 7 following crush injury. Representative digital ultramicrographs from the sciatic nerves of experimental mice 7 days following crush injury show HRP-impermeable endoneurial microvessels in GDNF WT (A) and HET (D) mice with a HRP-permeable endoneurial microvessel in a CKO mouse (G). HRP-impermeable endoneurial microvessels are seen in Sham control nerves for all genotypes (B, E, H). Less frequent HRP-permeable endoneurial microvessels are seen in WT (C) and HET (F) crush-injured nerves, with HRP-impermeable endoneurial microvessels less commonly observed in CKO crush-injured nerves (I). Scale bars = 2 µm.
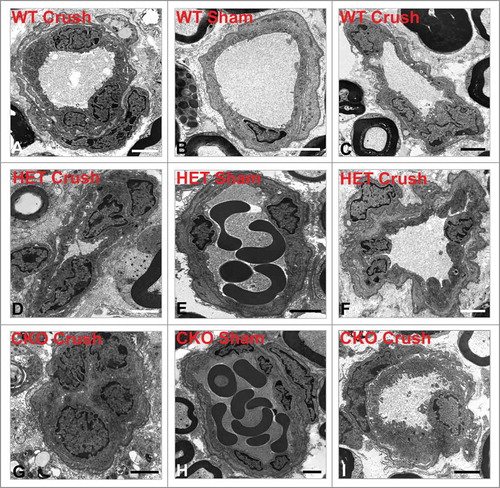
Full recovery in BNB HRP impermeability was observed in WT and HET mice by day 14 following sciatic nerve crush injury, with no difference in mean % permeable endoneurial microvessels compared to Sham surgery control nerves in the same mice [WT 8.1 (± 4.9)% vs. 5.0 (± 5.0)%, p = 0.37; HET 5.6 (± 5.6) vs. 4.2 (± 4.2)%, p = 0.5]. However, BNB functional recovery was delayed in CKO mice relative to its Sham controls [37.9 (± 5.2)% vs. 8.3 (± 8.3)%, p = 0.01], as shown in . As observed at day 7, there was no significant difference in the mean % permeable Sham-treated endoneurial microvessels between the genotypes (p-values 0.34-0.45). Similarly, upregulation in sciatic nerve GDNF expression (as demonstrated by ELISA) was significantly associated with reduced % of HRP permeable endoneurial microvessels compared to conditional knockdown (WT vs. CKO p = 0.003, HET vs. CKO p = 0.004) with no significant difference between WT and HET mice (p = 0.37), as shown in .
Figure 6. Quantitative effect of GDNF on sciatic nerve endoneurial microvessel HRP permeability on day 14 following crush injury. Bar histograms of mean % HRP-permeable endoneurial microvessels day 14 following sciatic nerve crush injury show no significant differences in HRP permeability in GDNF WT (blue histograms) and HET(green histograms) mice, with a significant difference observed in CKO (red histograms) mice compared to their contralateral Sham surgery controls (purple histograms) for each genotype (A). Significant reductions in % permeable microvessels were seen in WT and HET mice relative to CKO mice, with no differences between the former two genotypes (B). These data imply GDNF plays a significant role in facilitating complete restrictive BNB recovery 14 days after crush injury relative to baseline macromolecular permeability seen in Sham surgery control nerves. N = 12 mice, with 151 microvessels evaluated. **indicates p < 0.01, ****indicates p < 0.0001 and N.S. indicates not significant. Upper indicators in the comparison bar in B indicate significance relative to Sham controls, while lower indicators indicate significance relative to CKO.
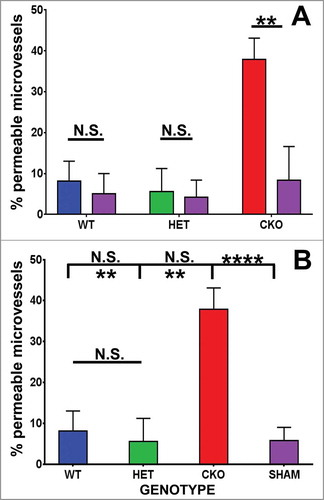
Mean % permeable endoneurial microvessels in WT and HET mice following crush injury were not significantly different from all Sham surgery control nerves [SHAM 5.8 (± 3.2)% vs. WT, p = 0.36; vs. HET, p = 0.48) with a significant difference observed compared to CKO mice (p = 9.0 × 10–5, ]. These data show that GDNF significantly restores restrictive BNB function to baseline by 14 days following non-transecting crush injury in murine sciatic nerves, consistent with observations in rat sciatic nerves, using Evans Blue to determine BNB permeability.Citation58 Representative light microscopy digital photomicrographs demonstrate differences in axonal degeneration, regeneration, remyelination and myelinated axon density in WT and HET mice compared to CKO mice at day 14 (), with digital ultramicrographs demonstrating HRP-permeable and impermeable endoneurial microvessels in the crush-injured WT, HET and CKO mice, compared to Sham surgery controls ().
Figure 7. Qualitative effect of GDNF on sciatic nerve morphology on day 14 following crush injury. Representative digital light photomicrographs from the sciatic nerves of experimental mice 14 days following sciatic nerve crush injury at low (A, E, H) and high magnification (B, D, F, I) show a completely regenerated nerve segment in a GDNF WT mouse (A, B). Increased axonal density with clusters of numerous thinly myelinated regenerating axons (black arrows) are seen in another GDNF WT (D) and a HET (E, F) mouse compared to CKO mice (H, I) which is consistent with previous reports demonstrating a supportive role for GDNF in peripheral axonal regeneration following injury. Intact appearing endoneurial microvessels (white arrows) are shown in the regenerating WT (D) and HET (F) mouse sciatic nerves at higher magnification. Uninjured Sham surgery control nerves (C, G, J) from these mice are also shown. Scale bars = 50 µm (B, C, D, F, G, I, J) and 200 µm (A, E, H).
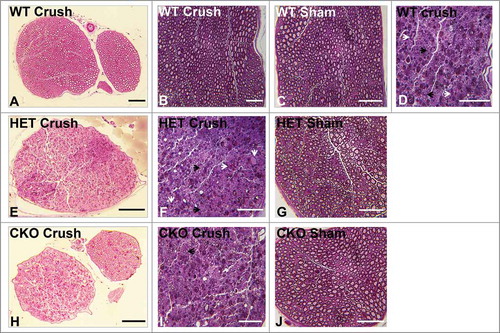
Figure 8. Qualitative effect of GDNF on sciatic nerve endoneurial microvessel HRP permeability on day 7 following crush injury. Representative digital ultramicrographs from the sciatic nerves of experimental mice 14 days following crush injury show HRP-impermeable endoneurial microvessels in GDNF WT (A) and HET (D) mice with a HRP-permeable endoneurial microvessel in a CKO mouse (G). HRP-impermeable endoneurial microvessels are seen in Sham control nerves for all genotypes (B, E, H). As observed at day 7, less frequent HRP-permeable endoneurial microvessels are seen in WT (C) and HET (F) crush-injured nerves, with HRP-impermeable endoneurial microvessels less commonly observed in CKO crush-injured nerves (I) compared to WT and HET mice that had equivalent numbers to Sham controls. Scale bars = 2 µm apart from (B) 1 µm.
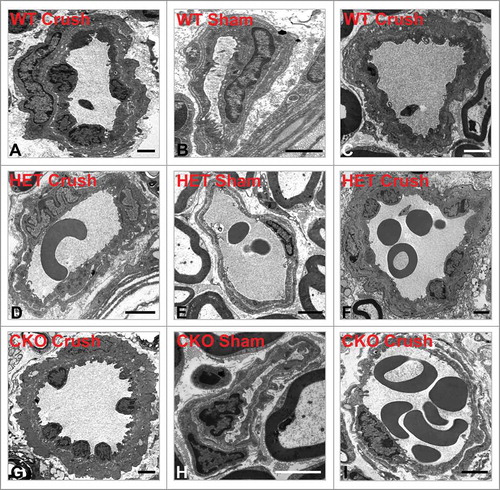
Sciatic nerve endoneurial microvessel VE-Cadherin and claudin-5 expression is not altered by crush injury or modulated by GDNF during recovery
There was no significant difference in the % of VE-Cadherin or claudin-5 positive endoneurial microvessels observed within three hours of sciatic nerve crush injury (indicative of the acute phase) or during recovery on days 7 and 14 relative to Sham surgery nerves. No differences were also observed between the different genotypes and different time points (). The average numbers of endoneurial microvessels analyzed on days 0, 7 and 14 were 282, 278 and 307 respectively. Mean % endoneurial microvessels in the crush-injured sciatic nerves of GDNF WT, HET and CKO mice that were VE-Cadherin positive was 80.7%, 77.6% and 98.1% (compared to Sham surgery expression of 86.1%, 89.0% and 100% respectively) on day 0. 100% of the endoneurial microvessels in crush-injured and Sham surgery nerves expressed VE-Cadherin on days 7 and 14 in all genotypes. Representative digital photomicrographs depicting sciatic nerve VE-Cadherin expression on day 0 (), day 7 () and day 14 () following sciatic nerve crush injury in GDNF WT, HET and CKO mice are shown.
Figure 9. Quantitative expression of VE-Cadherin and claudin-5 during the immediate acute and recovery phases following crush injury. Bar histograms of mean % of CD31+ endoneurial microvessels expressing VE-Cadherin and claudin-5 on days 0, 7 and 14 following sciatic nerve crush injury show no differences between crush-injured GDNF WT (blue histograms), HET (green histograms) and CKO (red histograms) nerves and their Sham surgery control (purple histograms) nerves, or differences in expression between GDNF WT, HET and CKO mice at the different time points studied. These data imply that neither VE-Cadherin nor claudin-5 regulation is associated with the initial injury or the observed GDNF-mediated restoration in BNB macromolecular permeability function following non-transecting sciatic nerve crush injury in mice. N = 12 mice per time point, and N.S. indicates not significant.
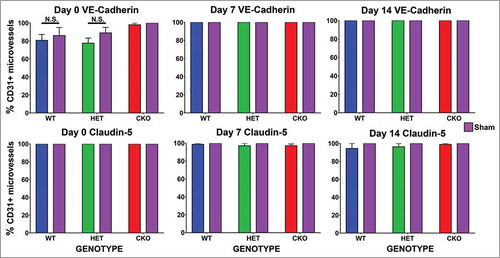
Figure 10. Qualitative expression of VE-Cadherin on endoneurial microvessels in GDNF WT, HET and CKO mice within 3 hours following crush injury. Representative digital photomicrographs from the sciatic nerves of experimental mice within three hours following crush injury (day 0) at low magnification to demonstrate most of the nerve show endoneurial microvessels (green; A, E and I) that are VE-Cadherin positive (red; B, F and J) in crush-injured nerves, further shown with merged images (yellow; C, G and K). An equivalent % is seen in Sham surgery control nerves (yellow; D, H and L). Examples of VE-Cadherin positive endoneurial microvessels are demonstrated by the single white arrows. VE-Cadherin expression is also observed in the perineurium (double white arrows) and endoneurial cells with Schwann cell profiles and distribution (white asterisk). Nuclei are depicted in blue. Scale bars = 100 µm, apart from L (50 µm).
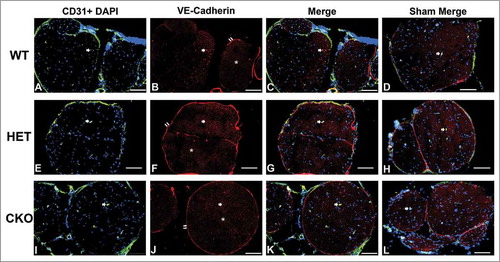
Figure 11. Qualitative expression of VE-Cadherin on endoneurial microvessels in GDNF WT, HET and CKO mice on day 7 following crush injury. Representative digital photomicrographs from the sciatic nerves of experimental mice on day 7 following crush injury at low magnification to demonstrate most of the nerve show endoneurial microvessels (green; A, E and I) that are VE-Cadherin positive (red; B, F and J) in crush-injured nerves, further shown with merged images (yellow; C, G and K). An equivalent % is seen in Sham surgery control nerves (yellow; D, H and L). Examples of VE-Cadherin positive endoneurial microvessels are demonstrated by the single white arrows. VE-Cadherin expression is also observed in the perineurium (double white arrows) and endoneurial cells with Schwann cell profiles and distribution (white asterisk). Nuclei are depicted in blue. Scale bars = 100 µm.
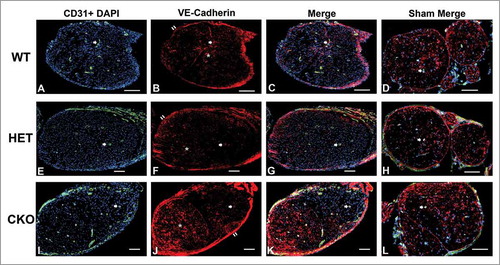
Figure 12. Qualitative expression of VE-Cadherin on endoneurial microvessels in GDNF WT, HET and CKO mice on day 14 following crush injury. Representative digital photomicrographs from the sciatic nerves of experimental mice on day 7 following crush injury at low magnification to demonstrate most of the nerve show endoneurial microvessels (green; A, E and I) that are VE-Cadherin positive (red; B, F and J) in crush-injured nerves, further shown with merged images (yellow; C, G and K). An equivalent % is seen in Sham surgery control nerves (yellow; D, H and L). Examples of VE-Cadherin positive endoneurial microvessels are demonstrated by the single white arrows. VE-Cadherin expression is also observed in the perineurium (double white arrows) and endoneurial cells with Schwann cell profiles and distribution (white asterisk). Nuclei are depicted in blue. Scale bars = 100 µm.
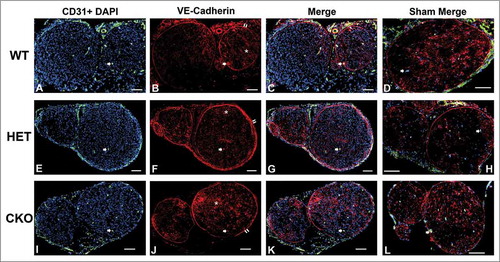
100% of the endoneurial microvessels in the crush-injured sciatic nerves retained claudin-5 expression within three hours of injury. Mean % of endoneurial microvessels in GDNF WT, HET and CKO mice that were claudin-5 positive was 98.9%, 97.3% and 97.3% on day 7 and 94.3%, 96.3% and 98.9% on day 14 (compared to 100% on Sham surgery nerves). Representative digital photomicrographs depicting sciatic nerve claudin-5 expression on day 0 (), day 7 () and day 14 () following sciatic nerve crush in GDNF WT, HET and CKO mice are shown.
Figure 13. Qualitative expression of claudin-5 on endoneurial microvessels in GDNF WT, HET and CKO mice within 3 hours following crush injury. Representative digital photomicrographs from the sciatic nerves of experimental mice within three hours following crush injury (day 0) at low magnification to demonstrate most of the nerve show endoneurial microvessels (green; A, E and I) that are claudin-5 positive (red; B, F and J) in crush-injured nerves, further shown with merged images (yellow; C, G and K). An equivalent % is seen in Sham surgery control nerves (yellow; D, H and L). Examples of claudin-5 positive endoneurial microvessels are demonstrated by the white arrows. Scale bars = 500 µm.
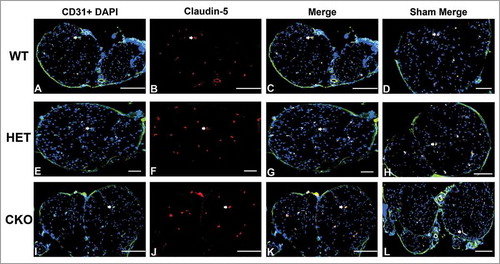
Figure 14. Qualitative expression of claudin-5 on endoneurial microvessels in GDNF WT, HET and CKO mice on day 7 following crush injury. Representative digital photomicrographs from the sciatic nerves of experimental mice on day 7 following crush injury at low magnification to demonstrate most of the nerve show endoneurial microvessels (green; A, E and I) that are claudin-5 positive (red; B, F and J) in crush-injured nerves, further shown with merged images (yellow; C, G and K). An equivalent % is seen in Sham surgery control nerves (yellow; D, H and L). Examples of claudin-5 positive endoneurial microvessels are demonstrated by the white arrows. Scale bars = 500 µm, apart from A-C and H (200 µm).
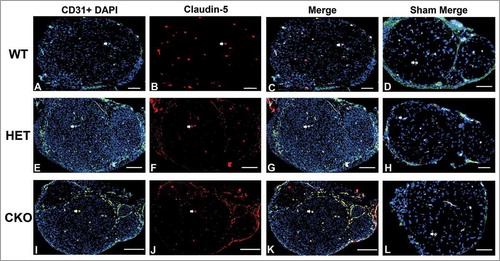
Figure 15. Qualitative expression of claudin-5 on endoneurial microvessels in GDNF WT, HET and CKO mice on day 14 following crush injury. Representative digital photomicrographs from the sciatic nerves of experimental mice on day 14 following crush injury at low magnification to demonstrate most of the nerve show endoneurial microvessels (green; A, E and I) that are claudin-5 positive (red; B, F and J) in crush-injured nerves, further shown with merged images (yellow; C, G and K). An equivalent % is seen in Sham surgery control nerves (yellow; D, H and L). Examples of claudin-5 positive endoneurial microvessels are demonstrated by the white arrows. Scale bars = 500 µm, apart from D (200 µm), H (100 µm) and L (1000 µm).
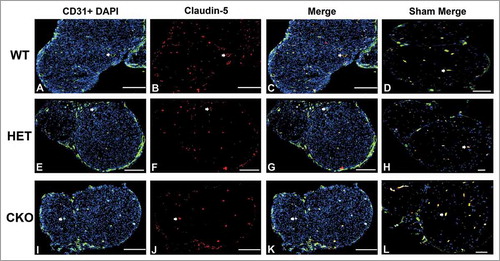
These data imply that neither VE-Cadherin nor claudin-5 expression on endoneurial microvessels was associated with the loss of the intercellular junction complex seen acutely or the restoration of the restrictive BNB macromolecular permeability mediated by GDNF (as evidenced by reduced BNB HRP permeability in WT and HET mice compared to CKO mice), as demonstrated by electron microscopy. This is consistent with prior observations showing no significant regulation of these molecules during GDNF-mediated recovery of the human BNB biophysical properties in vitro following serum withdrawal,Citation47 suggesting that other cadherins, claudins or associated intercellular junctional complex molecules may be more physiologically relevant to the specialized restrictive mammalian BNB function.
Discussion
In this study, we provide evidence supporting an important role for GDNF in restoring restrictive mouse BNB characteristics, as defined as impermeability to a large macromolecule, following sciatic nerve crush injury. Horseradish peroxidase is not known to undergo restrictive barrier-forming microvascular endothelial cell transcytosis under normal physiologic conditions (due to the relative paucity of pinocytic vesicles),Citation40,Citation59-Citation61 consistent with paracellular luminal transport across the BNB as seen in this study. Electron microscopy provides the most sensitive way of evaluating individual microvessel paracellular permeability and structural integrity. Low power light and fluorescent photomicrographs were also frequently displayed to demonstrate global changes that occur within the sciatic nerve endoneurium. These photomicrographs also validate the method of inducing nerve injury and subsequent tissue processing, as the crush-injured nerves maintained the morphological characteristics of mouse sciatic nerves with a demonstrable perineurial layer. Transecting nerve injury or tissue fragmentation due to inadequate nerve processing could result in inaccurate BNB molecular or permeability inferences.
The data supports our previous work demonstrating a sufficient role of GDNF in restoring restrictive human BNB biophysical properties in vitro at low nanomolar concentrations following diffuse injury mediated by serum withdrawal (which causes endothelial cell detachment and formation of intercellular gaps).Citation47 In that study, partial or insufficient roles in restoring restrictive BNB properties were observed with transforming growth factor-β1, basic fibroblast growth factor, hydrocortisone and cyclic AMP-protein kinase A (cAMP-PKA) signaling. Mouse endoneurial microvessels develop altered intercellular junctions in vivo following sciatic nerve crush injury (as shown by electron microscopy, see ), similar to the intercellular junctional membrane detachment seen between adjacent primary human endoneurial endothelial cells (that form the BNB) following serum withdrawal in vitro, with associated increases in macromolecular permeability.Citation47
GDNF is expressed by myelinating and nonmyelinating Schwann cells in peripheral nerves and Schwann cell proliferation is a known compensatory event in peripheral nerves in response to endoneurial injury or inflammation in mammals.Citation26,Citation62,Citation63 Increased GDNF expression (as shown in this study) is a known consequence of peripheral nerve or nerve root injury, and its upregulated expression is temporally associated with axonal regeneration. Recent work in adult male Sprague-Dawley rats demonstrated activation of Schwann cell P2 type purinergic receptors following sciatic nerve injury that resulted in protein kinase C signaling and subsequent activation of protein kinase D, resulting in induced GDNF transcription.Citation26 However, a direct role of GDNF in restoring BNB function in vivo was previously unknown.
GDNF has been proposed to serve as a general paracrine regulator of endothelial tight junctions in restrictive blood-tissue barriers based on work with porcine brain capillary and human brain microvascular cells (blood-brain barrier),Citation49,Citation51 rat retinal microvascular endothelial cells (inner blood-retinal barrier)Citation50 and rat testicular microvessels (blood-testis barrier).Citation52 These specialized endothelial cells express GDNF receptor, GFR α1 on their cell membranes in vitro or in situ. There is also evidence that GDNF (secreted by enteric glial cells) regulates the intestinal epithelial barrier in vitro and in vivo, with a potential role in barrier maturation (via p38 MAPK pathway), wound healing (via cAMP/PKA pathway) and in the pathogenesis of inflammatory bowel disease.Citation53-Citation56
Human primary endoneurial endothelial cells that form the BNB express the GDNF receptor, GFRα1 and its canonical signaling complex RET in vitro and in situ, with in vitro evidence suggesting some autocrine GDNF secretion following diffuse injury.Citation46-Citation48 Interestingly, indirect immunohistochemistry suggested endoneurial GDNF microvessel expression in freshly dissected uninjured ex vivo rat sciatic nerve segments (which was not observed in myelinating and non-myelinating Schwann cells),Citation26 suggesting that autocrine GDNF secretion may be an early compensatory event to maintain essential BNB function necessary for endoneurial homeostasis prior to paracrine secretion by Schwann cells. In support of this hypothesis and to support potential biological relevance in humans, our recent work deducing the normal human adult BNB transcriptome showed endogenous transcript expression of P2 purinergic receptors P2RX4, P2RX7, P2RY2 an P2RY11; protein kinase C isoforms PRKCA, PRKCD, PRKCE, PRKCH, PRKCI and PRKCZ, and protein kinase D isoforms PRKD1, PRKD2 and PRKD3 by RNA-sequencing.Citation48 This implies that endoneurial endothelial cells that form the BNB have the molecular machinery to secrete GDNF in response to injury, as seen in Schwann cells.
Ongoing work suggests a crucial role for Ras-MAPK signaling in GDNF-mediated restoration of the human BNB in vitro following diffuse injury.Citation64 However, GDNF had very modest heparin-dependent effects on primary human endoneurial endothelial cell proliferation and angiogenesis relative to basal medium without added mitogens, with no significant effect on the sterile micropipette-induced wound healing in vitro (which was primarily mediated by vascular endothelial growth factor [VEGF]A165).Citation46 These observations imply that GDNF may function as an essential regulator of restrictive barrier formation following angiogenesis in peripheral nerve endoneurium. Based on this, it is plausible that Schwann cells could directly regulate tight junction formation in endoneurial microvessels following injury, as well as during peripheral nerve development via GDNF secretion. Direct evaluation of Ras-MAPK signaling in vivo following crush injury is challenging due to the widespread application of this signaling pathway in homeostatic biologic functions. A significant goal is to elucidate downstream signaling pathways preferentially activated in endoneurial microvessels during GDNF-mediated BNB restoration after injury.
Murine sciatic nerve crush injury was not associated in changes in VE-Cadherin or claudin-5 expression on endoneurial microvessels acutely (within three hours of injury) or during restoration of BNB macromolecular permeability function mediated by GDNF, consistent with observations made at the human BNB in vitro following serum withdrawal.Citation47 Recent work elucidating the normal adult human BNB transcriptome (supported by indirect immunohistochemistry) demonstrated the extensive molecular repertoire of the intercellular junctional complex in endoneurial microvessels.Citation48 Apart from endoneurial microvessels, the innermost layer of the perineurium and myelinated Schwann cells also form specialized tight junctions in peripheral nerves, with some molecular component overlap.Citation65 Coupled with the cytoskeletal changes that occur during Schwann cell proliferation and remyelination and axonal regeneration during peripheral nerve recovery following injury, this complexity makes deducing the key adherens and tight junction molecules responsible for BNB macromolecular impermeability a challenge in vivo. Work is ongoing to decipher which specific adherens and tight junction molecules are modulated by GDNF during human BNB functional restoration in vitro for subsequent in vivo validation in mice, taking into consideration that phenotypic differences may occur between the human and mouse BNB.
Treatment strategies to repair injured peripheral nerves should consider BNB functional restoration within the endoneurium following microvascular angiogenesis in addition to axonal regeneration and re-attachment to the distal nerve stump to maintain connective tissue alignment as being essential facets to recovery. It is logical to assume the early restoration of the endoneurial microenvironment by a fully functional BNB could aid provide a permissive environment for axonal repair. There is some evidence that strategies involving increased local expression of VEGF and GDNF resulted in more rapid and effective repair in a spinal cord tissue injury model.Citation66 Human endoneurial endothelial cells are also known to express connective tissue growth factor in vitro and in situ,Citation48 implying that a healthy functional BNB may contribute to local wound healing and restoration of endoneurial homeostasis and its fibrous architecture following injury. Restoring BNB function may be a necessary requisite to re-establish the tightly regulated microenvironment required for axonal regeneration and physiologic signal transduction in traumatic peripheral neuropathies, suggested by a study demonstrating restrictive BNB recovery preceding complete axonal regeneration by 6 weeks in a rat sciatic nerve crush injury model.Citation58
GDNF knockout mice are embryonically lethal, so conditional knockout models in adult mice provide tools to elucidate its role in restoring BNB function in vivo, adding knowledge to its known role in axonal regeneration. Tissue specific deletion in Schwann cells would provide additional specificity; however, it has been shown that myelinating and non-myelinating Schwann cells are the source of GDNF in rodents following sciatic nerve crush,Citation26 and our prior in vitro data demonstrated a crucial role for exogenous GDNF in restoring human BNB function following diffuse endothelial injury.Citation47 Further work is needed to confirm our in vitro observations of GDNF-mediated cytoskeletal remodeling as essential for human BNB function restoration, and the molecular determinants and mechanisms by which the intercellular junctional complex is rebuilt and BNB function restored in mammalian nerves following injury. Targeted GDNF delivery and assessment of endoneurial microvessel macromolecular permeability should be considered in addition to assessments of axonal regeneration when designing therapeutic strategies in traumatic peripheral neuropathies.
Materials and methods
Conditional GDNF Knockout Mouse Model
GDNFFlox/Flox mice (129/SvJ: C57BL/6, containing LoxP flanking sequences on GDNF exon 3) and GDNFLacZ/+ were obtained via a Materials Transfer Agreement from Instituto de Biomedicina de Sevilla (IBiS), Seville, Spain. Mice were housed in the Research Services Building, University of Alabama at Birmingham, Birmingham, in a specific dedicated pathogen-free room maintained at 24°C. All mice were kept in micro-isolator cages with chow and water provided ad libitum, maintaining a 12 hour light–dark cycle with standard environmental enrichment without restrictions to free movement. Research studies received approval from the Institutional Animal Care and Use Committee, and were conducted in accordance with the United States Policy on Humane Care and Use of Laboratory Animals.
To generate experimental mice, heterozygous GDNF C57BL/6 mice (GDNFLacZ/+) were crossed with the commercially available hemizygous Esr1-Cre recombinase (Esr1-cre/+) C57BL/6 transgenic mice (JAX Mice) to generate GDNFLacZ/+; Esr1-Cre/+ mice. These mice were crossed with GDNFFlox/Flox mice to generate experimental tamoxifen-inducible conditional GDNF knockout mice (CKO; GDNFLacZ/Flox; Esr1-Cre/+) and control littermates (wildtype [WT]: GDNF Flox/+; +/+; heterozygous [HET]: GDNFLacZ/Flox; +/+).Citation57
Genomic DNA was extracted from tail snips of tagged mice at 3 weeks of age during weaning using the DirectPCR Lysis Reagent (Viagen Biotech, cat # 102-T). Mouse genotype was determined by polymerase chain reaction (PCR) using LacZ-specific primers; Forward: 5′- CTT GCC TGG TGC GGT TCT CT-3′ and Reverse: 5′- GCT GGC GAA AGG GGG ATG TG-3′; and Cre-specific primers; Forward: 5′- ACG GGC ACT GTG TCC AG –3′ and Reverse: 5′- TGT TCA GGG ATC GCC AG –3′, as previously published.Citation57 To induce GDNF knockout, CKO (GDNFLacZ/Flox; Esr1-Cre/+) mice at least 8 weeks of age were injected intraperitoneally with 100 µL of tamoxifen 100 mg/kg body weight (Sigma-Aldrich, CAS # T-5648) dissolved in corn oil (Sigma-Aldrich, CAS # C-8267) per diem for four consecutive days. GDNFFlox/Flox mice and CKO mice without tamoxifen injection were used as experimental controls when indicated.
Real time-Quantitative Polymerase Chain Reaction (rt-qPCR)
To determine the effect of tamoxifen-induced conditional GDNF knockout on messenger RNA (mRNA) expression in adult mouse sciatic nerves, we performed TaqMan® rt-qPCR in WT, HET, and CKO mice with and without tamoxifen injection 4 days after initial injection. Briefly, mRNA was extracted from snap frozen full-length mouse sciatic nerves harvested under deep anesthesia (from sciatic notch to the popliteal fossa) and homogenized with a disposable pestle device on ice, using TRIzol® reagent (Invitrogen, cat # 15596026) according to the manufacturer‘s instructions, as previously published.Citation67,Citation68 cDNA was synthesized using SuperScript™ IV VILO™ Master Mix with ezDNase™ Enzyme (Invitrogen, cat # 11766050). rt-qPCR was performed with an ABI StepOnePlus Real-Time PCR System (Applied Biosystems) using fast TaqMan® Master Mix (Applied Biosystems) and light cycler amplification conditions with adjustable threshold detection as recommended by the manufacturer. rt-qPCR was performed on 3–4 mice per experimental group (in duplicate).
To quantify GDNF mRNA, sequence amplification from Exon 1 to Exon 3 was performed using forward primer 5′- CGG GCC ACT TGG AGT TAA TG-3′, reverse primer 5′- TAA TCT TCA GGC ATA TTG GAG TCA CT-3′ and probe 5′- CTG CCT GGT GTT GCT-3′, with 40 ng of cDNA template for each reaction. In each sample, β-actin mRNA was also quantified as an internal housekeeping gene control. 12.5 ng of cDNA template was used with the following primers; Forward: 5′-GGC CCA GAG CAA GAG AGG TA-3′; Reverse: 5′-CAT GTC GTC CCA GTT GGT AAC A-3′. Standardized FAM-labeled TaqMan® probe amplification assays were performed and GDNF expression relative to β-actin was determined, with comparisons between experimental groups using the 2–ΔΔCt method relative to GDNF WT mouse expression.
GDNF Enzyme-Linked Immunosorbent Assay (ELISA)
Mouse sciatic nerve GDNF protein levels were quantified in adult GDNF WT, HET and tamoxifen-induced CKO mice under basal conditions and following sciatic nerve crush injury (see protocol below) using a commercially available ELISA kit (GDNF Emax Immunoassay System, Promega cat # G7620). GDNFFlox/Flox mice were also used as WT mice in some experiments for comparison. From each mouse, the full-length sciatic nerves were harvested under deep anesthesia and total protein was extracted in 200 μL buffer containing 20 mM Tris (pH 8.0), 137 mM NaCl, 1% NP-40, 10% glycerol, 1 mM phenylmethylsulfonyl fluoride, 0.5 mM sodium orthovanadate and proteinase inhibitor cocktail (Sigma Aldrich, cat # P8340) by homogenizing and sonicating with a Fisher Scientific Sonic Dismembrator F60 for 10 s (output 2–3). Samples were centrifuged at 4°C for 20 min at 18,000 g using a Beckman Coulter microfuge 20R centrifuge and supernatant collected and stored at –80°C for future use. 3–4 mice per genotype were used in each experiment (performed in duplicate).
Total protein concentration was determined using the Pierce BCA protein Assay Kit (Thermo Fisher Scientific, cat # 23225), with absorbance measured at 562 nm. GDNF ELISA was performed following the manufacturer‘s protocol, with a standard curve of known GDNF concentrations used for each assay to determine GDNF concentration based on absorbance measured at 450 nm. A BioTek Synergy 2 multi-mode microplate reader was used to measure absorbance for both assays. The amount of GDNF (in pg) was normalized per µg of total sciatic nerve protein from the same mouse to facilitate comparative analyses between mice. To determine the relative effect of sciatic nerve crush injury on GDNF expression, the ratio of normalized GDNF protein following sciatic nerve crush to the contralateral Sham surgery (uncrushed) nerve was deduced in WT, HET and CKO mice following tamoxifen injection.
Murine sciatic nerve crush injury model
In order to mimic a traumatic peripheral neuropathy with endoneurial microvascular disruption, non-transecting sciatic nerve crush injury was performed in age- and sex-matched adult (>12 weeks old) GDNF WT, HET and CKO mice at least 4 weeks following tamoxifen injection. Survival surgery using aseptic techniques was performed on a temperature-regulated heated platform. Mice were anesthetized with inhalational isoflurane and hind limb hair removed bilaterally using Nair® hair remover. The operative sites were cleaned with 3% chloroxylenol for 2 min. Buprenorphine 0.1 mg/kg body weight was administered subcutaneously as a local analgesic prior to surgical incision.
Single longitudinal cutaneous incisions were performed bilaterally from the sciatic notch to the popliteal fossa with retraction of the hamstring muscles laterally. For each mouse, the right sciatic nerve underwent crush injury by applying firm pressure to the middle of the nerve with sterile forceps for 2–3 seconds. Sham surgery was performed on the left side by exposing the sciatic nerve only (i.e. without applying the crush procedure). Absorbable polyglactin 910 sutures (Ethicon Coated Vicryl 5-0; cat # J493G) were utilized to reattach the separated muscles and subcutaneous fascia planes; with non-absorbable nylon 6 sutures (Ethicon Ethlion 4-0; cat # 699G) used to indicate the site of sciatic nerve crush injury and to stitch the skin. Mice were monitored on a heating pad until fully awake and ambulatory prior to cage transfer. Subcutaneous buprenorphine (0.1 mg/kg) was administered postoperatively every 12 hours for 48 hours starting at 6:00 pm on the day of surgery to avoid interruption of light-dark cycle. Mice were monitored daily for the next 14 days.
Horseradish peroxidase endoneurial microvascular permeability assay
Horseradish peroxidase (HRP; molecular mass ∼44 kDa) was used to evaluate BNB integrity in vivo following sciatic nerve crush injury, adapting previously published macromolecular permeability assay techniques in rodent microvessels.Citation59,Citation69-Citation73 Two hours after, and on days 7 and 14 following sciatic nerve crush injury, cohorts of experimental mice were pre-warmed to 37°C in metal cages for 10 min and each mouse was comfortably placed in a restrainer. 30 mg/kg sterile filtered Type II HRP (Sigma-Aldrich, cat # P8250-25KU) in 1X PBS (reconstituted at 5 mg/mL) was administered via tail vein injection. 15 min afterwards, mice were anesthetized with ketamine-xylazine (100 mg-10 mg/kg body weight) in 100 μL1X PBS and both sciatic nerves were harvested 30 min after injection, as previously published.Citation63,Citation67,Citation74-Citation76 Nerve alignment was maintained by placing on filter paper with the sciatic nerve crush injury site indicated. The nerves were fixed with freshly prepared 3% glutaraldehyde in 0.1 M cacodylate buffer containing 2 mM CaCl2 for 4 hours in the dark at room temperature.
In order to enhance HRP detection by electron microscopy, fixed nerves were incubated with a diaminobenzidine (DAB)-hydrogen peroxide (H2O2) solution (0.5 mg/mL DAB + 0.01% H2O2 + 5% sucrose in 0.1 M cacodylate buffer containing 2 mM CaCl2) for 30 min at 37°C in a shaking incubator at 100 revolutions per minute in the dark. Following washes with 0.1 M cacodylate buffer twice, with 0.1 M phosphate buffer once for 5 min in the dark, fixed sciatic nerves were post-fixed in 1% osmium tetroxide for 2 hours. Following post-fixation, the sciatic nerves were transected at the site of sciatic nerve crush and embedded in epoxy resin side-by-side with the crushed ends superficially placed to generate plastic-embedded blocks, as previously published.Citation67,Citation74,Citation75
Light microscopy
Plastic-embedded sciatic nerve blocks were trimmed and axially sectioned with an LKB Ultrotome III ultramicrotome using a DiATOME Histo diamond knife. 0.5-1.0 µm semi-thin sections were mounted on glass slides and stained with basic fuchsin, followed by methylene blue counterstain. Briefly, 2–3 drops of basic fuchsin (Rosalinine chloride, basic violet 14 powder; 0.1 mg/mL in 30% ethanol) were applied to each glass slide to completely cover the sections. Slides were heated on a hot plate at ∼90–95°C for 15 seconds. Excessive stain was washed from each slide with a stream of distilled water. The methylene blue counterstain was performed by covering basic fuchsin-stained sections with 1–2 drops of methylene blue (0.6 mg/mL in 2% aqueous sodium tetraborate). Slides were heated on a hot plate at ∼90-95°C for 10 seconds followed by a wash with a stream of distilled water. Each slide was air-dried and mounted with coverslips using Cytoseal 60 mounting medium prior to taking digital photomicrographs using a Nikon DS-Fi2 color camera mounted on a Leitz Laborlux S light microscope. Images were processed using the Nikon Elements software.
Transmission electron microscopy
To identify the endoneurial microvessels in each mouse sciatic nerve and determine HRP permeability in situ, ultra-thin axial sections (70-90 nm) were cut from the plastic-embedded blocks using an LKB Ultrotome III ultramicrotome with a DiATOME Histo diamond knife and mounted on copper mesh grids, covering the entire cross-sectional area of each nerve. The sections were stained for contrast using 4% aqueous uranyl acetate, followed by Reynolds lead citrate stain. Briefly, sections were stained by placing grids section-side down on a drop of 4% aqueous uranyl acetate in a Petri dish. The dish was placed in an oven at 70–80°C for 8 min, washed in a stream of distilled water, and dried by capillary action by holding a strip of Whatman #1 filter paper to the edge of the grid. Each grid was subsequently placed section-side down on a drop of undiluted Reynolds lead citrate solution on a strip of Parafilm® at room temperature for 6 min, washed and dried as described above, and completely air-dried prior to insertion into specimen chamber of a Philips 201 transmission electron microscope operating at 60 kV. Digital ultramicrographs were obtained of each sciatic nerve endoneurial microvessel using a retro-fitted side-mounted SIA L3C 4.2 megapixel digital camera.
HRP-permeable endoneurial microvessels were defined as vessels demonstrating abluminal electron-dense HRP staining that obscures cell membrane borders between endoneurial endothelial cells and pericytes (which share the same basement membrane), while HRP-impermeable endoneurial microvessels were defined as vessels with electron-dense HRP restricted within their lumens with distinct cell membrane borders between the endoneurial cells and pericytes.Citation59,Citation69-Citation73 The percentage of HRP-permeable microvessels in each sciatic nerve was determined with direct comparisons between crush-injured and Sham control nerves performed per mouse, as well as comparisons between GDNF WT, HT and tamoxifen-induced CKO mice at days 7 and 14 post-injury.
Indirect fluorescent immunohistochemistry
In order to determine the molecular changes associated with adherens and tight junctions at the murine BNB following sciatic nerve crush injury and recovery under the influence of GDNF, indirect immunohistochemistry was performed to detect and quantify VE-Cadherin and claudin-5 expression on sciatic nerve endoneurial microvessels in GDNF WT, HET and tamoxifen-induced CKO adult mice on the day of crush injury and on days 7 and 14 following injury, using the contralateral Sham surgery nerves as internal controls. Four age- and sex-matched mice were studied per experimental group and time point.
Sciatic nerve crush injury and Sham surgery were performed as previously described. Within three hours of crush injury, a cohort of 12 mice was anesthetized with ketamine-xylazine and both sciatic nerves harvested as previously described. Following nerve alignment on filter paper, the mid-sciatic nerve at the site of crush injury was very carefully transected and both sections were aligned next to each other longitudinally and mounted in Optimum Cutting Temperature® compound with the transected regions most superficial. The transected nerves were stored at –80°C. This process was repeated on days 7 and 14 post-injury in other cohorts of 12 mice. Subsequent sectioning and sample processing for immunohistochemistry occurred at the same time for all the specimens to reduce technical variability.
Indirect immunohistochemistry was performed on serial 10 µm thick axial cryostat sections to detect and quantify VE-Cadherin (marker of adherens junctions) and claudin-5 (marker of tight junctions) on CD31 + endoneurial microvessels within mouse sciatic nerves, using previously published protocols.Citation63,Citation67,Citation68,Citation74-Citation76 Each axial section consisted of the two adjacent sciatic nerve sections from the crush-injured site. Sections were fixed in acetone for 10 min at –20°C, washed and air-dried prior to blocking with 10% normal goat serum in 1X PBS for 1 hour at room temperature. The primary and secondary antibodies were diluted in 2% normal goat serum in 1X PBS. The following primary antibodies were used: Rat anti-mouse CD31 IgG2a antibody (Endothelial cell marker, Invitrogen, cat # 14-0311-82: 1 μg/mL) and polyclonal rabbit anti-mouse VE-Cadherin IgG antibody (Abcam, cat # ab33168, 5 µg/mL) or polyclonal rabbit anti-mouse claudin-5 IgG antibody (Invitrogen, cat # 34–1600, 0.5 μg/mL). The following secondary antibodies were used: Goat anti-rat IgG (H+L) Alexa Fluor® 488 (Invitrogen, cat # A-11006) and goat anti-rabbit IgG (H+L) Alexa Fluor® 594 (Invitrogen, cat # A-11014) conjugated antibodies, both 4 µg/mL.
The sections were incubated with the primary and secondary antibodies for 1 hour at room temperature with two washes using 1X PBS between incubations. Sections were subsequently stained with 0.45 μM 4′, 6-diamidino-2-phenylindole (DAPI) for 5 min to detect nuclei and mounted with ProLong® Gold antifade mounting medium (Invitrogen, cat # P36930). Photomicrographs were taken using an Eclipse Ci-S Upright epifluorescent microscope with a D5-Qi2 camera (Nikon). Quantification of VE-Cadherin positive and claudin-5 positive endoneurial microvessels leukocytes per section was manually performed on merged photomicrographs generated at 400X magnification using the NIS-Elements AR software (Nikon). Data were expressed as the % of CD31+ endoneurial microvessels per mouse sciatic nerve, with data averaged between the adjacent sections from the same mouse prior to comparative analyses between crush-injured and Sham surgery nerves in the same mouse, between GDNF, WT, HET and CKO mice and the three different time points.
Statistical analyses
Investigator-blinded data analyses were performed for all parameters evaluated using the GraphPad Prism® 6 statistical program (GraphPad Software, Inc., La Jolla, CA). One- or two-tailed paired/unpaired Student‘s/Welch‘s t-test was used based on the Shapiro-Wilk test of normality (including measures of skew and kurtosis). Holm-Sidak‘s test was used for multiple variable comparisons. Means are displayed for each parameter evaluated, with variations of the mean depicted as standard errors. Statistical significance is defined as a p-value < 0.05.
Potential conflicts of interest
E.E.U. has received royalties from Springer Science + Business Media for an edited book on laboratory protocols that describes a flow-dependent human in vitro BNB assay (not used in this study). C.D., E.S.H., P.Z., X.O., X. d‘A.T., A.P. and J. L.-B. have nothing to disclose.
Acknowledgments
Special thanks to Lingling Guo, University of Alabama at Birmingham Small Animal Microsurgical Core Facility for assistance with mouse tail nerve injections and Elizabeth Weeks, EMLABS Inc. for expertise in electron microscopy.
Additional information
Funding
References
- American Diabetes A. Economic costs of diabetes in the U.S. in 2012. Diabetes Care. 2013;36:1033–46. doi:10.2337/dc12-2625. PMID:23468086.
- Sadosky A, Mardekian J, Parsons B, Hopps M, Bienen EJ, Markman J. Healthcare utilization and costs in diabetes relative to the clinical spectrum of painful diabetic peripheral neuropathy. J Diabetes Complications. 2015;29:212–7. doi:10.1016/j.jdiacomp.2014.10.013. PMID:25498300.
- Bennett GJ. Neuropathic pain: new insights, new interventions. Hosp Pract (1995). 1998;33:95–8, 101– 4, 7–10 passim. doi:10.3810/hp.1998.10.114.
- Feldman EL, Nave KA, Jensen TS, Bennett DL. New horizons in diabetic neuropathy: Mechanisms, bioenergetics, and pain. Neuron. 2017;93:1296–313. doi:10.1016/j.neuron.2017.02.005. PMID:28334605.
- Dworkin RH. An overview of neuropathic pain: syndromes, symptoms, signs, and several mechanisms. Clin J Pain. 2002;18:343–9. doi:10.1097/00002508-200211000-00001. PMID:12441827.
- Smith BH, Torrance N. Epidemiology of neuropathic pain and its impact on quality of life. Curr Pain Headache Rep. 2012;16:191–8. doi:10.1007/s11916-012-0256-0. PMID:22395856.
- Yawn BP, Wollan PC, Weingarten TN, Watson JC, Hooten WM, Melton LJ, 3rd. The prevalence of neuropathic pain: clinical evaluation compared with screening tools in a community population. Pain Med. 2009;10:586–93. doi:10.1111/j.1526-4637.2009.00588.x. PMID:20849570.
- Gordois A, Scuffham P, Shearer A, Oglesby A, Tobian JA. The health care costs of diabetic peripheral neuropathy in the US. Diabetes Care. 2003;26:1790–5. doi:10.2337/diacare.26.6.1790. PMID:12766111.
- Argoff CE, Cole BE, Fishbain DA, Irving GA. Diabetic peripheral neuropathic pain: clinical and quality-of-life issues. Mayo Clin Proc. 2006;81:S3–11. doi:10.1016/S0025-6196(11)61474-2. PMID:16608048.
- Frenzen PD. Economic cost of Guillain-Barre syndrome in the United States. Neurology. 2008;71:21–7. doi:10.1212/01.wnl.0000316393.54258.d1. PMID:18591502.
- Watson JC, Dyck PJ. Peripheral Neuropathy: A Practical Approach to Diagnosis and Symptom Management. Mayo Clin Proc. 2015;90:940–51. doi:10.1016/j.mayocp.2015.05.004. PMID:26141332.
- Callaghan BC, Price RS, Feldman EL. Distal Symmetric Polyneuropathy: A Review. JAMA. 2015;314:2172–81. doi:10.1001/jama.2015.13611. PMID:26599185.
- Noble J, Munro CA, Prasad VS, Midha R. Analysis of upper and lower extremity peripheral nerve injuries in a population of patients with multiple injuries. J Trauma. 1998;45:116–22. doi:10.1097/00005373-199807000-00025. PMID:9680023.
- Palispis WA, Gupta R. Surgical repair in humans after traumatic nerve injury provides limited functional neural regeneration in adults. Exp Neurol. 2017;290:106–14. doi:10.1016/j.expneurol.2017.01.009. PMID:28111229.
- Grinsell D, Keating CP. Peripheral nerve reconstruction after injury: a review of clinical and experimental therapies. Biomed Res Int. 2014;2014:698256. doi:10.1155/2014/698256. PMID:25276813.
- Bär KJ, Saldanha GJ, Kennedy AJ, Facer P, Birch R, Carlstedt T, Anand P. GDNF and its receptor component Ret in injured human nerves and dorsal root ganglia. Neuroreport. 1998;9:43–7. doi:10.1097/00001756-199801050-00009. PMID:9592045.
- Bennett DL, Michael GJ, Ramachandran N, Munson JB, Averill S, Yan Q, McMahon SB, Priestley JV. A distinct subgroup of small DRG cells express GDNF receptor components and GDNF is protective for these neurons after nerve injury. J Neurosci. 1998;18:3059–72. doi:10.1523/JNEUROSCI.18-08-03059.1998. PMID:9526023.
- Hammarberg H, Piehl F, Cullheim S, Fjell J, Hökfelt T, Fried K. GDNF mRNA in Schwann cells and DRG satellite cells after chronic sciatic nerve injury. Neuroreport. 1996;7:857–60. doi:10.1097/00001756-199603220-00004. PMID:8724660.
- Naveilhan P, ElShamy WM, Ernfors P. Differential regulation of mRNAs for GDNF and its receptors Ret and GDNFR alpha after sciatic nerve lesion in the mouse. Eur J Neurosci. 1997;9:1450–60. doi:10.1111/j.1460-9568.1997.tb01499.x. PMID:9240402.
- Chen ZY, Chai YF, Cao L, Lu CL, He C. Glial cell line-derived neurotrophic factor enhances axonal regeneration following sciatic nerve transection in adult rats. Brain Res. 2001;902:272–6. doi:10.1016/S0006-8993(01)02395-2. PMID:11384621.
- Trupp M, Ryden M, Jornvall H, Funakoshi H, Timmusk T, Arenas E, Ibáñez CF. Peripheral expression and biological activities of GDNF, a new neurotrophic factor for avian and mammalian peripheral neurons. J Cell Biol. 1995;130:137–48. doi:10.1083/jcb.130.1.137. PMID:7790368.
- Magill CK, Tuffaha SH, Yee A, Luciano JP, Hunter DA, Mackinnon SE, Borschel GH. The short- and long-term effects of Seprafilm on peripheral nerves: a histological and functional study. J Reconstr Microsurg. 2009;25:345–54. doi:10.1055/s-0029-1215526. PMID:19396746.
- Tannemaat MR, Eggers R, Hendriks WT, de Ruiter GC, van Heerikhuize JJ, Pool CW, Malessy MJ, Boer GJ, Verhaagen J. Differential effects of lentiviral vector-mediated overexpression of nerve growth factor and glial cell line-derived neurotrophic factor on regenerating sensory and motor axons in the transected peripheral nerve. Eur J Neurosci. 2008;28:1467–79. doi:10.1111/j.1460-9568.2008.06452.x. PMID:18973572.
- Jubran M, Widenfalk J. Repair of peripheral nerve transections with fibrin sealant containing neurotrophic factors. Exp Neurol. 2003;181:204–12. doi:10.1016/S0014-4886(03)00041-4. PMID:12781993.
- Hoke A, Cheng C, Zochodne DW. Expression of glial cell line-derived neurotrophic factor family of growth factors in peripheral nerve injury in rats. Neuroreport. 2000;11:1651–4. doi:10.1097/00001756-200006050-00011. PMID:10852218.
- Xu P, Rosen KM, Hedstrom K, Rey O, Guha S, Hart C, Corfas G. Nerve injury induces glial cell line-derived neurotrophic factor (GDNF) expression in Schwann cells through purinergic signaling and the PKC-PKD pathway. Glia. 2013;61:1029–40. doi:10.1002/glia.22491. PMID:23553603.
- Beck KD, Valverde J, Alexi T, Poulsen K, Moffat B, Vandlen RA, Rosenthal A, Hefti F. Mesencephalic dopaminergic neurons protected by GDNF from axotomy-induced degeneration in the adult brain. Nature. 1995;373:339–41. doi:10.1038/373339a0. PMID:7830767.
- Henderson CE, Phillips HS, Pollock RA, Davies AM, Lemeulle C, Armanini M, Simmons L, Moffet B, Vandlen RA, Simpson LC corrected to Simmons L, et al. GDNF: a potent survival factor for motoneurons present in peripheral nerve and muscle. Science. 1994;266:1062–4. doi:10.1126/science.7973664. PMID:7973664.
- Takahashi M. The GDNF/RET signaling pathway and human diseases. Cytokine Growth Factor Rev. 2001;12:361–73. doi:10.1016/S1359-6101(01)00012-0. PMID:11544105.
- Airaksinen MS, Saarma M. The GDNF family: signalling, biological functions and therapeutic value. Nat Rev Neurosci. 2002;3:383–94. doi:10.1038/nrn812. PMID:11988777.
- Jing S, Wen D, Yu Y, Holst PL, Luo Y, Fang M, Tamir R, Antonio L, Hu Z, Cupples R, et al. GDNF-induced activation of the ret protein tyrosine kinase is mediated by GDNFR-alpha, a novel receptor for GDNF. Cell. 1996;85:1113–24. doi:10.1016/S0092-8674(00)81311-2. PMID:8674117.
- Ibanez CF. Structure and physiology of the RET receptor tyrosine kinase. Cold Spring Harb Perspect Biol. 2017;18:477–494. doi:10.1101/cshperspect.a009134. PMID:23378586.
- Ibanez CF, Andressoo JO. Biology of GDNF and its receptors – Relevance for disorders of the central nervous system. Neurobiol Dis. 2017;97:80–9. doi:10.1016/j.nbd.2016.01.021. PMID:26829643.
- Allen SJ, Watson JJ, Shoemark DK, Barua NU, Patel NK. GDNF, NGF and BDNF as therapeutic options for neurodegeneration. Pharmacol Ther. 2013;138:155–75. doi:10.1016/j.pharmthera.2013.01.004. PMID:23348013.
- Natsume A, Wolfe D, Hu J, Huang S, Puskovic V, Glorioso JC, Fink DJ, Mata M. Enhanced functional recovery after proximal nerve root injury by vector-mediated gene transfer. Exp Neurol. 2003;184:878–86. doi:10.1016/S0014-4886(03)00334-0. PMID:14769380.
- Marconi S, Castiglione G, Turano E, Bissolotti G, Angiari S, Farinazzo A, Constantin G, Bedogni G, Bedogni A, Bonetti B. Human adipose-derived mesenchymal stem cells systemically injected promote peripheral nerve regeneration in the mouse model of sciatic crush. Tissue Eng Part A. 2012;18:1264–72. doi:10.1089/ten.tea.2011.0491. PMID:22332955.
- Hoke A, Gordon T, Zochodne DW, Sulaiman OA. A decline in glial cell-line-derived neurotrophic factor expression is associated with impaired regeneration after long-term Schwann cell denervation. Exp Neurol. 2002;173:77–85. doi:10.1006/exnr.2001.7826. PMID:11771940.
- Mizisin AP, Weerasuriya A. Homeostatic regulation of the endoneurial microenvironment during development, aging and in response to trauma, disease and toxic insult. Acta neuropathologica. 2011;121:291–312. doi:10.1007/s00401-010-0783-x. PMID:21136068.
- Reina MA, Lopez A, Villanueva MC, de Andres JA, Leon GI. [Morphology of peripheral nerves, their sheaths, and their vascularization]. Rev Esp Anestesiol Reanim. 2000;47:464–75. PMID:11171467.
- Reina MA, Lopez A, Villanueva MC, De Andres JA, Maches F. [The blood-nerve barrier in peripheral nerves]. Rev Esp Anestesiol Reanim. 2003;50:80–6. PMID:12712870.
- Ubogu EE. The molecular and biophysical characterization of the human blood-nerve barrier: current concepts. J Vasc Res. 2013;50:289–303. doi:10.1159/000353293. PMID:23839247.
- Aird WC. Phenotypic heterogeneity of the endothelium: I. Structure, function, and mechanisms. Circulation Res. 2007;100:158–73. doi:10.1161/01.RES.0000255691.76142.4a. PMID:17272818.
- Aird WC. Phenotypic heterogeneity of the endothelium: II. Representative vascular beds. Circulation res. 2007;100:174–90. doi:10.1161/01.RES.0000255690.03436.ae. PMID:17272819.
- Yano K, Gale D, Massberg S, Cheruvu PK, Monahan-Earley R, Morgan ES, Haig D, von Andrian UH, Dvorak AM, Aird WC. Phenotypic heterogeneity is an evolutionarily conserved feature of the endothelium. Blood. 2007;109:613–5. doi:10.1182/blood-2006-05-026401. PMID:16990601.
- Potente M, Makinen T. Vascular heterogeneity and specialization in development and disease. Nat Rev Mol Cell Biol. 2017. doi:10.1038/nrm.2017.36. PMID:28537573.
- Reddy CL, Yosef N, Ubogu EE. VEGF-A165 potently induces human blood-nerve barrier endothelial cell proliferation, angiogenesis, and wound healing in vitro. Cellular Mol Neurobiol. 2013;33:789–801. doi:10.1007/s10571-013-9946-3.
- Yosef N, Ubogu EE. GDNF restores human blood-nerve barrier function via RET tyrosine kinase-mediated cytoskeletal reorganization. Microvasc Res. 2012;83:298–310. doi:10.1016/j.mvr.2012.01.005. PMID:22326552.
- Palladino SP, Helton ES, Jain P, Dong C, Crowley MR, Crossman DK, Ubogu EE. The Human Blood-Nerve Barrier Transcriptome. Sci Rep. 2017;7:17477. doi:10.1038/s41598-017-17475-y. PMID:29234067.
- Shimizu F, Sano Y, Saito K, Abe MA, Maeda T, Haruki H, Kanda T. Pericyte-derived glial cell line-derived neurotrophic factor increase the expression of claudin-5 in the blood-brain barrier and the blood-nerve barrier. Neurochem Res. 2012;37:401–9. doi:10.1007/s11064-011-0626-8. PMID:22002662.
- Igarashi Y, Chiba H, Utsumi H, Miyajima H, Ishizaki T, Gotoh T, Kuwahara K, Tobioka H, Satoh M, Mori M, et al. Expression of receptors for glial cell line-derived neurotrophic factor (GDNF) and neurturin in the inner blood-retinal barrier of rats. Cell Struct Funct. 2000;25:237–41. doi:10.1247/csf.25.237. PMID:11129793.
- Igarashi Y, Utsumi H, Chiba H, Yamada-Sasamori Y, Tobioka H, Kamimura Y, Furuuchi K, Kokai Y, Nakagawa T, Mori M, et al. Glial cell line-derived neurotrophic factor induces barrier function of endothelial cells forming the blood-brain barrier. Biochem Biophys Res Communications. 1999;261:108–12. doi:10.1006/bbrc.1999.0992.
- Kamimura Y, Chiba H, Utsumi H, Gotoh T, Tobioka H, Sawada N. Barrier function of microvessels and roles of glial cell line-derived neurotrophic factor in the rat testis. Med Electron Microsc. 2002;35:139–45. doi:10.1007/s007950200017. PMID:12353134.
- Meir M, Flemming S, Burkard N, Bergauer L, Metzger M, Germer CT, Schlegel N. Glial cell line-derived neurotrophic factor promotes barrier maturation and wound healing in intestinal epithelial cells in vitro. Am J Physiol Gastrointest Liver Physiol. 2015;309:G613–24. doi:10.1152/ajpgi.00357.2014. PMID:26294673.
- Brun P, Giron MC, Qesari M, Porzionato A, Caputi V, Zoppellaro C, Banzato S, Grillo AR, Spagnol L, De Caro R, et al. Toll-like receptor 2 regulates intestinal inflammation by controlling integrity of the enteric nervous system. Gastroenterology. 2013;145:1323–33. doi:10.1053/j.gastro.2013.08.047. PMID:23994200.
- Steinkamp M, Geerling I, Seufferlein T, von Boyen G, Egger B, Grossmann J, Ludwig L, Adler G, Reinshagen M. Glial-derived neurotrophic factor regulates apoptosis in colonic epithelial cells. Gastroenterology. 2003;124:1748–57. doi:10.1016/S0016-5085(03)00404-9. PMID:12806607.
- Meir M, Flemming S, Burkard N, Wagner J, Germer CT, Schlegel N. The glial cell-line derived neurotrophic factor: a novel regulator of intestinal barrier function in health and disease. Am J Physiol Gastrointest Liver Physiol. 2016;310:G1118–23. doi:10.1152/ajpgi.00125.2016. PMID:27151942.
- Pascual A, Hidalgo-Figueroa M, Piruat JI, Pintado CO, Gomez-Diaz R, Lopez-Barneo J. Absolute requirement of GDNF for adult catecholaminergic neuron survival. Nat Neurosci. 2008;11:755–61. doi:10.1038/nn.2136. PMID:18536709.
- Bridge PM, Ball DJ, Mackinnon SE, Nakao Y, Brandt K, Hunter DA, Hertl C. Nerve crush injuries–a model for axonotmesis. Exp Neurol. 1994;127:284–90. doi:10.1006/exnr.1994.1104. PMID:8033968.
- Ueno M, Sakamoto H, Kanenishi K, Onodera M, Akiguchi I, Hosokawa M. Ultrastructural and permeability features of microvessels in the hippocampus, cerebellum and pons of senescence-accelerated mice (SAM). Neurobiol Aging. 2001;22:469–78. doi:10.1016/S0197-4580(01)00213-5. PMID:11378254.
- Hashizume K, Black KL. Increased endothelial vesicular transport correlates with increased blood-tumor barrier permeability induced by bradykinin and leukotriene C4. J Neuropathol Exp Neurol. 2002;61:725–35. doi:10.1093/jnen/61.8.725. PMID:12152787.
- Martins T, Burgoyne T, Kenny BA, Hudson N, Futter CE, Ambrosio AF, Silva AP, Greenwood J, Turowski P. Methamphetamine-induced nitric oxide promotes vesicular transport in blood-brain barrier endothelial cells. Neuropharmacology. 2013;65:74–82. doi:10.1016/j.neuropharm.2012.08.021. PMID:22960442.
- Ubogu EE. Inflammatory neuropathies: pathology, molecular markers and targets for specific therapeutic intervention. Acta Neuropathol. 2015;130:445–68. doi:10.1007/s00401-015-1466-4. PMID:26264608.
- Xia RH, Yosef N, Ubogu EE. Clinical, electrophysiological and pathologic correlations in a severe murine experimental autoimmune neuritis model of Guillain-Barre syndrome. J Neuroimmunol. 2010;219:54–63. doi:10.1016/j.jneuroim.2009.11.019. PMID:20034679.
- Ubogu EE. Translational strategies in peripheral neuroinflammation and neurovascular repair. Transl Neurosci. 2012;3:373–83. doi:10.2478/s13380-012-0039-4. PMID:23638307.
- Peltonen S, Alanne M, Peltonen J. Barriers of the peripheral nerve. Tissue Barriers. 2013;1:e24956. doi:10.4161/tisb.24956. PMID:24665400.
- Mukhamedshina YO, Gilazieva ZE, Arkhipova SS, Galieva LR, Garanina EE, Shulman AA, Yafarova GG, Chelyshev YA, Shamsutdinova NV, Rizvanov AA. Electrophysiological, Morphological, and Ultrastructural Features of the Injured Spinal Cord Tissue after Transplantation of Human Umbilical Cord Blood Mononuclear Cells Genetically Modified with the VEGF and GDNF Genes. Neural Plast. 2017;2017:9857918. doi:10.1155/2017/9857918. PMID:28421147.
- Yuan F, Yosef N, Lakshmana Reddy C, Huang A, Chiang SC, Tithi HR, Ubogu EE. CCR2 gene deletion and pharmacologic blockade ameliorate a severe murine experimental autoimmune neuritis model of Guillain-Barre syndrome. PLoS One. 2014;9:e90463. doi:10.1371/journal.pone.0090463. PMID:24632828.
- Xia RH, Yosef N, Ubogu EE. Selective expression and cellular localization of pro-inflammatory chemokine ligand/receptor pairs in the sciatic nerves of a severe murine experimental autoimmune neuritis model of Guillain-Barre syndrome. Neuropathol Appl Neurobiol. 2010;36:388–98. doi:10.1111/j.1365-2990.2010.01092.x. PMID:20500551.
- Smith CE, Atchabahian A, Mackinnon SE, Hunter DA. Development of the blood-nerve barrier in neonatal rats. Microsurgery. 2001;21:290–7. doi:10.1002/micr.1055. PMID:11754428.
- Kaya M, Ahishali B. Assessment of permeability in barrier type of endothelium in brain using tracers: Evans blue, sodium fluorescein, and horseradish peroxidase. Methods Mol Biol. 2011;763:369–82. doi:10.1007/978-1-61779-191-8_25. PMID:21874465.
- Nico B, Frigeri A, Nicchia GP, Corsi P, Ribatti D, Quondamatteo F, Herken R, Girolamo F, Marzullo A, Svelto M, et al. Severe alterations of endothelial and glial cells in the blood-brain barrier of dystrophic mdx mice. Glia. 2003;42:235–51. doi:10.1002/glia.10216. PMID:12673830.
- Ma N, Hunt NH, Madigan MC, Chan-Ling T. Correlation between enhanced vascular permeability, up-regulation of cellular adhesion molecules and monocyte adhesion to the endothelium in the retina during the development of fatal murine cerebral malaria. Am J Pathol. 1996;149:1745–62. PMID:8909263.
- Saadoun S, Tait MJ, Reza A, Davies DC, Bell BA, Verkman AS, Papadopoulos MC. AQP4 gene deletion in mice does not alter blood-brain barrier integrity or brain morphology. Neuroscience. 2009;161:764–72. doi:10.1016/j.neuroscience.2009.03.069. PMID:19345723.
- Dong C, Greathouse KM, Beacham RL, Palladino SP, Helton ES, Ubogu EE. Fibronectin connecting segment-1 peptide inhibits pathogenic leukocyte trafficking and inflammatory demyelination in experimental models of chronic inflammatory demyelinating polyradiculoneuropathy. Exp Neurol. 2017;292:35–45. doi:10.1016/j.expneurol.2017.02.012. PMID:28215575.
- Dong C, Palladino SP, Helton ES, Ubogu EE. The pathogenic relevance of alphaM-integrin in Guillain-Barre syndrome. Acta Neuropathol. 2016;132:739–52. doi:10.1007/s00401-016-1599-0. PMID:27460017.
- Ubogu EE, Yosef N, Xia RH, Sheikh KA. Behavioral, electrophysiological, and histopathological characterization of a severe murine chronic demyelinating polyneuritis model. J Peripheral Nervous System. 2012;17:53–61. doi:10.1111/j.1529-8027.2012.00375.x.