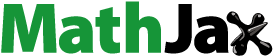
Abstract
Objective: Colorectal cancer (CRC) ranked second in females and third in males among all type of cancers diagnosed. About 1.4 million cases took place with 693,900 deaths in 2012. It can occur either in colon or rectum. Thus, we aimed to develop and optimize oxaliplatin (OP) loaded solid lipid nanoparticles (SLNs).
Materials and methods: SLNs containing tristearin, 1,2-distearoyl-sn-glycero-3-phosphoethanolamine (DSPE), Lipoid S75, and Tween 80 was developed. Box–Behnken design was applied for optimization of SLNs and optimized formulation was selected for conjugation with folic acid (FA). Optimized formulations were evaluated for various physiochemical parameters viz., particle size (PS), zeta potential, %entrapment efficiency (EE), morphology, X-ray diffraction (XRD), and differential scanning calorimetry (DSC).
Results and discussion: OP loaded uncoupled SLNs (OPSLNs) and OP loaded FA coupled SLNs (OPSLNFs) formulations revealed good EE, 49.2 ± 0.38% and 43.5 ± 0.59%, respectively and small PS, 146.2 ± 4.4 nm, and 158.8 ± 5.6 nm, respectively. XRD pattern and DSC results confirmed that OP was uniformly distributed in amorphous form within SLNs. In vitro drug release study of OPSLNs and OPSLNFs formulation revealed sustained drug release pattern of OP for up to 6 d. Anticancer activity on HT-29 cell line indicated the highest potency of OPSLNFs as compared to OPSLNs and OP solution.
Conclusion: The present work illustrated the higher sensitivity of HT-29 cells to the drug entrapped in OPSLNFs as compared to OPSLNs and OP solution. Hence, this novel strategy might be a promising approach for the management of CRC.
Introduction
Scientists focused on solid lipid nanoparticles (SLNs) in the middle of 1990s, as a substitute to liposomes, polymeric nanoparticles, and nanoemulsion [Citation1]. Despite some advantages, i.e. controlled and sustained drug release, polymeric nanoparticles are associated with acute and chronic toxicity [Citation2], which insist scientists to shift towards biocompatible lipid-based nanocarriers. SLNs are synthesized from lipid molecules which found in solid states at room temperature. They combine the advantage of several other novel carrier systems such as physical stability, controlled drug release, and excellent biocompatibility. In addition, SLN overcomes few problems associated with other carrier systems, i.e. reduced drug leaching, good membrane stability, and feasibility of large scale production [Citation3]. In recent studies, SLNs have been employed with great potential in the targeting of several types of cancer [Citation4–6].
Active targeting of anticancer agents exploits specific interactions among receptors available in abundant quantity on the surface of cancer cells and targeting ligand attached to the polymer backbone. This approach, therefore, undertakes advantage of the effect i.e. enhanced permeability and retention (EPR) effect and further enhances the therapeutic index via receptor-mediated uptake by the targeting cancer cells. Vascular pore size up to 400 nm has been reported in the LS174T tumour of human colon adenocarcinoma [Citation7]. Hence, nanoparticles existing below in particle size (PS) (i.e. < 400 nm) can be successfully targeted via the passive targeting owing to EPR effect. Folate receptors are extremely over expressed on the surface of cancer cells of epithelial origin such as ovarian, breast, colorectal carcinomas [Citation8], cervical [Citation9], and choroids plexus [Citation10]. Therefore, colorectal cancer (CRC) tumour with folate receptors will offer high affinity for folic acid (FA) (vitamin B9) and can be utilized as a targeting ligand for efficient delivery of anticancer agents to tumour cells. Thus, folate grafted SLNs bearing anticancer agent could be a highly efficient approach for specific targeting of all anticancer drugs with narrow therapeutic window.
Oxaliplatin {cis-[(1 R,2 R)-1,2-cyclohexanediamine-N, N′]-oxalatoplatinum (II), OP} is one of the widely used third-generation chemotherapeutic agent of the family, cisplatin [cis-Pt(NH3)2Cl2]. OP is available as the treatment regimen for the management of CRC after approval granted by the European Union and the United States, in 1999 and in 2002, respectively [Citation11]. OP is now accepted as first-line chemotherapy regimen in combination with another anticancer drug i.e. 5-fluorouracil for the treatment of CRC. Despite its improved tolerability over other platinum (Pt) compounds (i.e. carboplatin and cisplatin), OP is associated with some side effects like cumulative peripheral distal neurotoxicity and acute dysesthesias, which restrict dose size. Systemic chemotherapy using OP with other anticancer drugs or alone is effective, but produce adverse effects, which often result into patient non-compliance and increased failure rates [Citation12]. To overcome these associated problems, it would be advantageous to deliver the least amount of anticancer agents directly into the tumour, thereby enhancing the prospects for the effective treatment [Citation13].
Hence, it was attempted to develop FA grafted SLNs bearing OP for targeted delivery to CRC. Tristearin is a biocompatible and biodegradable solid lipid, which has good stability at physiological temperature [Citation14,Citation15]. Therefore, it was selected to prepare lipid matrix of SLNs. 1,2-distearoyl-sn-glycero-3-phosphoethanolamine (DSPE) was employed for conjugation of FA via its carboxyl group to its amine group. Lipoid S75 and Tween 80 were used as surfactant and co-surfactant, respectively. The formulation was optimized using Box–Behnken design (BBD) and then characterized for the PS, zeta potential (ZP), X-ray diffraction (XRD), differential scanning calorimetry (DSC), and in vitro drug release study. Cell cytotoxicity and stability study was also performed of the developed formulations.
Materials and methods
Materials
The drug OP was received as a gift sample from M/s Khandelwal Laboratories Ltd. (Mumbai, India). Lipoid S75 and DSPE were gifted from Lipoid (Ludwigshafen, Germany). Tristearin and dialysis membrane (MWCO 12–14 kDa) were procured from HiMedia (Mumbai, India). 1-Ethyl-3-(3-dimethyl-aminopropyl) carbodiimide (EDC), N-hydroxysulfosuccinimide sodium salt (Sulfo-NHS), anhydrous dimethyl sulfoxide (DMSO), Sephadex G-50, Tween 80, FA, and 0.22 µm nylon membrane filters (Millipore, Billerica, MA) were purchased from Sigma-Aldrich (St. Louis, MO). All other chemicals and solvents were of analytical grade and procured from HiMedia.
Methods
Preparation and optimization of SLNs
SLNs were prepared using solvent diffusion method with minor modifications () [Citation16]. Briefly, organic phase was prepared by dissolving various quantities of different components such as Lipoid S75 (20, 40, and 60 mg) in a mixture of tristearin and DSPE in ratio 10:1 (100, 200, and 300 mg) in ethanol (10 ml) with heating at 70 °C in a closed container. Another, 20 ml of the aqueous phase was prepared by adding Tween 80 (0.5%) and OP (50, 75, and 100 mg) in double-distilled water (DDW) and kept to the same temperature. Then hot lipid phase was added into the aqueous phase at 70 °C under continuous magnetic stirring at 1200 rpm to develop coarse emulsion. The prepared coarse emulsion was ultra-sonicated using bath sonicator (Analytical Technologies Ltd., Baroda, India) for 10 min. The resultant formulation was ice cold to form OP loaded SLNs (OPSLNs) dispersion. Then it was filtered through 0.22 µm filter to remove impurities from the dispersion. The effect of drug content (DC), surfactant/cosurfactant (S/CoS), and lipid concentration (LC) on PS, % entrapment efficiency (EE), and % drug loading (DL) was determined using BBD method.
Preparation of optimized SLNs
Finally, SLNs were prepared using optimized parameters. The organic phase (12 mg/ml) was prepared by dissolving tristearin, Lipoid S75, and DSPE (9:2:1, w/w) in 20 ml ethanol with heating at 70 °C in a closed container. Meanwhile, the aqueous phase containing Tween 80 (0.5%) and OP (3.75 mg/ml) was prepared using DDW and kept to the same temperature. The organic phase was injected into the aqueous phase at 70 °C under magnetic stirring at 1200 rpm to form a coarse emulsion. Then coarse emulsion was sonicated using bath sonicator for 10 min. The resulting formulation was ice cold to form an OPSLNs dispersion. Then it was filtered via 0.22 µm filter to remove impurities from the dispersion. Blank SLNs were also synthesized using same procedure devoid of OP.
Conjugation of FA to SLN
Conjugation of FA with SLNs was performed via post insertion technique as described by Gupta et al. [Citation17], with suitable modifications (). The FA was conjugated by its carboxyl group (activated with Sulfo-NHS) to the amine of DSPE of preformed SLNs using EDC as the coupling agent. Briefly, FA was activated using Sulfo-NHS and EDC in anhydrous DMSO. Then Sulfo-NHS activated FA was added drop-wise into the dispersion of SLNs (SLN: FA, 90:10, w/w) in DDW. Thus, obtained mixture was vortexed for 3 h followed by incubation for 2 h at room temperature. An excess amount of FA was removed after passing dispersion through the column of Sephadex G-50.
SLNs characterization
Assessment of PS, polydispersity index (PDI), and ZP
The average PS, PDI, and ZP of SLN formulations were determined by the photon correlation spectroscopy via Malvern Zetasizer (Malvern, Worcestershire, UK) at 25 °C. Samples were measured at refractive index 1.33 after diluting with phosphate buffer (pH 7.4). All studies were run at least in triplicate.
Shape and surface morphology
Developed SLNs were investigated for shape and size using transmission electron microscopy (TEM) (model TECNAI 200 Kv TEM, Fei Electron Optics, Japan). SLNs were dried on Cu grid and then stained (−ve) using 1% uranyl acetate. Prepared grids were observed after 1 h under the microscope at accelerating voltage (200 kV). The surface of lyophilized SLN was investigated using scanning electron microscopy (SEM) (Leo 435 VP, Carl Zeiss NTS GmbH, Oberkochen, Germany). The lyophilized SLNs were fixed on the brass stub via the paired adhesive tape. Then stub was kept in an ion sputter coater for gold coating. The sample was viewed after fixing stub in the sample holder at high vacuum.
X-ray diffraction (XRD) study
The internal crystallization structure of samples was investigated using small angle XRD patterns acquired at room temperature. X-ray diffractometer (Rigaku Smart Lab X-ray diffractometer, Tokyo, Japan) was run at 40 kV and 45 mA to produce Copper Kα X-ray.
Differential scanning calorimetry (DSC) study
DSC examination of samples was carried out using DSC-60 (Shimadzu, Japan). About 5 mg of OP, bulk tristearin, a physical mixture of OP and tristearin, and SLN with or without OP were weighed, crimped into an aluminium pan, and scanned with the heating rate at 10 °C/min. Baseline optimization was run before each experiment.
Determination of EE and DL
EE and drug loading capacity of OP in SLN formulations were assessed after separating unentrapped drug through Sephadex G-50 minicolumn via centrifugation technique [Citation18]. Sephadex G-50 (1.0 g) was allowed to swell in 0.9% NaCl at room temperature, with occasional shaking, for at least 5 h, after which the gel was formed and stored at 4 °C. Minicolumn was prepared by placing Whatman paper (# 41) pads on the lowermost of the barrels of 1.0 ml syringe, which was filled with the gel. Excess water was removed using centrifugation (REMI, India, centrifuge) at 3000 rpm for 3 min. SLNs (200 µl) were applied drop wise to the centre of the column and the column was centrifuged at 2000 rpm for 3 min. After centrifugation, the clear supernatant was analyzed for DC via UV spectrophotometer (UV-1800, Shimadzu, Japan) at 247 nm:
In vitro drug release
The drug release from OPSLNs and coupled SLNs (OPSLNFs) were performed in PBS 7.4 using dialysis bag (MWCO, 12–14 kDa) method. The dialysis bag retains SLNs and simultaneously allows the free drug to go into dissolution media. Further, the dialysis bags were saturated using DDW for 24 h, before use. Two millilitres of OPSLNs and OPSLNFs was transferred into the bag tied with the thread at one end. Then, bag was placed in a beaker and 50 ml of fluid was added. The beaker was then placed on a magnetic stirrer at 37 °C with 100 rpm. After different time intervals, i.e. 0.5, 1, 2, 3, 4, 8, 24 h, and then up to 144 h (at 24 h intervals), the 1 ml sample was taken out from the beaker and replaced with fresh dialysis medium to maintain sink condition. The filtrate was then analyzed by UV spectroscopy.
Cytotoxicity potential
Human HT-29 cells were cultured in RPMI 1640 medium and supplemented using 2 mm L-glutamine, 10% foetal bovine serum (FBS), 100 IU/ml penicillin and 100 µg/ml streptomycin serum at 37 °C in a 100% relative humidified atmosphere of 5% CO2. Cell viability study was performed to examine the effect of OPSLNs and OPSLNFs on the viability of HT-29 cell line. Briefly, the cell line was grown in RPMI 1640 medium added with 2 mm L-glutamine cells and 10% FBS. 96-well plates were used to inoculate the cells at 100 µl density. After inoculation, the plates were incubated at 37 °C in 95% air, 5% CO2, and 100% relative humidity, for 24 h to ensure proper attachment prior to addition of formulations. OP, OPSLNs and OPSLNFs were added in different concentrations (10, 20, 40 and 80 µg ml−1) to the well plates after replacing the culture medium. Cell viability was observed for 48 h. The culture without drug or any formulation was treated as a negative (−ve) control for cell proliferation. Adriamycin (1 mm) treated cells were considered as positive (+ve) control. The assay was terminated via the addition of cold trichloroacetic acid (TCA). In situ, fixation of cells was done after adding 50 µl of cold 30% (w/v) TCA to get a resultant concentration of 10% TCA and incubated at 4 °C for 60 min. After discarding supernatant, the well plates were cleaned using tap water for five times before air dried. Fifty microlitres sulforhodamine B solution (0.4%, w/v in 1% acetic acid) was injected into every well. Then, plates were incubated for 20 min at room temperature. Free dye was recovered after staining, and the remaining dye was removed after washing five times with 1% acetic acid. Afterward, the plates were dried in air. Further, trizma base (10 mm) was used to elute bound stain, and then absorbance was taken at wavelength 540 nm and 690 nm (reference wavelength) using the plate reader. All studies were repeated in triplicates. Based on a plate-by-plate, %growth was determined for test wells relative to control wells. Per cent growth was expressed in percentage of control.
Stability study
The stability of OPSLNs and OPSLNFs was determined by measuring drug retaining potential at different temperature conditions, i.e. 4 ± 1 °C and 25 ± 1 °C for the period of 6 months. Both SLN formulations were flushed with nitrogen and stored in 10 ml transparent vial (Merck, Kenilworth, NJ). Samples were withdrawn after 0, 1, 2, 3, and 6 months and analyzed for DC and PS.
Statistical analysis
Results for each experiment were stated as mean ± SD. The statistical analysis was calculated using one-way analysis of variance (ANOVA). A probability level of p < .05 was considered significant.
Results and discussion
SLNs protect trapped drug from proteolytic degradation and offer a selective intracellular targeting [Citation19]. SLN can load both lipophilic as well as hydrophilic drug and are stable morphologically and organoleptically for 1 year from its production [Citation20]. SLNs are synthesized using physiologically accepted lipids, therefore decreases menace of toxicity. Tristearin is a well-recognized solid lipid and has been employed to produce SLN to deliver drug via oral, topical, and parenteral route [Citation21–23].
Optimization using BBD
SLNs were produced by solvent diffusion method and optimized using 3-factors, 3-levels BBD in Design-Expert software (trial version 10.0.1.0, Stat-Ease Inc., Minneapolis, MN). Small PS, high %EE, and high %DL was considered for optimization of SLN formulation. The independent variables; OP (X1), lipids ratio (tristearin/DSPE, 10:1) (X2), and the ratio of S/CoS (Lipoid S75: Tween 80) (X3) with their low, medium, and high levels were selected to prepare 17 formulations. The dependent variables were PS (Y1), % EE (Y2), and % DL (Y3) with constraints applied to the formulation of SLN (). The S/CoS tested in this study are Lipoid S75 and Tween 80, respectively. Based on preliminary studies, the quantity of different components was selected; OP ranging from 50 mg to 100 mg; tristearin/DSPE (10:1) ranging from 100 mg to 300 mg; and Lipoid S75/Tween 80 ratio ranging from 1:5 to 3:5 (w/w). Further, from the optimization study, the values of three dependent variables were ranged from 197.83 ± 9.43 nm to 144.12 ± 5.46 nm for PS, 49.87 ± 4.19% to 14.28 ± 1.43% for EE, and 26.41 ± 4.33% to 10.49 ± 0.87% for DL. The quadratic model was found best-fitted for all the dependent variables (responses) studied, i.e. PS (nm), %EE, and %DL. The quadratic equations obtained for various responses are:
(1)
(1)
(2)
(2)
(3)
(3)
Table 1. Levels of variables in BBD.
The positive sign for the numeric values of different factor shows a synergistic effect, i.e. improved response, and vice-versa. The lack-of-fit was not significant in all the cases, at 95% confidence level. However, all the remaining parameters were significant (p < .05).
Response-surface plots
A total of 17 experimental runs were generated for three-levels and three-factors using the BBD. summarize complete detail of experimental runs related to dependent and independent variables. The three-dimensional response-surface plots were produced to investigate the pattern of interaction between dependent and independent variables. The results of various statistical parameters such as degree of freedom, multiple correlation coefficients (R2), p values, F-value, standard deviation (SD), coefficient of variation (CV), mean square (MS), and predicted residual sum of squares (SS) values are summarized in . The response-fitting values indicated optimized parameters of SLNs, which exhibit high %EE and %DL, and small mean PS in term of coded values at the concentration of OP (0.26), lipid (−0.16), and S/CoS ratio (−0.10). The surface plot showing the effect of various variables on the PS, %EE, and %DL is shown in . The mean PS was observed highest (197.83 ± 9.43 nm) in batch F6 at mid-level of lipids, low-level of surfactants, and high-level of OP and lowest (144.12 ± 5.46 nm) in batch F1 at mid-levels of all variables (). PS (Y1) was significantly influenced by all factors viz., X1, X2, X3, X12, X22, X32, X1X2, X1X3, and X2X3. EE was found highest (49.87 ± 4.19%) in batch F8 at mid-levels of lipids, drug, and S/CoS (2:5) and lowest (14.28 ± 1.43%) in the batch F3 at mid-level of lipids, high level of surfactants and low-level of drug (). %EE (Y2) was significantly (p < .05) influenced by X1, X3, X12, X22, X32, X1X3, and X2X3. DL was found highest (26.41 ± 4.33%) in batch F13 at higher levels of lipids and drug, mid-level of S/CoS and lowest (10.49 ± 0.87%) in the batch F3 at mid-level of lipids, high level of S/CoS and low-level of drug (). Response Y3 was significantly influenced by X1, X3, X22, X32, and X2X3.
Table 2. Observed responses in the BBD for independent variables (mean ± SD).
Table 3. Summary of the results of regression analysis for responses and ANOVA for %EE, %DL and mean PS.
Effect of S/CoS ratio on the PS, EE, and DL
The S/CoS ratios exhibited a predominant effect on the PS, %EE, and %DL. A higher ratio decreases the surface tension, which prevented agglomeration of the particles, and resulted in a decrease in PS. This decrease in PS with an increase in the ratio was seen up to the optimal value (2:5), and after that, a further increase in the S/CoS ratio produced an inverse effect, i.e. an increase in the mean PS. This shows that increase in S/CoS ratio up to an optimal level reduces the PS significantly. In the case of the %EE and %DL, increase in %EE and %DL was noticed with the increase in the ratio of S/CoS up to the optimal ratio (2:5), and after that, further, increase in the S/CoS ratio resulted to an inverse effect. This might be due to solubilization of drug owing to increased S/CoS ratio up to the optimum value ().
Effect of LC on the PS, EE, and DL
LC also produced a significant effect on the PS, %EE, and %DL. The response-surface plot () shows that the mean PS increased in all the cases with the increase in LC from 100 mg to 300 mg. The SLN dispersions containing highest LC, comparatively, showed largest PS, over the dispersions with low LC. The %EE and %DL also varied with an increase in LC. Initially, %EE and %DL was increased upon an increase in LC, this might be due to the solubilization of free drug present in dispersion by the lipid, afterwards, it decreased upon a further increase in LC. Reduction of PS was observed in diluted dispersions comprising low lipid, due to uniform distribution of sonication energy. It is reported elsewhere that upon an increase in LC led to larger sized particles along with the broader PS distribution [Citation24,Citation25].
Effect of DC on PS, EE, and DL
There was the remarkable and significant difference in the PS of SLNs prepared with different DC ranging from 0.25 to 0.50%. Initially, PS decreased significantly with an increase in DC, but later, at higher values of DC (above 0.375%), the PS increased, this may be owing to the presence of a higher amount of DC that decreased the effect of surfactants. However, %EE and %DL was found increasing with the increase in DC all the time during experiments ().
Conjugation of FA to SLN
Conjugation was carried out in between carboxyl group (–COOH) of FA and primary amine (–NH2) group of DSPE available on the surface of SLNs in the presence of Sulfo-NHS (COOH activating agent) and EDC as a coupling agent. FTIR studies were performed to confirm the coupling of the conjugated SLNs (). IR spectra of uncoupled SLNs exhibited a broad stretch peak at 3600–3100 cm−1, which confirmed free ammonium ion (NH3+) on the surface of SLNs. Coupled SLNs showed a peak at 1640 cm−1 (stretch), which is characteristic of C = O amide bond. The formation of primary N-H (stretch) amide bond was confirmed by the presence of peaks at 3354 cm−1 (asymmetric) and 3170 cm−1 (symmetric).
Assessment of PS, PDI, and ZP
Optimized method was used to prepare SLN formulation and then they were characterized by different parameters. represents PS, PDI, and ZP. PS of OPSLNs and OPSLNFs was found to be 146.2 ± 4.4 nm and 158.8 ± 5.6 nm, respectively. The significant increase in the PS of OPSLNFs was observed as compared to OPSLNs, this might be due to the conjugation of FA on the surface of SLN. Further, increase in PS also enhanced PDI value of OPSLNs (0.211 ± 0.02) and OPSLNFs (0.241 ± 0.03). ZP is essential to determine the stability of the colloidal dispersion. ZP of OPSLNs and OPSLNFs formulations were observed to be −22.6 ± 1.1 mV and −28.4 ± 1.6 mV, respectively. Obtained values of ZP suggested good stability of the formulations owing to their high repulsive force, which hinders aggregation of particles [Citation26].
Determination of EE and DL
Hydrophilic drugs exhibit less EE in SLN as compared to lipophilic drugs [Citation27,Citation28]. The EE of OPSLNs and OPSLNFs was observed to be 49.2 ± 0.38% and 43.5 ± 0.59%, respectively. In addition, DL capacity of OPSLNs and OPSLNFs was found to be 26.9 ± 2.2% and 24.6 ± 2.7%, respectively. OPSLNFs showed less %EE and %DL over OPSLNs. The decrease in %EE and %DL values of OPSLNFs was observed as compared to OPSLNs (). The %EE and %DL of OPSLNs were found significantly higher than OPSLNFs, and it might be due to dissolution and subsequent loss of OP from the outer stratum or adsorbed on the surface of SLNs in DDW, which was used as a medium for conjugation of SLNs. Our findings are well supported by previous investigations [Citation6].
Shape and surface morphology
SEM and TEM analysis of OPSLNs and OPSLNFs formulation was performed to assess the shape and surface morphology. SEM photograph advocated spherical shape and smooth surface of both formulations (). TEM analysis provides morphological information of the nanoparticles (). Images showed spherical shape of SLNs with size approximately 140–160 nm, which was also correlated with PS obtained from Zetasizer ().
XRD studies
shows XRD pattern of pure OP drug, bulk tristearin, a physical mixture of tristearin and drug, blank SLN, and OPSLNs. X-ray pattern revealed a significant difference between the diffraction of bulk tristearin, blank SLN, and OPSLNs. Tristearin showed characteristic high-energy diffraction peaks at 2θ values between 18° and 25° with a sharp narrow peak at 21.25°, while SLNs gave broader peak with low intensity at the same degree. This suggested partial recrystallization of tristearin in SLN. However, the complete absence of characteristic sharp peak of pure OP at 20.38° suggests that the OP is present in amorphous form in OPSLNs. These findings clearly suggest that the OP is molecularly dispersed with the lipids in SLN.
DSC studies
The DSC study () gave analogous results to the XRD study. Polymorphic change in lipid can be observed during SLN preparation, which can be assessed by DSC [Citation29]. DSC explores crystallinity of colloidal SLN system. DSC graph of OP showed a characteristic melting peak at 259.7 °C. In contrast to bulk tristearin, the endothermic peak of blank SLN and OPSLNs drifted 3 °C and 3.3 °C, respectively to the left. The thermogram of OPSLNs did not confirm melting peak of the crystalline OP at around 259.7 °C, which indicated the amorphous state of the drug in the formulation. The tristearin exhibited a melting endothermic peak at around 73.2 °C. However, OPSLNs drifted endothermic peak by about 3.3 °C suggesting the formation of less well-ordered lipid crystals owing to the embedding of the drug inside SLN. The decrease in the melting point of SLN over pure tristearin can be ascribed to the colloidal property of the SLN particles. The broadening and decline in the sharpness of the endothermic peak suggest an increased number of lattice defects [Citation30]. The results of DSC and XRD were also correlated in respect of the amorphous form of OP.
In vitro drug release study
The release profile of OPSLNs and OPSLNFs formulation is shown in . In vitro release investigation was performed at physiological pH 7.4 (37 ± 0.5 °C) using dialysis method. Results revealed that the OP release was higher for both OPSLNs and OPSLNFs formulation in the initial hours, i.e. 34.76% and 32.56%, respectively reflecting a burst release of the drug [Citation31]. Afterwards, formulations showed sustained release up to 144 h. After 144 h, drug release from OPSLNs and OPSLNFs was found to 99.39 ± 1.3% and 93.69 ± 1.1% that indicated sustained drug release pattern [Citation32]. The sustained release profile of OP was attributed to the diffusing property of tristearin through the lipoidal membrane. Burst release of drug occurred initially up to 34% from SLN. A probable mechanism, which may be responsible for the burst release is the drug entrapped in the outer stratum or adsorbed on the surface.
Figure 10. Drug release profile of OP loaded SLN formulations in PBS (pH 7.4) at 37 °C (±SD, n = 3).
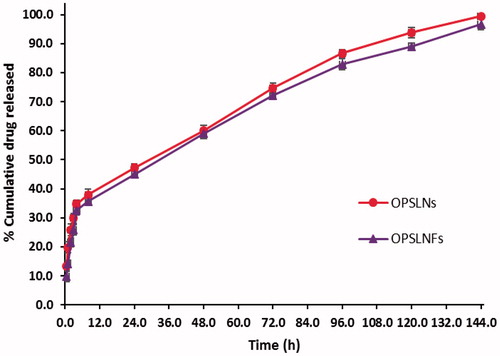
This may be credited to the aqueous solubility of OP accounting rapid dissolution of drug available in the surface of the particles. After 4 h, the drug release rate was observed slower and sustained for 144 h. The existing biphasic drug release behaviour is also supported by several research groups [Citation33]. However, grafting of FA on the SLN surface decreased drug release that could be owing to structural veracity offered by the coupling of FA, which might result into dual barrier effect for OP diffusion. In vitro release results of OPSLNs and OPSLNFs formulations were further analyzed after treating data in different kinetic models such as zero-order, first-order, Korsmeyer–Peppas, Higuchi, and Hixson–Crowell. Both formulations best-fitted in Higuchi model as compared to other models (), which is confirmed by comparing obtained values of regression coefficients for various kinetics models (). Higuchi kinetics model shows high linearity and depict that the drug release pattern obeys Fick’s Law and follows simple diffusion.
Table 4. Results for kinetic models of OP-loaded coupled and uncoupled SLNs by fitting in vitro release study.
Anticancer potential
The cell viability results are presented in . Cytotoxicity potential of OPSLNs and OPSLNFs was determined against HT-29 cell line. Results depicted that cell viability in all the three formulations, i.e. OPSLNs, OPSLNFs, and free OP solution is concentration dependent. OP-free SLNFs (Blank) did not confirm any significant cytotoxicity to HT-29 cells. All the formulations, i.e. OPSLNFs, OPSLNs, and free OP solution showed less IC50 value (i.e. IC50<10 µg/ml). The cytotoxicity studies showed that OPSLNFs had significant higher cytotoxicity than OPSLNs and free OP solution. However, OP solution has exerted the potent cytotoxic effect, but the IC50 value of free OP solution was found to be higher than OPSLNFs and OPSLNs. The IC50 value of OPSLNs was found to be higher than OPSLNFs but was lower than free OP. OP-loaded folate coupled SLNs exhibited significantly higher cytotoxicity as compared to plain drug solution. This phenomenon may be attributed to the conjugation of SLN with FA, which enhanced cellular uptake, via receptor mediated internalization by the cells [Citation34]. The folate conjugation on SLNs periphery might have contributed to lower IC50 value. OPSLNFs showed higher uptake than OPSLNs owing to receptor mediated endocytosis by the HT-29 cell lines (). This might explain the higher toxicity of OPSLNFs than OPSLNs and free OP. revealed that there was a significant change in % control growth in a number of cells treated with of OP solution, OPSLNs, and OPSLNFs.
Stability study
The results showed that there was a slight increase in the PS during the 6 months of storage at 4 ± 1 °C and 25 ± 1 °C, size of OPSLNs increased from 146 ± 2.14 nm to 154 ± 2.68 nm and 159 ± 3.01 nm, respectively, while size of OPSLNFs increased from 158 ± 2.14 nm to 167 ± 2.96 nm and 170 ± 3.42 nm, respectively (). This shows good physical stability against aggregation and/or coalescence. In contrast, the %EE of the OPSLNs was found to be from 49.2 ± 0.38% to 48.4 ± 0.57% and 47.6 ± 0.89%, respectively, and for OPSLNFs, it was found to be 43.5 ± 0.59% to 42.7 ± 0.74% and 42.1 ± 1.09%, respectively, at 4 ± 1 °C and 25 ± 1 °C. Decrease in the EE might be owing to transitions of the lipid from metastable to stable form that happen gradually during storage due to small size of the particle and in the presence of emulsifier, which may result in drug expulsion through SLNs.
Conclusions
The developed OPSLNs and OPSLNFs formulations revealed good EE, 49.2 ± 0.38% and 43.5 ± 0.59%, respectively and small PS, 146.2 ± 4.4 nm, and 158.8 ± 5.6 nm, respectively. XRD pattern and DSC results confirmed that OP was uniformly distributed in amorphous form within SLNs. In vitro drug release study of OPSLNs and OPSLNFs formulation revealed sustained drug release pattern for up to 6 d. Anticancer activity on HT-29 cell line indicated the highest potency of OPSLNFs as compared to OPSLNs and OP solution. The present work illustrated the higher sensitivity of HT-29 cells to the drug entrapped in OPSLNFs as compared to OPSLNs and OP solution. Hence, this novel strategy might be a promising approach for the management of CRC.
Disclosure statement
Authors declare no conflict of interest.
Additional information
Funding
References
- Schwarz C, Mehnert W, Lucks JS, et al. Solid lipid nanoparticles (SLN) for controlled drug delivery. I. Production, characterization and sterilization. J Control Release. 1994;30:83–96.
- Taylor MS, Daniels AU, Andriano KP, et al. Six bioabsorbable polymers: in vitro acute toxicity of accumulated degradation products. J Appl Biomater. 1994;5:151–157.
- Jenning V, Gohla SH. Encapsulation of retinoids in solid lipid nanoparticles (SLN). J Microencapsul. 2001;18:149–158.
- Pooja D, Kulhari H, Kuncha M, et al. Improving efficacy, oral bioavailability, and delivery of paclitaxel using protein-grafted solid lipid nanoparticles. Mol Pharm. 2016;13:3903–3912.
- Wang F, Li L, Liu B, et al. Hyaluronic acid decorated pluronic P85 solid lipid nanoparticles as a potential carrier to overcome multidrug resistance in cervical and breast cancer. Biomed Pharmacother. 2016;86:595–604.
- Soni N, Soni N, Pandey H, et al. Augmented delivery of gemcitabine in lung cancer cells exploring mannose anchored solid lipid nanoparticles. J Colloid Interface Sci. 2016;481:107–116.
- Yuan F, Dellian M, Fukumura D, et al. Vascular permeability in a human tumor xenograft: molecular size dependence and cutoff size. Cancer Res. 1995;55:3752–3756.
- Yang SJ, Lin FH, Tsai KC, et al. Folic acid-conjugated chitosan nanoparticles enhanced protoporphyrin IX accumulation in colorectal cancer cells. Bioconjug Chem. 2010;21:679–689.
- Ji J, Zuo P, Wang YL. Enhanced antiproliferative effect of carboplatin in cervical cancer cells utilizing folate-grafted polymeric nanoparticles. Nanoscale Res Lett. 2015;10:453.
- Kennedy MD, Jallad KN, Lu J, et al. Evaluation of folate conjugate uptake and transport by the choroid plexus of mice. Pharm Res. 2003;20:714–719.
- Graham J, Muhsin M, Kirkpatrick P. Oxaliplatin. Nat Rev Drug Discov. 2004;3:11–12.
- Culy CR, Clemett D, Wiseman LR. Oxaliplatin. A review of its pharmacological properties and clinical efficacy in metastatic colorectal cancer and its potential in other malignancies. Drugs. 2000;60:895–924.
- Jain A, Jain SK, Ganesh N, et al. Design and development of ligand-appended polysaccharidic nanoparticles for the delivery of oxaliplatin in colorectal cancer. Nanomedicine. 2010;6:179–190.
- Cho HJ, Park JW, Yoon IS, et al. Surface-modified solid lipid nanoparticles for oral delivery of docetaxel: enhanced intestinal absorption and lymphatic uptake. Int J Nanomedicine. 2014;9:495–504.
- Raj R, Raj PM, Ram A. Preparation and characterization of solid lipid nanoparticles loaded with cytarabine via a micellar composition for leukemia. RSC Adv. 2016;6:53578–53586.
- Hu FQ, Yuan H, Zhang HH, et al. Preparation of solid lipid nanoparticles with clobetasol propionate by a novel solvent diffusion method in aqueous system and physicochemical characterization. Int J Pharm. 2002;239:121–128.
- Gupta Y, Jain A, Jain SK. Transferrin-conjugated solid lipid nanoparticles for enhanced delivery of quinine dihydrochloride to the brain. J Pharm Pharmacol. 2007;59:935–940.
- Fry DW, White JC, Goldman ID. Rapid separation of low molecular weight solutes from liposomes without dilution. Anal Biochem. 1978;90:809–815.
- Cavaco MC, Pereira C, Kreutzer B, et al. Evading P-glycoprotein mediated-efflux chemoresistance using Solid Lipid Nanoparticles. Eur J Pharm Biopharm. 2017;110:76–84.
- Mehnert W, Mäder K. Solid lipid nanoparticles: production, characterization and applications. Adv Drug Del Rev. 2001;47:165–196.
- Luo Y, Teng Z, Li Y, et al. Solid lipid nanoparticles for oral drug delivery: chitosan coating improves stability, controlled delivery, mucoadhesion and cellular uptake. Carbohydr Polym. 2015;122:221–229.
- Taveira SF, De Santana DC, Araujo LM, et al. Effect of iontophoresis on topical delivery of doxorubicin-loaded solid lipid nanoparticles. J Biomed Nanotechnol. 2014;10:1382–1390.
- Martins SM, Sarmento B, Nunes C, et al. Brain targeting effect of camptothecin-loaded solid lipid nanoparticles in rat after intravenous administration. Eur J Pharm Biopharm. 2013;85:488–502.
- Alex MRA, Chacko AJ, Jose S, et al. Lopinavir loaded solid lipid nanoparticles (SLN) for intestinal lymphatic targeting. Eur J Pharm Sci. 2011;42:11–18.
- Chalikwar SS, Belgamwar VS, Talele VR, et al. Formulation and evaluation of Nimodipine-loaded solid lipid nanoparticles delivered via lymphatic transport system. Colloids Surf B Biointerfaces. 2012;97:109–116.
- Müller RH, Mäder K, Gohla S. Solid lipid nanoparticles (SLN) for controlled drug delivery – a review of the state of the art. Eur J Pharm Biopharm. 2000;50:161–177.
- Morel S, Terreno E, Ugazio E, et al. NMR relaxometric investigations of solid lipid nanoparticles (SLN) containing gadolinium(III) complexes. Eur J Pharm Biopharm. 1998;45:157–163.
- Mukherjee S, Ray S, Thakur RS. Solid lipid nanoparticles: a modern formulation approach in drug delivery system. Indian J Pharm Sci. 2009;71:349–358.
- Bunjes H, Steiniger F, Richter W. Visualizing the structure of triglyceride nanoparticles in different crystal modifications. Langmuir. 2007;23:4005–4011.
- Bunjes H, Westesen K, Koch MHJ. Crystallization tendency and polymorphic transitions in triglyceride nanoparticles. Int J Pharm. 1996;129:159–173.
- Bishnoi M, Jain A, Hurkat P, et al. Aceclofenac-loaded chondroitin sulfate conjugated SLNs for effective management of osteoarthritis. J Drug Target. 2014;22:805–812.
- Kharya P, Jain A, Gulbake A, et al. Phenylalanine-coupled solid lipid nanoparticles for brain tumor targeting. J Nanopart Res. 2013;15:2022.
- Jain A, Kesharwani P, Garg NK, et al. Galactose engineered solid lipid nanoparticles for targeted delivery of doxorubicin. Colloids Surf B Biointerfaces. 2015;134:47–58.
- Kim H, Jo A, Baek S, et al. Synergistically enhanced selective intracellular uptake of anticancer drug carrier comprising folic acid-conjugated hydrogels containing magnetite nanoparticles. Sci Rep. 2017;7:41090.