Abstract
Strontium ranelate (SrR) is an anti-osteoporosis drug with excellent osteogenic and angiogenic capacity. In this study, we aimed to investigate the osteogenesis and angiogenesis effects of SrR and the underlying mechanism involved. RNA-Seq was conducted to examine the effects of SrR on gene expression in ovariectomy rat bone marrow mesenchymal stem cells (OVX-rBMSCs). To validate the different expressed gene in vitro, the effects of gene interference and overexpression in osteogenic induction environment of OVX-rBMSCs and in primary osteoblasts were studied. RNA-Seq showed that ROCK1 significantly increased after SrR treatment in OVX-rBMSCs, and further validated by real-time PCR and western blotting. Overexpression of ROCK1 promoted osteogenic differentiation of OVX-rBMSCs and induced cell viability and inhibited apoptosis of primary osteoblasts, which was reversed by inhibition of ROCK1 by RNA interference or ROCK1 inhibitor (Y-27632) after SrR treatment. Furthermore, the SrR was loaded on nano-structured hydroxyapatite (nano-HAp) particulates to promote osteogenesis and angiogenesis in repairing of the femoral condyle bone defect using ovariectomy rat model. Taken together, ROCK1 is one of the targets that SrR promotes the osteogenic differentiation of OVX-rBMSCs and cell viability of primary osteoblasts, the nano-HAp particles could act as carriers for SrR to repair bone defects.
Introduction
Osteoporosis is a systemic skeletal disease characterized by bone loss and structural destruction, and clinical manifest as increases bone fragility and risk of fracture. Patients with osteoporosis seriously affect the prognosis of oral and maxillofacial tumours, fractures and implants, for their degenerative osteoblasts, increased osteoclast function and inadequate bone formation [Citation1]. Along with the severe condition of social aging, large amount of osteoporosis patients will bring big challenge to the dental implant treatment. Although the long-term successful rate, osseointegration rate and the pull out strength of implant showed no significant difference between osteoporosis and normal patients, the poorer initial stability and longer healing time raise the risk of failure [Citation2]. Therefore, the acceleration of the local bone formation rate and promotion of the early osseointegration and bone regeneration in osteoporosis patients is the key issue of the current study.
Strontium ranelate (SrR) has been reported to significantly improve bone mineral density, bone quality and reduce the risk of fractures to the spine or limb bones, and it is a long-term clinical benefit for osteoporosis patients, especially post-menopausal women with osteoporosis, and possess high safety [Citation3,Citation4]. The dual anti-osteoporosis effect of SrR, which promotes osteogenesis, inhibits osteoclast differentiation and induces osteoclast apoptosis [Citation5], is mainly involved in the RANK/RANKL/OPG system. Moreover, SrR also promotes osteoblast differentiation, calcium nodules and bone matrix mineralization through activating a series of signalling pathway, including Wnt [Citation6], ERK1/2 [Citation7], Akt [Citation8], BMP-2/Smad [Citation9], Ras/MAPK [Citation10] and Hedgehog [Citation11], etc. In addition to promoting bone formation, recent studies also suggested that strontium ions (Sr2+) released from Sr-containing bioceramics can also promote angiogenesis through increasing secretion of angiogenic factors of HUVEC cells [Citation12,Citation13], leading to cell migration, adhesion and viability [Citation14,Citation15]. However, as for SrR drug, most researches were performed on the combination of SrCl2 and sodium ranelate (NaR), a few researches focus on the effect and mechanism of the intact molecular of SrR on osteogenesis and angiogenesis [Citation16], which has not been fully understood.
Hydroxyapatite (HAp) is the main component of mineralized tissue in human body, has favourable biocompatibility and osteogenic activity, and is widely used in the field of bone regeneration and bone tissue engineering. In recent years, more and more attention has been focused on the HAp with nanostructures surfaces because it can increase specific surface area and surface energy of the material and the structure of pore space itself can promote cell viability, migration and differentiation [Citation17]. In addition to above advantage, nano-HAp particulates also showed the function of adsorbing and releasing drugs along with osteogenic and angiogenic effects through loaded icariin [Citation18] and quercetin [Citation19], etc. However, the local application of SrR in animal models of osteoporosis and new nano-HAp particulates loaded SrR has not yet been explored.
The osteoporotic model of ovariectomized rats is an ideal model for testing the treatment of osteoporosis. Compared with the normal rats, the bone marrow mesenchymal stem cells of the ovariectomized rats (OVX-rBMSCs) exhibited less proliferative ability, decreased ALP synthesis and activity, and delayed the secretion of osteocalcin [Citation20]. The pharmacological effects of SrR on OVX rat models have been established [Citation21], while its effect on osteoblast differentiation at cellular level and its possible mechanisms are still relatively shallow. In the present study, RNA-Seq is used to analyse the differentially expressed genes caused by SrR in OVX-rBMSCs and explore the possible signalling pathways. The effect of special gene or SrR on osteogenic differentiation, cell viability, apoptosis and angiogenesis were further validated in OVX-rBMSCs and osteoblasts as well as in OVX rat models.
Materials and methods
Establishment of an OVX rat model and culture of OVX-rBMSCs
Three-month-old female healthy Sprague–Dawley (SD) rats were purchased from Shanghai Super B&K laboratory animal Corp. Ltd. (Shanghai, China), separated into cages and fed laboratory feed and water, and kept under a 12 h light/dark cycle at a constant temperature of 25 °C. The rats were subjected to ovariectomy through two dorsal incisions under anaesthesia. Twelve weeks after the surgery, the rats were sacrificed by cervical dislocation under anaesthesia, and the OVX-rBMSCs were collected from passages 2 to 4 as previously described [Citation22] and used in this study. The OVX-rBMSCs were cultured in DMEM (Thermo Fisher Scientific, Waltham, MA) containing 10% FBS, 100 U/mL penicillin and 100 mg/L streptomycin (Thermo Fisher Scientific) or in osteogenic induction cultural medium (containing dexamethasone, β-glycerophosphate and ascorbic acid) in an incubator at 37 °C, 100% humidity and 5% CO2. This study was performed in strict accordance with the Guide for the Care and Use of Laboratory Animals of the National Institutes of Health (9th edition, 2010) and approved by the Animal Research Committee of the Shanghai Sixth People’s Hospital (Shanghai, China).
Isolation and culture of osteoblasts
New-born SD rats (Shanghai Super-B&K laboratory animal Corp. Ltd, China) were selected to isolate and culture osteoblast. Briefly, SD rats were treated with 75% alcohol for 5–10 min, and the skull were collected and plated into a culture dish containing PBS buffer supplemented with 3 × 105 U/L penicillin and streptomycin [Citation23]. Then skull was digested by 0.25% trypsin and 3–4 mL 0.4% collagenase type I, and added 10% FBS-containing DMEM medium to terminate the digestion. After centrifuged at 2000 rpm for 10 min, the precipitate was resuspended by DMEM medium containing 10% FBS and 1% penicillin and streptomycin. The cells were seeded in 10 cm-diameter plates and cultured at 37 °C in a 5% CO2 incubator. To remove the non-adherent cells, the medium was first changed after 48 h and then renewed every 2–3 days.
RNA-sequencing and bio-informatic analysis
OVX-rBMSCs were cultured in DMEM medium in the absence or presence of 250 μM SrR for 7 days, and the RNA sequencing was performed as previously described [Citation24]. Briefly, poly(A) mRNA was fragmented, and first-strand cDNA was prepared using random hexamers. Following second strand cDNA synthesis, end repair, addition of a single A base, adaptor ligation, agarose gel isolation of 200-bp cDNA, and PCR amplification of the 200-bp cDNA. Changes in gene expression with p values less than .05 and fold change ≥1.5 were considered as statistical significance. Gene set enrichment analysis (GSEA) was performed for identify signalling enriched between control groups (n = 3) and SrR treatment groups (n = 3).
Cell transfection
ROCK1 coding sequence was cloned into the pLVX-Puro vector for constructing pLVX-Puro-ROCK1 expressing vector. The primers were as follow: ROCK1-F 5′-GCGAATTCATGTCGACTGGGGACAGTTTTG-3′ and ROCK1-R 5′-CGGGATCCACTAGTTTTTCCAGATGTATTT-3′. 293T cells were plated in 6-well plates and transfected with 1 ng blank pLVX-Puro or pLVX-Puro-ROCK1 vector, 0.1 ng psPAX2 and 0.9 ng pMD2G for 4–6 using Lipofectamine reagent (Invitrogen, Carlsbad, CA) according to the instruction of the manufacturer. The specific shRNA sequence which targets ROCK1 coding sequence were cloned into the pLKO.1-puro lentiviral vector. The sequences of shRNA were as follow: ROCK1-shRNA-1 (point 745–767), AGGCGGTGATGGCTATTAT; ROCK1-shRNA-2 (point 2762–2784), AGAAACTCTTTCGACTCAG; ROCK1-shRNA-3 (point 3777–3799), GATATAGAAGTGGAACCAG. 293T cells were plated in 6-well plates and transfected with 1 ng pLKO.1-puro-scramble shRNA or pLKO.1-puro-ROCK1-shRNA vector, 0.9 ng psPAX2 and 0.1 ng pMD2G for 4–6 h using Lipofectamine reagent (Invitrogen, Carlsbad, CA) according to the instruction of the manufacturer. After transfection, the free-serum medium was moved and cells were cultured in complete medium. Viruses were collected 48 h post-transfection and infected OVX-rBMSCs or osteoblasts. The blank pLVX-Puro vector and pLKO.1-puro-scramble shRNA was used as negative control.
CCK-8 assay
The cell viability of osteoblasts was measured by CCK-8 assay. Briefly, the osteoblasts (1 × 103 cells/well) were plated in 96-well plates and cultured with 5% CO2 at 37 °C overnight. After (1) treatment with different doses of SrR (10 μM, 100 μM, 500 μM, 1 mM and 2 mM) for 0, 1, 3, 5 and 7 days, (2) transfection with pLVX-Puro-ROCK1 or pLKO.1-puro-ROCK1-shRNA for 0, 24, 48 and 72 h or (3) treatment with SrR (100 μM, 5 days) and/or ROCK 1 inhibitor Y-27632 (5 μL, 24 h) [Citation25], the cells were added with 10 μL CCK-8 solution with 5% CO2 at 37 °C for 1 h. The cell viability was calculated using a microplate reader (ELX 800; Bio-tek Instruments, Winooski, VT, USA) at a wavelength of 450 nm.
Apoptosis analysis
The cell apoptosis of osteoblasts was measured by flow cytometry assay. Briefly, the osteoblasts (3 × 105 cells/well) were plated in 6-well plates and cultured with 5% CO2 at 37 °C overnight. After transfection with pLVX-Puro-ROCK1 or pLKO.1-puro-ROCK1-shRNA for 0, 24, 48 and 72 h, or treatment with SrR (100 μM, 5 days) and/or Y-27632 (5 μL, 24 h), the cells stained with 195 μL Annexin V-FITC and 5 μL propidium iodide. Apoptotic cells were analysed by flow cytometry, by use of a FAC Scan system flow cytometric analysis (Becton-Dickinson FACS Calibur, San Joes, CA) equipped with Cell Quest 3.3 software.
ALP activity
OVX-rBMSCs (1 × 104 cell/well) were plated in 24-well plates and cultured in osteogenic induction cultural medium. After 7 days, the ALP activity of OVX-rBMSCs was measured using an ALP assay kit (Nanjing Jiancheng Bioengineering Institute) according to the manufacturer’s protocol [Citation26,Citation27]. The absorbance at 520 nm was measured using a microplate reader (BioTek Instruments).
Quantitative real-time PCR
Whole RNA was extracted from OVX-rBMSCs or osteoblasts by RNAiso Plus (TaKaRa, Dalian, People’s Republic of China), and PrimeScript reagent kit of reverse reaction (DRR037A, TaKaRa) was used to carry out reverse transcription reaction on RNA in accordance with protocols of manufacturer. Quantitative analysis on the change of expression level was conducted by Maxima SYBR Green/ROX qPCR Master Mix (cat. no. K0223; Finnzymes; Thermo Fisher Scientific, Inc.) in ABI 7500 (Thermo Fisher Scientific, Waltham, MA, USA). The primer sequences were shown subsequently in . The change in expression of mRNA within OVX-rBMSCs or osteoblasts was assessed by the 2−ΔΔCt approach.
Table 1. Primers sequences used in this study.
Western blotting
Total protein was extracted from OVX-rBMSCs or osteoblasts with RIPA lysis buffer supplemented with protease inhibitors. The concentration of protein was estimated employing the assay kit of the bicinchoninic acid (Thermo Fisher Scientific, Inc.). Equal quantities of protein (15 μL) got divided subsequently through 10% SDS-PAGE gels, and then were subsequently moved onto the membranes of polyvinylidene fluoride (EMD Millipore, Billerica, MA). After blocking, these membranes got immunoblotted overnight at 4 °C with first antibodies: anti-ALP, anti-Runx-2, anti-OPN, anti-PCNA, anti-Bcl-2, anti-Bax and anti-GAPDH. Horseradish peroxidase-conjugated secondary antibodies (1:1000; Beyotime Institute of Biotechnology, Inc.) were used to incubate membranes after they were washed for 1 h at 37 °C. Tris-buffered saline including 20% Tween 20 (Amresco, LLC, Solon, OH) was used to wash these membranes. Detection for signals employed an improved system of chemiluminescence system (Pierce, Rockford, IL) and determination for them employed software of Image J software version 1.46.
Preparation of SrR loaded nano-HAp particulates
The nano-HAp particulates were fabricated by the hydrothermal transformation method using α-tricalcium phosphate [α-Ca3(PO4)2, α-TCP] particulates with 300–450 µm size as precursors according to our previous study [Citation18,Citation28]. The morphology of the obtained nano-HAp particles was observed using a field emission scanning electron microscopy (FESEM). The characteristics of the obtained nano-HAp particles was characterized by X-ray diffraction (XRD: D/max 2550 V, Rigaku, Japan) using monochromated Cu-Kα radiation and Fourier transform infrared spectroscopy (FTIR).
The loading and sustained releasing of SrR
About 0.1 g of the obtained nano-HAp particles containing 80 μL SrR at concentrations of 2 mM was immersed in 2 mL PBS at 37 °C [Citation18]. The supernatant was then refreshed and collected at 2 h, 4 h, 8 h, 24 h, 3 d, 5 d, 7 d, 9 d, 11 d, 13 d, 15 d, 17 d, 19 d and 21 d, respectively. The release of SrR was quantified by ultraviolet spectrophotometry.
Animal surgery
The femoral condyle bone defect model of ovariectomy rat (OVX rat model) was made as previously described [Citation18,Citation19]. Briefly, incisions on the both of distal side of femoral condyle were made after fully anaesthesia, and exposed the bone surface clearly. Then, a defect of 3.5 mm diameter, 5 mm in depth were made by a slow speed trephine drill with saline solution of cooling. Twenty OVX SD rats were randomly allocated into two groups: one administered nano-HAp particles without SrR (Control, n = 10) and one administered nano-HAp particles loaded with SrR at a concentration of 200 mM (n = 10).
Sequential fluorescent labelling
To observe the new bone formation and mineralization of bone regeneration within the defect area, those rats for 8 weeks observation time were injected every 2 weeks with the sequence of tetracycline hydrochloride (TE, Sigma), alizarin red (AL, Sigma) and calcium (CA, Sigma) at the dosage of 25–30 mg/kg.
Microfil perfusion and microcomputed tomography (micro-CT) measurement
Rats of 8 weeks time point were euthanatized by excessive dosage of phenobarbitione, then exposed the heart, and opened the left ventricular and right atrium immediately. 20 ml of heparinized saline, 20 ml paraformaldehyde and 20 ml of Microfil (Flowtech, Carver, MA) were perfused into blood vessel. Samples were collected after 24 h of refrigerate storage, and routine decalcified by EDTA-NA2 for 2 weeks. Samples fixed with 4% formaldehyde and fully decalcified of those perfused with Microfil were examined by micro-CT (skyscan1176, Bruker, Belgium). The measurements were similar as previous described [Citation18,Citation19]. For the samples only fixed with formaldehyde, the interesting areas were focus on the bone tissue, the bone mineral density (BMD), bone volume/tissue volume (BV/TV), trabecular thickness (Tb.Th) and trabecular number (Tb.N) were measured. For the samples of microfilm perfusion, the interesting areas were selected to the blood vessel, vascular volume fractions were calculated regarding to the value of BV/TV.
Histological and histomorphometric observation
The samples of femur were fixed with 4% formaldehyde at 4 °C for 2 days, then paraffin section or hard tissue slices were made. The paraffin sections were stained with Haematoxylin & Eosin (H&E), Tartrate Resistant Acid Phosphatase (TRAP) and CD31 immunohistochemistry staining for histological observation. The hard tissue slices were observed under confocal microscopy for the sequential fluorescent labelling.
Statistical analysis
Data were expressed as the mean ± SD and analysed with SPSS 18.0 software. The significant differences were assessed by one-way analysis of variance (ANOVA). p < .05 was considered statistically significant.
Results
Gene expression differed in OVX-rBMSCs
Although our previous study has reported that SrR could promote osteogenic differentiation and angiogenic factor expression of OVX-rBMSCs [Citation29], the underlying mechanisms involved have not been fully understood. First, we compared the gene-expression difference between control OVX-rBMSCs (n = 3) and OVX-rBMSCs with SrR treatment (n = 3) using RNA-sequencing analysis. We identified 90 out of 12,330 transcripts exhibited distinct expression patterns between control and SrR (). About 41 of the transcripts were increased in OVX-rBMSCs with SrR treatment. Among these transcripts, the five highest increase were Exoc7 (17.11-fold), Dhrs9 (14.46-fold), Cyp4f37 (10.86-fold), Ly6g5b (7.15-fold) and Kcns3 (7.07-fold). On the other hand, transcripts showed the five highest decrease were Nrg4 (0.12-fold), LOC100911576 (0.15-fold), Mir1956 (0.15-fold), Cecr2 (0.17-fold) and Brms1 (0.21-fold). Interestingly, ROCK1 which has been shown to promote osteogenesis was also found increased expression in OVX-rBMSCs with SrR treatment compared with the control OVX-rBMSCs (1.55-fold). To further determine the signalling pathways that were altered in OVX-rBMSCs, GSEA was performed. We found that ROCK1 was involved in two signalling pathways, including TGF-β signalling pathway (p = .015) and chemokine signalling pathway (p = .024) (), and the GSEA details in OVX-rBMSCs with SrR treatment and the control OVX-rBMSCs involving TGF-β signalling pathway () and chemokine signalling pathway () were also shown. These findings suggested that ROCK1 may associate with the effects of SrR on the osteogenic differentiation of OVX-rBMSCs and the role of ROCK1 is therefore further confirmed.
Figure 1. The signalling pathways that associated with ROCK1 in control and SrR treated OVX-rBMSCs. (a, b) The signalling pathways that associated with ROCK1 in control (n = 3) and SrR treated OVX-rBMSCs (n = 3) were analysed by GSEA, and GSEA details of TGF-β and chemokine signalling pathway were shown in C and D. * indicates the ROCK1 expression.
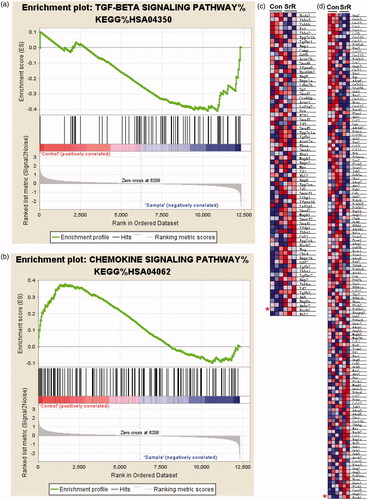
Table 2. Gene expression differed in OVX-rBMSCs treated with SrR or not.
SrR treatment increases the expression of ROCK1 in OVX-rBMSCs and osteoblasts
SrR with the concentrations of 10, 100 and 500 μM, according to our previous study [Citation29], was used in the present study, and the expression of ROCK1 in response to SrR in OVX-rBMSCs was validated by real-time PCR and western blotting. We found that SrR treatment for 5 and 7 days significantly increased the mRNA and protein expression of ROCK1 in OVX-rBMSCs (). To further investigate the role of SrR in osteoblasts, the CCK-8 assay was performed to detect the cell viability of osteoblasts. As shown in , SrR with the low concentration (10 and 100 μM) significantly increased the viability of osteoblasts at day 3, 5 and 7. However, SrR with the high concentration (500 μM, 1 mM and 2 mM) significantly decreased the viability of osteoblasts in a time-dependent manner. Therefore, SrR with the concentrations of 10, 100 and 500 μM was used for the following experiment. Our results showed that SrR treatment for 5 and 7 days also significantly increased the mRNA and protein expression of ROCK1 in osteoblasts ()). These data suggest that ROCK1 is associated with the regulation of SrR in the osteogenic differentiation of OVX-rBMSCs and viability of osteoblasts.
Figure 2. SrR treatment increased ROCK1 expression and viability of osteoclasts. The expression of ROCK1 in OVX-rBMSCs was measured by real-time PCR (a) and western blotting (b, c). The cell viability of osteoclasts and the expression of ROCK1 in OVX-rBMSCs were measured by CCK-8 (d), real-time PCR (e) and western blotting (b, f). *p < .05, **p < .01 compared with control.
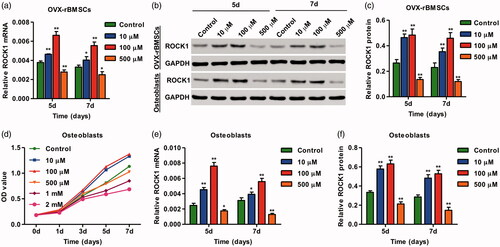
ROCK1 regulates the osteogenic differentiation ofOVX-rBMSCs
To test the osteogenic ability of OVX-rBMSCs with ROCK1, cultural medium without osteogenic induction supplements were used, lentivirus vector of pLVX-Puro-ROCK1 and pLKO.1-puro-ROCK1-shRNA was constructed and the ALP activity as well as the expression of Runx-2, ALP and OPN was measured by real-time PCR and western blotting. As shown in , pLVX-Puro-ROCK1 transfection in OVX-rBMSCs significantly increased the mRNA expression of ROCK1 by 9.11-fold, while three pLKO.1-puro-ROCK1-shRNAs transfection in OVX-rBMSCs significantly decreased the mRNA expression of ROCK1 by 78.03, 87.16 and 67.37%, respectively, and pLKO.1-puro-ROCK1-shRNA-2 was therefore used for our following experiments. Moreover, pLVX-Puro-ROCK1 transfection significantly increased the ALP activity as well as the mRNA and protein expression of Runx-2, ALP and OPN in OVX-rBMSCs, which was inhibited by pLKO.1-puro-ROCK1-shRNAs transfection (). These results indicate that ROCK1 up-regulation can promote the osteogenic differentiation of OVX-rBMSCs.
Figure 3. ROCK1 regulated the osteogenesis of OVX-rBMSCs. After transfection of OVX-rBMSCs with pLVXPuro-ROCK1 (a) and pLKO.1-puro-ROCK1-shRNA (b), the expression of ROCK1 was measured by real-time PCR, the activity of ALP was shown in (c), and the expression of Runx-2, ALP and OPN was measured by real-time PCR (d) and western blotting (e, f). **p < .01 compared with Vector. ##p < .01 compared with shRNA.
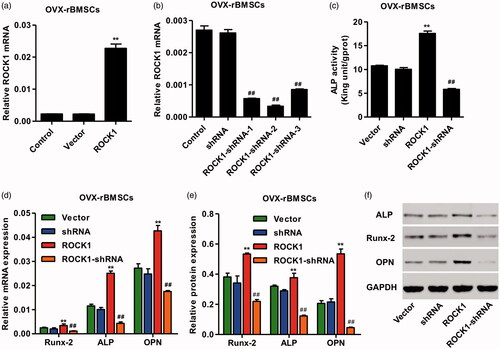
ROCK1 regulates the viability and apoptosis of osteoblasts
To investigate the role of ROCK1 in viability and apoptosis of osteoblasts, the lentivirus vector of pLVX-Puro-ROCK1 and pLKO.1-puro-ROCK1-shRNA was also constructed, and the CCK-8 and flow cytometry assay were measured. As shown in , pLVX-Puro-ROCK1 transfection in osteoblasts significantly increased the mRNA expression of ROCK1 by 13.43-fold, while three pLKO.1-puro-ROCK1-shRNAs transfection in OVX-rBMSCs significantly decreased the mRNA expression of ROCK1 by 70.60, 80.47 and 75.06%, respectively, and pLKO.1-puro-ROCK1-shRNA-2 was therefore used for our following experiment. Moreover, pLVX-Puro-ROCK1 transfection significantly increased the viability and inhibited apoptosis of osteoblasts, which were reversed by pLKO.1-puro-ROCK1-shRNAs transfection in osteoblasts (). The expression of viability and apoptosis-related genes measured by real-time PCR and western blotting showed that pLVX-Puro-ROCK1 transfection significantly increased the mRNA and protein expression of ALP, PCNA and Bcl-2, but decreased that of Bax. However, pLKO.1-puro-ROCK1-shRNAs transfection significantly decreased the mRNA and protein expression of ALP, PCNA and Bcl-2, but increased that of Bax in osteoblasts (). These results indicate that ROCK1 up-regulation can promote the viability and inhibit apoptosis of osteoblasts.
Figure 4. ROCK1 regulated the viability and apoptosis of osteoclasts. After transfection of osteoclasts with pLVX-Puro-ROCK1 (a) and pLKO.1-puro-ROCK1-shRNA (b), the expression of ROCK1 was measured by real-time PCR, the cell viability and apoptosis was measured by CCK-8 (c) and flow cytometry (d, e), and the expression of ALP, PCNA, Bcl-2 and Bax was measured by western blotting (f, g) and real-time PCR (h). **p < .01 compared with Vector. ##p < .01 compared with shRNA.
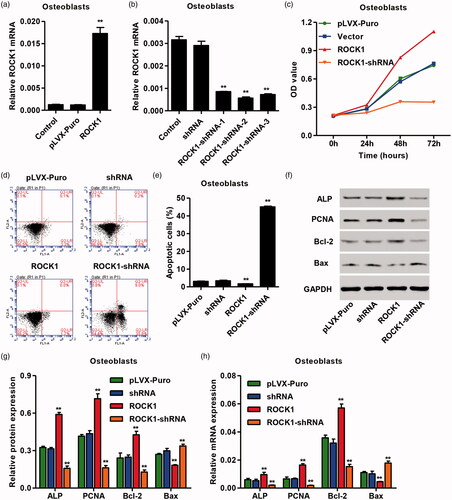
Inhibition of ROCK1 suppresses SrR-induced osteogenic differentiation of OVX-rBMSCs and viability and apoptosis of osteoblasts
Consistent with previous study that SrR could promote osteogenic differentiation of OVX-rBMSCs, we also found increased ALP activity and the mRNA and protein expression of Runx-2, ALP and OPN in OVX-rBMSCs with SrR treatment compared with control. However, treatment of OVX-rBMSCs with ROCK1 inhibitor Y-27632 significantly decreased the ALP activity and the mRNA and protein expression of Runx-2, ALP and OPN in OVX-rBMSCs compared with control. Meanwhile, SrR together with Y-27632 treatment markedly inhibited the effects of individual treatment with SrR or Y-27632 on the ALP activity and the expression of Runx-2, ALP and OPN (). Moreover, the effects of SrR and Y-27632 on the viability and apoptosis of osteoblasts were also examined. SrR treatment obviously increased the viability and inhibited apoptosis of osteoblasts (), and the expression of ALP, PCNA and Bcl-2 was also increased after SrR treatment with except decreased Bax expression (). However, Y-27632 had the exact opposite of what SrR observed. Meanwhile, SrR together with Y-27632 treatment markedly inhibited the effects of individual treatment with SrR or Y-27632 on the viability and apoptosis of osteoblasts ().
Figure 5. Effects of SrR and Y-27632 on the osteogenesis of OVX-rBMSCs and the viability and apoptosis of osteoclasts. After treatment of OVX-rBMSCs and osteoclasts with SrR and/or Y-27632, the ALP activity (a) as well as the gene expression of Runx-2, ALP and OPN (b) in OVX-rBMSCs was measured. The protein expression of RUNX-2 ALP and OPN was measured by western blotting (i, j). The cell viability and apoptosis was measured by CCK-8 (e) and flow cytometry (f, g), and the expression of ALP, PCNA, Bcl-2 and Bax in osteoclasts was measured by real-time PCR (h) and western blotting (i, j). **p < .01 compared with control. ##p < .01 compared with SrR. ΔΔp < .01 compared with Y-27632.
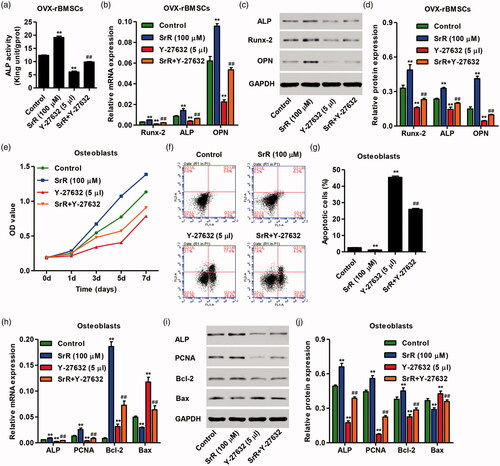
Characterization of nano-HAp particulates
As shown in , the SEM image with low magnification showed that the obtained nano-HAp particulates were irregular in shape with size of 300–450 μM. High-magnification imaging further revealed that the particulates hydrothermally transformed from α-TCP ceramic particulate precursors in deionized water were composed of nano-rods with diameter of approximately 60–100 nm and length of up to 10 μM. The XRD and FTIR results confirmed that the fabricated particles was pure HAp phase, the results also suggested that the α-TCP precursors were completely converted to HAp (). The SrR release from particulates was presented as percentage. As shown in , the release amount of SrR reached 70% at 8 h, approximately 100% at the 14 days. These results suggested that nano-HAp, as drug delivery vehicles, could provide sustained release kinetics.
Implantation of nano-HAp particulates loaded with SrR promotes new bone formation and angiogenesis
After implantation of rats with nano-HAp particulates, the rats had no wound infection and death. At 4 and 8 weeks, the wound healing was satisfactory (). The micro-CT results showed that the levels of BMD and Tb.N were significantly increased after implantation of nano-HAp particulates loaded with SrR at 4 weeks compared with implantation of nano-HAp particulates without SrR (). After 8 weeks of implantation, the nano-HAp particulates loaded with SrR, the levels of BMD, BV/TV, Tb.Th and Tb.N were significantly increased. Three-dimensional reconstruction of blood vessel showed that the amount of blood vessel growth in SrR groups was higher than that in controls; however, the difference was not significant (). Nevertheless, immunohistochemical staining in SrR groups showed significantly increased CD31 expression at 4 weeks after operation compared with control groups ().
Figure 7. Micro-CT evaluation and morphometric analysis of femur bone repair and angiogenesis. (a) The wound healing was satisfactory after surgery. (b) Representative 3D superficial image of femur bone defects. (c–f) Quantitative analysis of micro-CT data. (g) Three-dimensional reconstruction of blood vessel. The left two pictures show the three-dimensional reconstructions of blood vessel tissue in control and SrR groups, the picture on the top right corner presents the direction of the 3D reconstruction pictures, and the down right corner shows the quantitative data. (h, i) CD31 immunohistochemical staining and quantitative data. *p < .05 compared with control.
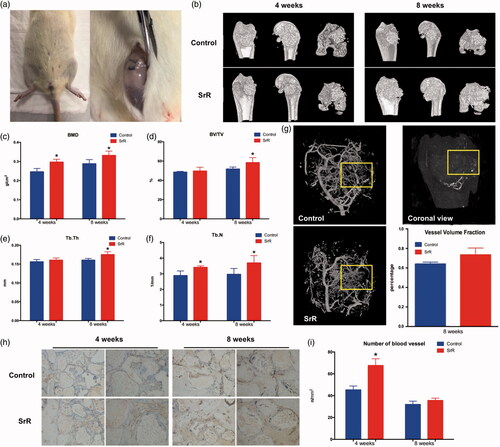
Implantation of nano-HAp particulates loaded with SrR promotes bone regeneration
In , the different fluorescent labels of TE, AL and CA represented that SrR promoted new bone formation and mineralization at weeks 2, 4 and 6 after operation. The analysis of H&E staining showed that only limited new bone tissue penetrated into the defect bottom of the control groups, whereas more newly formed bone formation was observed on the defect top of the SrR groups at weeks 8 after operation (). Moreover, TRAP staining showed that the number of osteoclasts was decreased at week 8 compared with that at week 4 after operation at both control and SrR groups, and even decreased in SrR groups compared with control groups at week 8 after operation ().
Figure 8. Sequential fluorescence labelling and histological images of newly formed bone in femur defects. (a) Confocal laser scanning of sequential fluorescent labelling on new bones. The yellow fluorescence represents the tetracycline (TE) of 2 weeks, the red fluorescence represents alizarin red (AL) of 4 weeks and the green represent the calcein (CA) of 6 weeks. The last two pictures showed the sections under light microscope (magnification 100×). (b, c) The histograms showed the result of qualitative analysis. (d, e) H&E staining and quantitative analysis. The left rows are in magnification 12.5×, right rows are in magnification 100×. (f, g) TRAP staining and quantitative analysis. The left rows are in magnification 100×, right rows are in magnification 200×. *p < .05 compared with control.
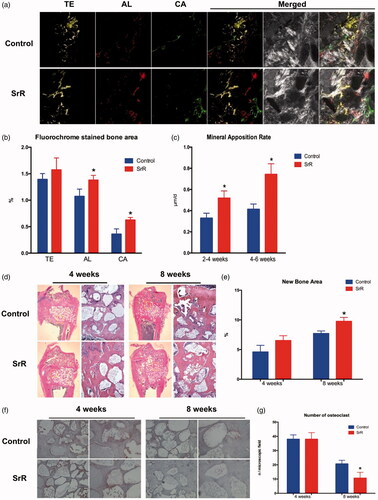
Discussion
SrR, a poorly soluble drug, is rarely studied for its direct action on cells. Previous study showed that SrR had significant osteogenic effect in the range of concentration from 250 μM to 1.0 mM, with the significant effect detected at the concentration of 250 μM [Citation29]. Therefore, the OVX-rBMSCs were treated with 250 μM SrR for 7 days and followed by RNA-sequencing analysis. We identified 90 out of 12,330 transcripts exhibited distinct expression patterns between control and SrR groups (p < .05 and fold change ≥1.5). Among those, ROCK1 was increased in SrR groups compared with control groups (fold change =1.55) and associated with the TGF-β signalling pathway and chemokine signalling pathway. Previous study has reported that RhoA-ROCK signalling pathway plays an important role in the cell migration and adhesion, which are of great importance in the adipogenic differentiation and osteogenic differentiation [Citation30]. Moreover, Wang et al. [Citation31], also showed that BMP-2 promoted osteogenic differentiation of BMSCs through activating RhoA-ROCK signalling pathway. These findings suggest that ROCK1 may involve in the osteogenesis of OVX-rBMSCs induced by SrR.
According to our previous study, OVX-rBMSCs with high concentrations of SrR (≥1 mM) treatment for 7 days showed inhibition of cell viability, while that with low concentrations of SrR (<500 μM) showed promotion of cell viability [Citation29]. In the present study, Real-time PCR and western blotting assay validated that SrR treatment significantly increased the expression of ROCK1 in OVX-rBMSCs at the concentration of 10 and 100 μM, while decreased ROCK1 expression was found in SrR at the concentration of 500 μM, which was consistent with the previous study [Citation29]. Moreover, the cell viability and ROCK1 expression of osteoblasts in response to SrR were also measured. Our results demonstrated that low concentrations of SrR (10 and 100 μM) significantly increased the cell viability and ROCK1 expression, while high concentration of SrR (500 μM, 1 mM and 2 mM) showed inhibitory effects. It is worth noting that previous studies mostly focused around the concentration of 1.0 mM as the optimum concentration [Citation32,Citation33], while 100 μM was served as the optimum concentration in our study. This is mainly due to the difference in the method for drug treatment and the cell model [Citation3,Citation16].
RhoA-ROCK signal pathway is one of the most important pathways in cell kinetics, plays a crucial role in cell adhesion, cell migration and cell skeleton and is involved in the regulation of metabolic behaviour, including endothelial permeability, tissue growth and contraction [Citation34,Citation35]. In the present study, ROCK1 up-regulation markedly promoted osteogenic differentiation of OVX-rBMSCs along with promoted viability and inhibited apoptosis of osteoblasts. The ALP activity and expression of Runx-2, ALP and OPN were significantly increased in OVX-rBMSCs after ROCK1 up-regulation, and the expression of ALP, PCNA and Bcl-2/Bax ratio was also increased in osteoblasts. In line with previous study, there is a great deal of intersection between RhoA-ROCK and ERK-MAPK pathway, which resulted in the activation of ERK1/2 and followed by increased expression of downstream Runx-2 as well as bone formation markers [Citation36]. However, inhibition of ROCK1 by RNA interference or its inhibitor Y-27632 treatment obviously inhibited osteogenic differentiation of OVX-rBMSCs as well as the viability and apoptosis of osteoblasts induced by SrR treatment or not. These results indicate that SrR promotes osteogenic differentiation of OVX-rBMSCs as well as promotes the viability and apoptosis of osteoblasts through up-regulation of ROCK1.
Drug loading and release on nano-HAp particulates are commonly used for drug delivery [Citation37]. A number of studies have been carried out on drug delivery on nano-HAp to achieve bone induction and have achieved good effects [Citation17,Citation18]. In vivo experiments demonstrated that implantation of nano-HAp particulates loaded with SrR could promote osteogenesis in femoral condyle bone defect model of ovariectomy rat. After 8 weeks of implantation nano-HAp particulates loaded with SrR, the levels of BMD, BV/TV, Tb.Th and Tb.N and the mineral apposition rate were significantly increased, suggested that SrR treatment promoted new bone formation and bone regeneration. Moreover, TRAP staining showed that the number of osteoclast in SrR groups was decreased at week 8 compared with control groups, indicated that SrR inhibited osteoclast activity in a long-term mechanism. In addition to its good biocompatibility and bone induction effects, HAp also has a good effect on promoting angiogenesis. On the one hand, the high surface energy of nano-HAp showed obvious protein adsorption and absorbed a large amount of fibronectin and growth factors in the matrix, which promoted cell adhesion and migration [Citation38]. On the other hand, the stent action of HAp guarantees the smooth climb of the cells into the defect [Citation39]. Our CD31 immunohistochemistry analysis showed the presence of a large number of small vessels and capillaries in the bone defect area, suggesting that local application of SrR can promote angiogenesis and play an important role in optimizing and accelerating tissue regeneration.
In conclusion, SrR could promote the osteogenic differentiation of OVX-rBMSCs as well as promote the viability and inhibit the apoptosis of osteoblasts through up-regulating ROCK1 expression. Furthermore, SrR could be released in a controlled manner by nano-HAp particles and nano-HAp particles loaded with SrR could promote both osteogenesis and angiogenesis in vivo.
Disclosure statement
The authors declare no conflicts of interest.
Additional information
Funding
References
- Curtis EM, Moon RJ, Dennison EM, et al. Recent advances in the pathogenesis and treatment of osteoporosis. Clin Med (London, England). 2015;15 Supplement 6:s92–s96.
- Devlin H, Allen PD, Graham J, et al. Automated osteoporosis risk assessment by dentists: a new pathway to diagnosis. Bone. 2007;40:835–842.
- Meunier PJ, Roux C, Ortolani S, et al. Effects of long-term strontium ranelate treatment on vertebral fracture risk in postmenopausal women with osteoporosis. Osteoporos Int. 2009;20:1663–1673.
- Cianferotti L, D'Asta F, Brandi ML. A review on strontium ranelate long-term antifracture efficacy in the treatment of postmenopausal osteoporosis. Ther Adv Musculoskelet Dis. 2013;5:127–139.
- Atkins GJ, Welldon KJ, Halbout P, et al. Strontium ranelate treatment of human primary osteoblasts promotes an osteocyte-like phenotype while eliciting an osteoprotegerin response. Osteoporos Int. 2009;20:653–664.
- Fromigue O, Hay E, Barbara A, et al. Essential role of nuclear factor of activated T cells (NFAT)-mediated Wnt signaling in osteoblast differentiation induced by strontium ranelate. J Biol Chem. 2010;285:25251–25258.
- Caverzasio J. Strontium ranelate promotes osteoblastic cell replication through at least two different mechanisms. Bone. 2008;42:1131–1136.
- Fromigue O, Hay E, Barbara A, et al. Calcium sensing receptor-dependent and receptor-independent activation of osteoblast replication and survival by strontium ranelate. J Cell Mol Med. 2009;13:2189–2199.
- Lv H, Huang X, Jin S, et al. Strontium ranelate promotes osteogenic differentiation of rat bone mesenchymal stem cells through bone morphogenetic protein-2/Smad signaling pathway. J South Med Univ. 2013;33:376–381.
- Peng S, Zhou G, Luk KD, et al. Strontium promotes osteogenic differentiation of mesenchymal stem cells through the Ras/MAPK signaling pathway. Cell Physiol Biochem. 2009;23:165–174.
- Jin SS, Jie-Fen HU, Wen WU, et al. Strontium renelate promotes osteogenesis via regulation of sonic Hedgehog signaling molecules in rat bone marrow mesenchymal stem cells. Chin JPathophysiol. 2014;30:159–164.
- Lin K, Liu P, Wei L, et al. Strontium substituted hydroxyapatite porous microspheres: surfactant-free hydrothermal synthesis, enhanced biological response and sustained drug release. Chem Eng J. 2013;222:49–59.
- Lin K, Xia L, Li H, et al. Enhanced osteoporotic bone regeneration by strontium-substituted calcium silicate bioactive ceramics. Biomaterials. 2013;34:10028–10042.
- Liu F, Zhang X, Yu X, et al. In vitro study in stimulating the secretion of angiogenic growth factors of strontium-doped calcium polyphosphate for bone tissue engineering. J Mater Sci Mater Med. 2011;22:683–692.
- Gu Z, Xie H, Li L, et al. Application of strontium-doped calcium polyphosphate scaffold on angiogenesis for bone tissue engineering. J Mater Sci Mater Med. 2013;24:1251–1260.
- Yamaguchi M, Weitzmann MN. The intact strontium ranelate complex stimulates osteoblastogenesis and suppresses osteoclastogenesis by antagonizing NF-κB activation. Mol Cell Biochem. 2012;359:399–407.
- Lin K, Xia L, Gan J, et al. Tailoring the nanostructured surfaces of hydroxyapatite bioceramics to promote protein adsorption, osteoblast growth, and osteogenic differentiation. ACS Appl Mater Interfaces. 2013;5:8008–8017.
- Wu Y, Xia L, Zhou Y, et al. Evaluation of osteogenesis and angiogenesis of icariin loaded on micro-nano hybrid structured hydroxyapatite granules as a local drug delivery system for fermoral defect repair. J Mater Chem B. 2015;3:4871–4883.
- Zhou Y, Wu Y, Ma W, et al. The effect of quercetin delivery system on osteogenesis and angiogenesis under osteoporotic conditions. J Mater Chem B. 2017;5:612–625.
- Tewari D, Khan MP, Sagar N, et al. Ovariectomized rats with established osteopenia have diminished mesenchymal stem cells in the bone marrow and impaired homing, osteoinduction and bone regeneration at the fracture site. Stem Cell Rev. 2015;11:309–321.
- Bain SD, Jerome C, Shen V, et al. Strontium ranelate improves bone strength in ovariectomized rat by positively influencing bone resistance determinants. Osteoporos Int. 2009;20:1417–1428.
- Shen Q, Zeng D, Zhou Y, et al. Curculigoside promotes osteogenic differentiation of bone marrow stromal cells from ovariectomized rats. J Pharm Pharmacol. 2013;65:1005–1013.
- Torricelli P, Fini M, Giavaresi G, et al. Osteoblasts cultured from osteoporotic bone: a comparative investigation on human and animal-derived cells]. Artif Cells Blood Substit Immobil Biotechnol. 2003;31:263–277.
- Toung JM, Morley M, Li M, et al. Rna-sequence analysis of human b-cells. Genome Res. 2011;21:991.
- Ishizaki T, Uehata M, Tamechika I, et al. Pharmacological properties of Y-27632, a specific inhibitor of rho-associated kinases[J]. Mol Pharmacol. 2000;57:976–983.
- Du X, Huang F, Zhang S, et al. Carboxymethylcellulose with phenolic hydroxyl microcapsules enclosinggene-modified BMSCs for controlled BMP-2 release in vitro[J]. Artif Cells Nanomed Biotechnol 2017;45:1710–1720.
- Han TY, Liu XW, Liang N, et al. In vitro effects of recombinant adenovirus-mediated bone morphogenetic protein 2/vascular endothelial growth factor 165 on osteogenic differentiation of bone marrow mesenchymal stem cells[J]. Artif Cells Nanomed Biotechnol. 2017;45:108–114.
- Xia L, Lin K, Jiang X, et al. Effect of nano-structured bioceramic surface on osteogenic differentiation of adipose derived stem cells. Biomaterials. 2014;35:8514–8527.
- Guo X, Wei S, Lu M, et al. Dose-dependent effects of strontium ranelate on ovariectomy rat bone marrow mesenchymal stem cells and human umbilical vein endothelial cells. Int J Biol Sci. 2016;12:1511–1522.
- McBeath R, Pirone DM, Nelson CM, et al. Cell shape, cytoskeletal tension, and Rhoa regulate stem cell lineage commitment. Dev Cell. 2004;6:483–495.
- Wang YK, Yu X, Cohen DM, et al. Bone morphogenetic protein-2-induced signaling and osteogenesis is regulated by cell shape, Rhoa/Rock, and cytoskeletal tension. Stem Cells Dev. 2012;21:1176–1186.
- Brennan TC, Rybchyn MS, Green W, et al. Osteoblasts play key roles in the mechanisms of action of strontium ranelate. Br J Pharmacol. 2009;157:1291–1300.
- Chen YW, Shi GQ, Ding YL, et al. In vitro study on the influence of strontium-doped calcium polyphosphate on the angiogenesis-related behaviors of HUVECs. J Mater Sci Mater Med. 2008;19:2655–2662.
- Oviedo PJ, Sobrino A, Laguna-Fernandez A, et al. Estradiol induces endothelial cell migration and proliferation through estrogen receptor-enhanced Rhoa/Rock pathway. Mol Cell Endocrinol. 2011;335:96–103.
- Cao X, Luo T, Luo X, et al. Resveratrol prevents angii-induced hypertension via ampk activation and Rhoa/Rock suppression in mice. Hypertens Res. 2014;37:803–810.
- Khatiwala CB, Kim PD, Peyton SR, et al. Ecm compliance regulates osteogenesis by influencing MAPK signaling downstream of Rhoa and Rock. J Bone Miner Res. 2009;24:886–898.
- Okada M, Furuzono T. Hydroxylapatite nanoparticles: fabrication methods and medical applications. Sci Technol Adv Mater. 2012;13:064103.
- Pezzatini S, Solito R, Morbidelli L, et al. The effect of hydroxyapatite nanocrystals on microvascular endothelial cell viability and functions. J Biomed Mater Res A. 2006;76:656–663.
- Chang CS, Su CY, Lin TC. Scanning electron microscopy observation of vascularization around hydroxyapatite using vascular corrosion casts. J Biomed Mater Res. 1999;48:411–416.