Abstract
Overcoming blood–brain barrier (BBB) and targeting tumor cells are two key steps for glioma chemotherapy. By taking advantage of the specific expression of Na+-coupled carnitine transporter 2 (OCTN2) on both brain capillary endothelial cells and glioma cells, l-carnitine conjugated poly(lactic-co-glycolic acid) nanoparticles (LC-PLGA NPs) were prepared to enable enhanced BBB permeation and glioma-cell targeting. Conjugation of l-carnitine significantly enhanced the uptake of PLGA nanoparticles in the BBB endothelial cell line hCMEC/D3 and the glioma cell line T98G. The uptake was dependent on Na+ and inhibited by the excessive free l-carnitine, suggesting involvement of OCTN2 in the process. In vivo mouse studies showed that LC-PLGA NPs resulted in high accumulation in the brain as indicated by the biodistribution and imaging assays. Furthermore, compared to Taxol and paclitaxel-loaded unmodified PLGA NPs, the drug-loaded LC-PLGA NPs showed improved anti-glioma efficacy in both 2D-cell and 3D-spheroid models. The PEG spacer length of the ligand attached to the nanoparticles was optimized, and the formulation with PEG1000 (LC-1000-PLGA NPs) showed the maximum targeting efficiency. We conclude that l-carnitine-mediated cellular recognition and internalization via OCTN2 significantly facilitate the transcytosis of nanoparticles across BBB and the uptake of nanoparticles in glioma cells, resulting in improved anti-glioma efficacy.
Graphical Abstract
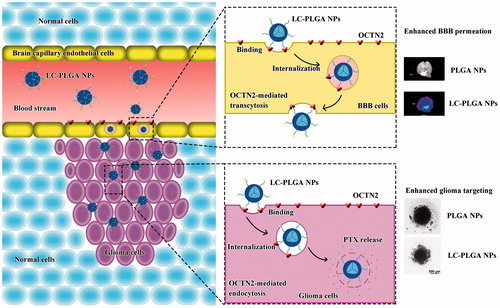
Introduction
Glioma is the most malignant form of brain tumor with high morbidity and mortality [Citation1,Citation2]. Due to its infiltrative, invasive and proliferative properties, glioma usually results in death of the patient even with aggressive treatment (surgery or radiation) [Citation3]. Chemotherapy is important in the adjunctive treatment for glioma. However, the efficacy of chemotherapy is highly limited by blood-brain barrier (BBB), an innate bio-barrier that separates the brain from systemic blood circulation and prevents the entry of more than 98% of small-molecule drugs into the brain [Citation4–6]. Furthermore, the poor biodistribution of therapeutics in glioma is a notable problem in anti-glioma therapy [Citation7,Citation8]. Thus, overcoming BBB and increasing glioma drug distribution are extremely essential for the drug delivery system in anti-glioma chemotherapy.
In recent years, the applications of nanotechnology such as nanoparticles, micelles, and liposomes, have made great contributions to increase the efficiency of drug delivery for cancer therapy [Citation9,Citation10]. However, the conventional nano-drug delivery system (nano-DDS) does not provide satisfactory permeation across the BBB for anti-glioma therapy [Citation7]. To improve the efficiency of drug delivery to glioma, various modifications of nano-DDS have been developed. Among them, a key strategy is to functionalize the surface of the nanoparticles with specific ligands, such as peptides and antibodies, that can specifically bind to receptors/transporters expressed on both blood-brain barrier and glioma cells [Citation8]. The current increased understanding and knowledge of the biology and biochemistry of BBB and tumor cells has also identified specific receptors/transporters highly expressed on BBB endothelial cells and on glioma cells, which further enabled to improve efficacy of nano-DDS against glioma [Citation4,Citation11].
Plasma membrane transporters have attracted increasing interest in recent years as they can be used as promising targets for nano-DDS. The various transporters expressed on BBB have been considered to play a critical role in supplying the brain with nutrients such as sugars, amino acids, peptides, and vitamins [Citation11–13]. These nutrient transporters also have great potential for use as portals for brain drug delivery. As a matter of fact, several of such transporters, including facilitative glucose transporter 1 (GLUT1, SLC2A1) [Citation14,Citation15], choline transporters (the members of SLC44 gene family) [Citation16], sodium-dependent vitamin C transporter 2 (SVCT2) [Citation17], and large neutral amino acid transporter (LAT1, SLC7A5) [Citation18] have been examined for drug delivery into cells in the form of nanoparticles by chemically modifying their surface with ligands that are recognized by the respective transporters. This approach has advantages to improve permeation across BBB by targeting the transporters that are expressed at high levels in the endothelial cells that form this barrier. The same logic could be used for drug delivery into tumor cells because selective nutrient transporters are upregulated in these cells to support their rapid growth. This is also true with glioma cells. It has been reported that the nutrient transporters GLUT1, SVCT2, LAT1, and choline transporters show significant promise for the delivery of drugs in the form of nanoparticles for glioma therapy [Citation14–18]. Novel organic cation/carnitine transporter 2 (OCTN2, SLC22A5) is a transporter for l-carnitine, a small molecule absolutely obligatory for β-oxidation of long-chain fatty acids in mitochondria [Citation19,Citation20]. This transporter is highly expressed in brain capillary endothelial cells that constitute the BBB [Citation21–24]. Even though brain cells do not utilize β-oxidation of fatty acids to any significant extent to generate energy, acetyl-l-carnitine plays a critical role in brain function and energy status [Citation24,Citation25]. OCTN2 in BBB facilitates the transfer of this important metabolite from systemic circulation to brain across the BBB. In the present study, we have found that OCTN2 is also highly expressed in glioblastoma multiforme T98G cells. As such, OCTN2 could be exploited as a portal for drug delivery using nano-DDS to facilitate permeation across the BBB and also to selectively target glioma cells for drug exposure.
In this study, with paclitaxel as a model drug and poly(lactic-co-glycolic acid) (PLGA) nanoparticles as a carrier, l-carnitine-conjugated nanoparticles (LC-PLGA NPs) were developed to target OCTN2 expressed on BBB and glioma cells to improve the anti-glioma efficacy of chemotherapeutics. In addition, as the length of polyethylene glycol (PEG) spacer of ligand/antibody attached to the surface of nanoparticles is known to affect the binding of ligand/antibody to the target and thus the targeting efficiency [Citation26–28] we also evaluated the PEG spacer length for optimal recognition by the transporter (Scheme 1). We employed the human brain capillary endothelial cell line hCEMC/D3 and the human glioblastoma multiforme cell line T98G as models for BBB and glioma. Biodistribution analysis was performed to determine the OCTN2-mediated BBB penetration and brain accumulation, and in vitro tumor growth with T98G cells as 3 D-cell spheroids was used to evaluate the anti-glioma efficacy.
Materials and methods
This section is displayed in the supplemental material.
Results
Synthesis and characterization of LC-PnS (n = 10, 25, 40)
l-Carnitine-modified polyoxyethylene stearate (LC-PnS) was synthesized using the synthetic scheme shown in Figure S1. The chemical structures of intermediates and final products were confirmed by 1H NMR, and the spectra were illustrated in Figure S2. As for l-carnitine, the carboxylic acid group and the trimethyl amino group were essential to be recognized by OCTN2 [Citation29]. Therefore, the carboxylic acid group was protected with benzyl group, and the hydroxyl group was conjugated with polyoxyethylene stearate with succinyl moiety. After conjugation, the characteristic peaks at δ 7.4 ppm for phenyl ring and at δ 3.3 ppm for trimethyl amino group were detectable (Figure S2(C)). The benzyl group was then removed by reduction, and the peak at δ 7.4 ppm disappeared.
Preparation and characterization of l-carnitine-conjugated nanoparticles (LC-PLGA NPs)
LC-PLGA NPs with different PEG spacer lengths (LC-0-PLGA NPs, LC-500-PLGA NPs, LC-1000-PLGA NPs, and LC-2000-PLGA NPs) and the unmodified nanoparticles (PLGA NPs) were prepared by the solvent extraction/evaporation method [Citation30]. Paclitaxel as a model drug and coumarin 6/DIR as a fluorescence probe were used to monitor the drug delivery efficiency of nanoparticles. The physicochemical characteristics of nanoparticles were summarized in . The size of the nanoparticles ranged from 180 to 210 nm with a narrow size distribution (PDI <0.2; ). After conjugation with l-carnitine, the size of nanoparticles increased slightly. The transmission electron microscope (TEM) images of LC-PLGA NPs shown in demonstrated a spheroid shape and narrow size distribution. However, the particle size of nanoparticles by TEM was smaller than that by dynamic light scattering (DLS). It might be due to the fact that the hydro-layer of nanoparticles was extensively stretched in aqueous solution (in DLS), but collapsed and shrunken when nanoparticles were dried (in TEM). In the in vitro release test (), paclitaxel-loaded LC-PLGA NPs with different PEG spacer lengths showed a sustained release of paclitaxel, compared to Taxol. The nanoparticles were quite stable in pH 7.4 PBS at 37 °C ().
Figure 1. (A) Particle size and size distribution of PLGA NPs and L-Carnitine-conjugated nanoparticles; TEM image of LC-0-PLGA NPs (B), LC-500-PLGA NPs (C), LC-1000-PLGA NPs (D), and LC-2000-PLGA NPs (E); (F) In vitro release profiles of Taxol, paclitaxel-loaded PLG PLGA NPs, and l-Carnitine-conjugated nanoparticles; (G) Colloidal stability of paclitaxel-loaded PLGA NPs and LC-PLGA NPs in pH 7.4 PBS at 37 °C. Data are shown as mean ± SD, n = 3.
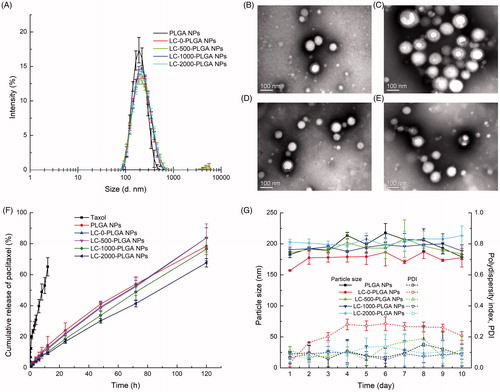
Table 1. Physicochemical characterization of LC-PLGA NPs.
Expression of OCTN2 in cell lines and brain
The expression of OCTN2 in brain capillary endothelial cells (hCEMC/D3) and the glioblastoma multiforme cells (T98G) was monitored by RT-PCR (), western blot (), and immunofluorescence (). In both RT-PCR and western blot, Caco-2 was used as a positive control. Both hCMEC/D3 and T98G cell lines showed high OCTN2 expression; the expression in T98G cells was a little higher than that in hCMEC/D3 cells. The immunofluorescence analysis in hCMEC/D3 () and T98G () cells was performed; for negative control, primary antibody was omitted. The red fluorescence signals in both cell lines confirmed the expression of OCTN2. In addition, four mice were sacrificed and the brains were taken out for the analysis of OCTN2 expression. The high OCTN2 expression in all four brain samples was demonstrated by RT-PCR (Figure S3(A)) and western blot (Figure S3(B)).
Cellular uptake assay
The uptake features of LC-PLGA NPs with different PEG spacer lengths and unmodified PLGA NPs were investigated in hCMEC/D3 and T98G cells. Coumarin 6 was used as a fluorescence probe to track the uptake process. As shown in , after l-carnitine conjugation, the cellular internalization of nanoparticles was significantly higher than that of the unmodified PLGA NPs in hCMEC/D3 cells. As for the formulations with different spacers, compared to the formulation without PEG spacer (LC-0-PLGA NPs), LC-1000-PLGA NPs increased the uptake of coumarin 6, but LC-500-PLGA NPs and LC-2000-PLGA NPs had no significant effect on the uptake. This phenomenon was confirmed by fluorescence microscopy (). These data indicated that there is an optimal length of PEG spacer for l-carnitine-conjugated nanoparticles for maximal uptake.
Figure 3. Characteristics of nanoparticles uptake in hCMEC/D3 cells. (A) Fluorescence images (20×) of hCMEC/D3 cells incubated with coumarin 6-loaded LC-PLGA NPs at 37 °C for 1 h. (B) Uptake assay for LC-PLGA NPs in NaCl buffer (control), NMDG chloride buffer (Na+-free), and NaCl buffer with 10 mM l-Carnitine. Data are shown as mean ± SD, n = 3. *p < .05, **p < .01 versus PLGA NPs group; α, p < .05, β, p < .01 versus control group. (C) Impact of inhibitors of various endocytosis pathways on the uptake of PLGA NPs and LC-1000-PLGA NPs in hCMEC/D3 cells. Data are shown as mean ± SD, n = 3. *p < .05, **p < .01 versus control group without inhibitor treatment.
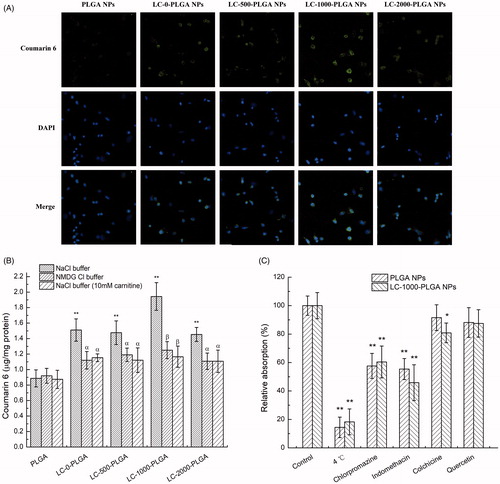
The increased uptake of l-carnitine-conjugated nanoparticles might be due to their interaction with OCTN2 expressed on cell membrane. To confirm the role of OCTN2 in the uptake of l-carnitine-conjugated nanoparticles, the influence of Na+ on the uptake of nanoparticles was examined in hCMEC/D3 cells (). The uptake of PLGA NPs was independent of Na+. In contrast, after conjugation with l-carnitine, the uptake of nanoparticles was dependent on Na+, and the uptake was significantly decreased when Na+ in uptake buffer was removed. In addition, excessive free l-carnitine in uptake buffer resulted in a significant decrease in the uptake of l-carnitine-conjugated nanoparticles in the presence of Na+ (). These data suggested that the l-carnitine conjugated on the surface of nanoparticles facilitated the uptake in hCMEC/D3 cells, and this process was stimulated by Na+ and inhibited by free l-carnitine. These findings suggest that the Na+-dependent carnitine transporter OCTN2 was involved in the uptake of l-carnitine-conjugated nanoparticles in hCMEC/D3 cells.
Furthermore, the endocytosis mechanisms were investigated by using various endocytosis inhibitors (). Prior that, the uptake assay was first conducted at 4 °C, and the uptake of both PLGA NPs and LC-1000-PLGA NPs was significantly decreased, indicating that endocytosis was involved in the uptake process. Both indomethacin (an inhibitor of caveolin-dependent endocytosis) and chlorpromazine (an inhibitor of clathrin-dependent endocytosis) displayed remarkably inhibitory effect on the uptake, but colchicine (an inhibitor of macropinocytosis) and quercetin (an inhibitor of clathrin- and caveolin-independent endocytosis) showed lower or no effect on the uptake process.
The uptake features of PLGA NPs, LC-0-PLGA NPs, LC-500-PLGA NPs, LC-1000-PLGA NPs, and LC-2000-PLGA NPs in T98G cells were similar to those in hCMEC/D3 cells (). These features demonstrated an OCTN2-mediated endocytosis employed by LC-PLGA NPs not only in BBB endothelial cells but also in glioma cells.
Figure 4. Characteristics of nanoparticles uptake in T98G cells. (A) Fluorescence images (20×) of T98G cells incubated with coumarin 6-loaded LC-PLGA NPs at 37 °C for 1 h. B, Uptake assay for LC-PLGA NPs in NaCl buffer (control), NMDG chloride buffer (Na+-free), and NaCl buffer with 10 mM l-Carnitine. Data are shown as mean ± SD, n = 3. *p < .05, **p < .01 versus PLGA NPs group; α, p < .05, β, p < .01 versus control group. (C) Impact of inhibitors of various endocytosis pathways on the uptake of PLGA NPs and LC-1000-PLGA NPs in T98G cells. Data are shown as mean ± SD, n = 3. *p < .05, **p < .01 versus control group without inhibitor treatment.
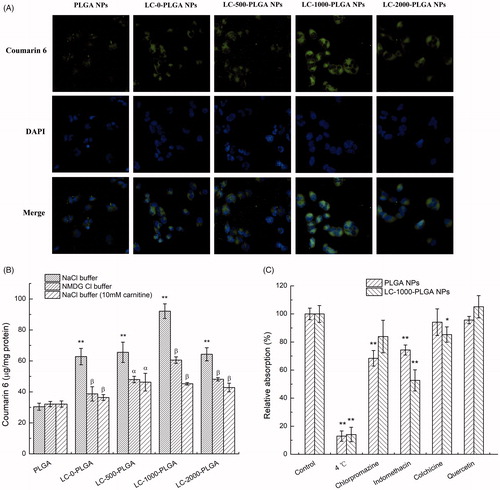
In vitro cytotoxicity
The in vitro anti-glioma effect of Taxol, paclitaxel-loaded PLGA NPs, LC-0-PLGA NPs, LC-500-PLGA NPs, LC-1000-PLGA NPs, and LC-2000-PLGA NPs was studied in glioblastoma multiforme cells (T98G) by MTT assay. As shown in , after conjugation with l-carnitine, the cytotoxicity with nanoparticles increased compared to PLGA NPs and Taxol, possibly due to the improved cellular uptake. Of them, LC-1000-PLGA NPs exhibited significantly higher cytotoxicity than other formulations, suggesting an optimal PEG spacer length for maximal cellular uptake of the nanoparticles.
OCTN2-mediated brain accumulation
The brain distribution patterns of Taxol and paclitaxel-loaded nanoparticles are expressed as paclitaxel (ng) in brain (mg) of mice after 2, 6, 12 h as shown in . Free Taxol distributed in brain is observed to be very little. LC-0-PLGA NPs, LC-500-PLGA NPs and LC-1000-PLGA NPs significantly enhanced the brain accumulation of paclitaxel compared to Taxol and paclitaxel-loaded PLGA NPs at 2 h, but not LC-2000-PLGA NPs. Of them, LC-1000-PLGA NPs improved the brain accumulation up to ∼13-fold compared to Taxol and ∼11-fold compared to PLGA NPs. At 6 h, LC-1000-PLGA NPs enhanced the brain accumulation of paclitaxel ∼14-fold compared to Taxol and ∼2-fold compared to PLGA NPs.
Figure 6. Brain distribution of paclitaxel after intravenous injection with Taxol, paclitaxel-loaded PLGA NPs and LC-PLGA NPs. Data are shown as mean ± SD, n = 3. *p < .05, **p < .01 versus Taxol; α, p < .05, β, p < .01 versus PLGA NPs.
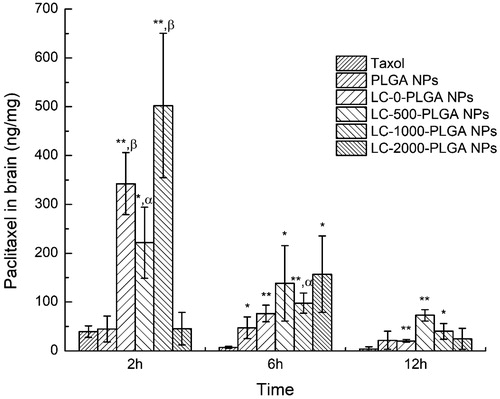
The brain accumulation of DIR-loaded PLGA NPs, LC-0-PLGA NPs and LC-1000-PLGA NPs were then investigated by FX Pro in vivo image system (). The signal of PLGA NPs in brain was undetectable at 2, 4, 6 and 12 h, indicating low permeability across BBB for bare nanoparticles. After conjugation with l-carnitine, LC-0-PLGA NPs and LC-1000-PLGA NPs accelerated DIR accumulation in the brain at all time points, and the signals were stronger for these conjugated NPs than for PLGA NPs. LC-1000-PLGA NPs increased the biodistribution of DIR in brain much further compared to LC-0-PLGA NPs and the signals lasted for 12 h, suggesting that the optimal PEG spacer could maximize the targeting efficiency of LC-PLGA NPs.
In vitro anti-glioma efficacy
Cancer cell 3 D spheroids are increasingly recognized as a desirable experimental model system for accurate evaluation of nano-DDS. T98G cell spheroids were used to evaluate the in vitro anti-glioma efficacy of LC-PLGA NPs. Taxol, paclitaxel-loaded PLGA NPs, LC-0-PLGA NPs, and LC-1000-PLGA NPs with two concentrations (1 and 10 µg/mL) were tested, and morphology and size of T98G spheroids with treatment were recorded. After treatment with paclitaxel-loaded formulation, the spheroids began to decomplex from the periphery (). The spheroids in control group without paclitaxel treatment grew tightly with time. As shown in , the growth of the spheroids decreased significantly with LC-PLGA formulations compared to the control group. Among them, LC-PLGA NPs exhibited enhanced toxicity, indicating OCTN2-mediated cellular uptake enhanced the anti-glioma efficacy. LC-1000-PLGA NPs were the most effective in in vitro tumor inhibition, demonstrating that the optimal PEG spacer resulted in maximal OCTN2-targeting efficiency and the most potent anti-glioma effect.
Figure 8. Response of T98G Spheroids to treatment with Taxol, paclitaxel-loaded PLGA NPs, LC-0-PLGA NPs and LC-1000-PLGA NPs. (A) The morphology change of T98G spheroids in a duration of 10 day-treatment with 10 μg/mL of Taxol, paclitaxel-loaded PLGA NPs and LC-PLGA NPs; (B) Compared to control group, the size change of T98G spheroids with treatment of 1 and 10 μg/mL of Taxol, paclitaxel-loaded PLGA NPs and LC-PLGA NPs. Data are shown as mean ± SD, n = 3.
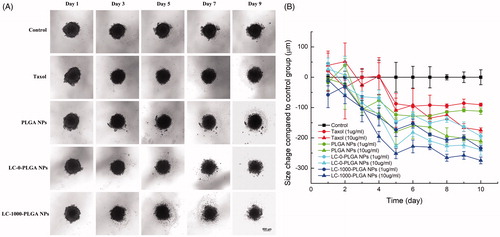
Discussion
Glioma is a primary malignant tumor in the central nervous system. The treatment of glioma is one of the most challenging problems in the clinic because there is no available curative treatment so far. Surgery is a basic treatment, but it is impossible to remove glioma due to the aggressive profile of the tumor. The peripheral infiltrating part remaining after surgery should be managed by adjunctive treatment, mainly chemotherapy. However, the current anti-cancer drugs remain mostly ineffective for glioma treatment owing to the limitations with regard to drug permeability across BBB and the low distribution of the drugs in tumor cells. Recently, nano-DDS has been examined to overcome the hurdle of the blood-brain barrier [Citation31], to target glioma cells [Citation32], and to overcome the BBB hurdle and also to target glioma cells at the same time [Citation33,Citation34].
Nano-DDS capable of overcoming BBB and targeting glioma cells have great potential for anti-glioma therapy. The brain is the most energy-consuming organ, consuming 20% of the energy produced by the body. Glioma with the feature of malignant growth also need a lot of energy. Thus, transporters for various nutrients are expressed at high levels on BBB and glioma cells; these transporters represent ideal targets for nano-DDS targeting both BBB and glioma cells [Citation11,Citation35]. GLUT1 is a transporter mainly responsible for the transport of glucose to provide energy for brain, and it is highly expressed on BBB and glioma cells. GLUT1 has been successfully used as a target to trigger the transcytosis across BBB and target glioma cells [Citation14,Citation15]. Choline transporters, SVCT2, and LAT1 have also been exploited for anti-glioma therapy using a similar strategy [Citation16–18]. It has been well documented that OCTN2, a Na+-coupled transporter for l-carnitine, is also expressed in brain capillary endothelial cells that constitute BBB [Citation21–24]. l-Carnitine-conjugated prodrugs have been studied to overcome BBB and enhance drug accumulation in brain using OCTN2-mediated pathway [Citation36]. There is no information available at present in published literature regarding the expression of OCTN2 in glioma. In the present study, we examined the expression of OCTN2 both in brain capillary endothelial cells (hCEMC/D3) and the glioblastoma multiforme cells (T98G) (), and found that both cell lines expressed OCTN2, suggesting that OCTN2 also can be used as an ideal portal to target nano-DDS for BBB and glioma cells for effective anti-glioma therapy.
LC-PLGA NPs with different PEG spacer lengths were prepared and characterized. The brain capillary endothelial cells (hCEMC/D3) and the glioblastoma multiforme cells (T98G) were used to evaluate the dual-targeting effect of l-carnitine-conjugated nanoparticles. By using coumarin 6 as a fluorescence probe, the cellular uptake of nanoparticles was investigated in both cells. Significantly higher cellular uptake of LC-PLGA NPs was achieved in both hCMEC/D3 and T98G cells than the unmodified PLGA NPs. The cellular uptake of LC-PLGA NPs was Na+-dependent and sensitive to inhibition by excess free l-carnitine; this was not the case with unconjugated PLGA NPs. In addition, LC-PLGA NPs mainly employed caveolin-dependent, clathrin-dependent endocytosis, and macropinocytosis. Of them, caveolin-dependent endocytosis was preferred by LC-PLGA NPs. The difference of endocytosis mechanism between PLGA NPs and LC-PLGA NPs might be due to the conjugation of l-carnitine [Citation30,Citation37]. These data strongly implicate that OCTN2-mediated endocytosis are involved in the cellular uptake of LC-PLGA NPs.
It has been suggested that the interaction of ligands with targets on cell surface mostly occurs through multivalent binding [Citation26,Citation30,Citation38,Citation39]. Therefore, it is reasonable that the PEG spacer length of ligand attached to nanoparticle surface would affect the binding of ligand to the target, and thus influence the target efficiency. Here, by modulating the length of PEG (PEG = 0, 500, 1000, 2000 Da) for the conjugation of l-carnitine, a series of formulations (LC-0-PLGA NPs, LC-500-PLGA NPs, LC-1000-PLGA NPs, and LC-2000-PLGA NPs) were used to investigate the role of PEG spacer in the interaction of l-carnitine to OCTN2 and the overall targeting process. In both hCMEC/D3 and T98G cells, the cellular uptake of LC-PLGA NPs varied with the length of PEG spacer. The uptake of LC-PLGA NPs increased with the length of PEG spacer increasing from 0 to 1000, but decreased with further increase in the spacer length. Within the range of the spacer length 0 to 1000, the PEG spacer could increase the flexibility and mobility, indicating a better targeting and binding efficiency for l-carnitine to OCTN2 (). However, with the further increase, the PEG spacer becomes too long and the relatively small molecule l-carnitine could be masked by PEG, thus interfering with the recognition of the l-carnitine -conjugated NPs by OCTN2 (). This phenomenon of optimal PEG spacer length was also evident in the in vivo biodistribution study ( and ) and in vitro anti-glioma assay ( and ).
Figure 9. Role of PEG spacer in the targeting process. With molecular weight increasing from 0 (A) to 1000 (C), the PEG spacer could increase the flexibility and mobility, indicating a better targeting and binding efficiency for l-Carnitine to OCTN2; however, when increased further to 2000 (D), the PEG spacer was too long and small molecule l-Carnitine might be embedded into the PEG, hindering the binding efficiency of l-Carnitine to OCTN2.

Using paclitaxel as a model drug, biodistribution in mice was used to monitor the BBB permeation efficiency of the formulation in vivo. The different formulation designs markedly impacted on the brain concentrations of paclitaxel. Taxol, paclitaxel solution for injection, exhibited the lowest brain distribution, probably due to rapid clearance. PLGA NPs did not enhance the paclitaxel distribution to brain because the bare nanoparticles were not permeable across BBB. After conjugation with l-carnitine, paclitaxel accumulation in brain was significantly increased, indicating that OCTN2 was an effective target for nano-DDS for enhanced BBB permeation. The enhanced brain accumulation of LC-PLGA NPs was attributed to the specific binding of conjugated l-carnitine to OCTN2 expressed on BBB and the triggered transcytosis thereafter. Of them, LC-1000-PLGA NPs achieved the highest paclitaxel accumulation in brain due to the optimal PEG spacer. By using DIR as a fluorescence probe, FX Pro in vivo image system gave a visualized insight into the brain distribution of PLGA NPs, LC-0-PLGA NPs and LC-1000-PLGA NPs. The conjugation of l-carnitine increased the brain accumulation of nanoparticles, and LC-1000-PLGA NPs with optimal PEG spacer showed the highest brain distribution. These results indicated that l-carnitine-conjugated, OCTN2-targeting nanoparticles were an effective formulation to enhance BBB permeability for chemotherapeutics.
The anti-glioma efficacy of LC-PLGA NPs was evaluated in 2 D and 3 D tumor growth models using the glioma cell line T98G. In MTT assay, LC-PLGA NPs showed increased toxicity to the glioblastoma multiforme cells compared to Taxol and paclitaxel-loaded PLGA NPs, and LC-1000-PLGA NPs showed the highest toxicity due to the optimal spacer resulting in the best targeting efficiency. The increased cytotoxicity of LC-PLGA NPs was due to their enhanced uptake based on OCTN2-mediated absorption. 3 D-cell spheroids could mimic the original tumor environment, providing a good solid tumor model to evaluate drug delivery carriers [Citation40,Citation41]. A strikingly increased anti-glioma efficacy was observed with LC-PLGA NPs compared to Taxol and paclitaxel-loaded PLGA NPs, and LC-1000-PLGA NPs showed the best anti-glioma efficacy.
The rational design combining BBB and glioma cell dual-targeting was an effective strategy for improving anti-glioma therapy. By targeting OCTN2, LC-PLGA NPs proved to have a marked enhancement in BBB permeation and prominent increase in glioma targeting, demonstrating that the strategy has great potential for use in anti-glioma therapy. The pitfall in the approach however, is the exposure of various other organs to the drugs encapsulated in l-carnitine-conjugated PLGA NPs even though the results were expected based on the expression of OCTN2 in other tissues as well. Therefore, additional studies are needed to find ways to prevent the off-target exposure of the drugs. Nonetheless, the studies presented here provide strong support to the idea that plasma membrane transporters represent ideal portals for targeting nanoparticles for enhanced cellular uptake with appropriate modification of the surface of the nanoparticles with suitable ligands. The results presented here validate this logic by successfully overcoming the BBB hurdle with l-carnitine-conjugated nanoparticles to selectively target the plasma membrane transporter OCTN2.
Conclusion
OCTN2, a Na+-coupled l-carnitine transporter, is expressed on both brain capillary endothelial cells and glioma cells. Using OCTN2 as a target, the present study developed and optimized l-carnitine-conjugated nanoparticles (LC-PLGA NPs) as a delivery system to enhance BBB permeation and increase glioma targeting for anti-glioma therapy. Compared to unmodified nanoparticles, the optimized LC-1000-PLGA NPs exhibited significantly higher cellular uptake in both hCMEC/D3 and T98G cells, higher accumulation in brain, and better anti-glioma efficiency in 3 D T98G spheroids. OCTN2-mediated recognition and internalization significantly facilitated the transcytosis of nanoparticles across BBB and the uptake of nanoparticles in glioma cells, resulting in improved anti-glioma efficacy. These results indicated that LC-PLGA NPs were able to significantly improve anti-glioma efficacy by targeting to OCTN2, providing a promising strategy for anti-glioma therapy.
Longfa_et_al._Supplementary_material.doc
Download MS Word (465 KB)Disclosure statement
The authors report no conflicts of interest.
Additional information
Funding
References
- Ostrom QT, Gittleman H, Farah P, et al. CBTRUS statistical report: primary brain and central nervous system tumors diagnosed in the United States in 2006–2010. Neuro Oncol. 2012;14(Suppl. 2): 1–56.
- Ostrom QT, Gittleman H, de Blank PM, et al. American brain tumor association adolescent and young adult primary brain and central nervous system tumors diagnosed in the United States in 2008-2012. Neuro Oncol. 2016;1(Suppl. 1):i1.
- Arko L, Katsyv I, Park GE, et al. Experimental approaches for the treatment of malignant gliomas. Pharmacol Ther. 2010;128:1–36.
- Agarwal S, Sane R, Oberoi R, et al. Delivery of molecularly targeted therapy to malignant glioma, a disease of the whole brain. Expert Rev Mol Med. 2011;13:e17.
- Allhenn D, Boushehri MA, Lamprecht A. Drug delivery strategies for the treatment of malignant gliomas. Int J Pharm. 2012;436:299–310.
- Pardridge WM. Blood-brain barrier drug targeting: the future of brain drug development. Mol Interv. 2003;3:90–105.
- Zhan C, Lu W. The blood-brain/tumor barriers: challenges and chances for malignant gliomas targeted drug delivery. Curr Pharm Biotechnol. 2012;13:2380–2387.
- Liu Y, Lu W. Recent advances in brain tumor-targeted nano-drug delivery systems. Expert Opin Drug Deliv. 2012;9:671–686.
- Wang Z, Guo W, Kuang X, et al. Nanopreparations for mitochondria targeting drug delivery system: current strategies and future prospective. Asian J Pharm Sci 2017 [cited May 24]. DOI:10.1016/j.ajps.2017.05.006.
- Shi J, Zhang H, Chen Z, et al. A multi-functional nanoplatform for efficacy tumor theranostic applications. Asian J Pharm Sci. 2016;12:235–249.
- Mittapalli RK, Manda VK, Adkins CE, et al. Exploiting nutrient transporters at the blood-brain barrier to improve brain distribution of small molecules. Ther Deliv. 2010;1:775–784.
- Geldenhuys WJ, Mohammad AS, Adkins CE, et al. Molecular determinants of blood-brain barrier permeation. Ther Deliv. 2015;6:961–971.
- Pardridge WM. Blood–brain barrier endogenous transporters as therapeutic targets: a new model for small molecule CNS drug discovery. Expert Opin Ther Targets. 2015;19:1059–1072.
- Shao K, Ding N, Huang S, et al. Smart nanodevice combined tumor-specific vector with cellular microenvironment-triggered property for highly effective antiglioma therapy. Acs Nano. 2014;8:1191–1203.
- Jiang X, Xin H, Ren Q, et al. Nanoparticles of 2-deoxy-D-glucose functionalized poly(ethylene glycol)-co-poly(trimethylene carbonate) for dual-targeted drug delivery in glioma treatment. Biomaterials. 2014;35:518–529.
- Li J, Guo Y, Kuang Y, et al. Choline transporter-targeting and co-delivery system for glioma therapy. Biomaterials. 2013;34:9142–9148.
- Salmaso S, Pappalardo JS, Sawant RR, et al. Targeting glioma cells in vitro with ascorbate-conjugated pharmaceutical nanocarriers. Bioconjugate Chem. 2009;20:2348–2355.
- Li L, Di X, Zhang S, et al. Large amino acid transporter 1 mediated glutamate modified docetaxel-loaded liposomes for glioma targeting. Colloids Surf B Biointerfaces. 2016;141:260–267.
- Wu X, Prasad PD, Leibach FH, et al. cDNA sequence, transport function, and genomic organization of human OCTN2, a new member of the organic cation transporter family. Biochem Biophys Res Commun. 1998;246:589–595.
- Tamai I, Ohashi R, Nezu J, et al. Molecular and functional identification of sodium ion-dependent, high affinity human carnitine transporter OCTN2. J Biol Chem. 1998;273:20378–20382.
- Kido Y, Tamai I, Ohnari A, et al. Functional relevance of carnitine transporter OCTN2 to brain distribution of l-carnitine and acetyl- l-carnitine across the blood–brain barrier. J Neurochem. 2001;79:959–969.
- Miecz D, Januszewicz E, Czeredys M, et al. Localization of organic cation/carnitine transporter (OCTN2) in cells forming the blood-brain barrier. J Neurochem. 2008;104:113–123.
- Berezowski V, Miecz D, Marszałek M, et al. Involvement of OCTN2 and B0,+ in the transport of carnitine through an in vitro model of the blood-brain barrier. J Neurochem. 2004;91:860–872.
- Inano A, Sai Y, Nikaido H, et al. Acetyl-L-carnitine permeability across the blood-brain barrier and involvement of carnitine transporter OCTN2. Biopharm Drug Dispos. 2003;24:357–365.
- Virmani A, Binienda Z. Role of carnitine esters in brain neuropathology. Mol Aspects Med. 2004;25:533–549.
- Ai P, Wang H, Liu K, et al. The relative length of dual-target conjugated on iron oxide nanoparticles plays a role in brain glioma targeting. Rsc Advances. 2017;7:19954–19959.
- Xing H, Li J, Xu W, et al. The effects of spacer length and composition on aptamer-mediated cell-specific targeting with nanoscale PEGylated liposomal doxorubicin. Chembiochem. 2016;17:1111–1117.
- Li Z, Han X, Zhai Y, et al. Critical determinant of intestinal permeability and oral bioavailability of pegylated all trans-retinoic acid prodrug-based nanomicelles: chain length of poly (ethylene glycol) corona. Colloids Surf B Biointerfaces. 2015;130:133–140.
- Tamai I. Pharmacological and pathophysiological roles of carnitine/organic cation transporters (OCTNs: SLC22A4, SLC22A5 and Slc22a21). Biopharm Drug Dispos. 2013;34:29–44.
- Kou L, Yao Q, Sun M, et al. Cotransporting ion is a trigger for cellular endocytosis of transporter-targeting nanoparticles: a case study of high-efficiency SLC22A5 (OCTN2)-mediated carnitine-conjugated nanoparticles for oral delivery of therapeutic drugs. Adv Healthcare Mater. 2017;6:1700165.
- Petri B, Bootz A, Khalansky A, et al. Chemotherapy of brain tumour using doxorubicin bound to surfactant-coated poly(butyl cyanoacrylate) nanoparticles: revisiting the role of surfactants. J Controlled Release. 2007;117:51–58.
- Hadjipanayis CG, Machaidze R, Kaluzova M, et al. EGFRvIII antibody-conjugated iron oxide nanoparticles for magnetic resonance imaging-guided convection-enhanced delivery and targeted therapy of glioblastoma. Cancer Res. 2010;70:6303–6312.
- He H, Li Y, Jia XR, et al. PEGylated Poly(amidoamine) dendrimer-based dual-targeting carrier for treating brain tumors. Biomaterials. 2011;32:478–487.
- Guo J, Gao X, Su L, et al. Aptamer-functionalized PEG-PLGA nanoparticles for enhanced anti-glioma drug delivery. Biomaterials. 2011;32:8010–8020.
- Bhutia YD, Babu E, Prasad PD, et al. The amino acid transporter SLC6A14 in cancer and its potential use in chemotherapy. Asian J Pharm Sci. 2014;9:293–303.
- Napolitano C, Scaglianti M, Scalambra E, et al. Carnitine conjugate of nipecotic acid: a new example of dual prodrug. Molecules. 2009;14:3268–3274.
- Kou L, Sun J, Zhai Y, et al. The endocytosis and intracellular fate of nanomedicines: implication for rational design. Asian J Pharm Sci. 2013;8:1–10.
- Kou L, Yao Q, Sivaprakasam S, et al. Dual targeting of L-Carnitine-conjugated nanoparticles to OCTN2 and ATB0,+ to deliver chemotherapeutic agents for colon cancer therapy. Drug Delivery. 2017;24:1338–1349.
- Zhu C, Williams TE. Modeling concurrent binding of multiple molecular species in cell adhesion. Biophys J. 2000;79:1850–1857
- Wu J, Chen Q, Liu W, et al. Recent advances in microfluidic 3D cellular scaffolds for drug assays., Trends Anal Chem. 2017;87:19–31.
- Breslin S, O’Driscoll L. Three-dimensional cell culture: the missing link in drug discovery. Drug Discov Today. 2013;18:240–249.