Abstract
Long segment tracheal stenosis often has a poor prognosis due to the limited availability of materials for tracheal reconstruction. Tissue engineered tracheal patches based on electrospun scaffolds and stem cells present ideal solutions to this medical challenge. However, the established engineering process is inefficient and time-consuming. In our research, to optimize the engineering process, core–shell nanofilms encapsulating TGF-β3 were fabricated as scaffolds for tracheal patches. The morphological and mechanical characteristics, degradation and biocompatibility of poly(l-lactic acid-co-ε-caprolactone)/collagen (PLCL/collagen) scaffolds with different compositions (PLCL:collagen 75:25, 50:50 and 25:75, respectively) were comparatively evaluated to determine the preferable compositional ratio. Then the chondrogenesis-inducing potential is investigated, and tracheal patches based on electrospun scaffolds and bone marrow mesenchymal stem cells (BMSCs) were constructed to restore tracheal defects in rabbit models. The results indicated that core–shell scaffolds with a PLCL/collagen proportion of 75:25 were eligible for tracheal patches. The stable and sustained release of TGF-β3 from scaffolds could efficiently promote the chondrogenic differentiation of BMSCs and shorten the incubation time. Tracheal integrity was well maintained for 2 months after restoration; meanwhile, re-epithelialization also achieved. In conclusion, TGF-β3-encapsulating core–shell electrospun scaffolds with a PLCL/collagen proportion of 75:25 could be used to optimize engineering process of tracheal patches.
Introduction
Tracheal stenosis is usually caused by congenital factors, trauma, inflammation and malignant neoplasia within the airway. For patients with a long, narrow airway, side-slide tracheoplasty is clinically recommended. However, postoperative complications, such as restenosis and anastomotic leakage, often easily arise because of the overwhelming tension of anastomosis [Citation1,Citation2]. In this condition, autografts, allografts and prosthetic materials have been utilized as patches for airway reconstruction [Citation3–5]. However, the growth potential and mechanical stability of these patches are so limited that severe tracheal stenosis still has a poor prognosis [Citation4].
Tissue engineered tracheal patches provide ideal solutions to this medical challenge, due to their potent growth potential [Citation6]. The essential components of tissue engineering include scaffolds and seed cells. Electrospun nanofibers compare favorably with the native structure of extracellular matrix (ECM), and they also possess excellent mechanical properties and biodegradability. Thus, electrospun scaffolds have been utilized in a series of tissue engineering applications, such as engineered vessel, skin, bone and cartilage [Citation7,Citation8]. In our previous research, tissue engineered cartilage, which is promisingly used for tracheal patches, has been successfully constructed with electrospun scaffolds and bone marrow mesenchymal stem cells (BMSCs) [Citation9,Citation10]. Nevertheless, chondrogenic incubation in vitro was very time-consuming and inefficient, prolonging the total time for patch construction [Citation11,Citation12]. Moreover, the induction factors were rapidly inactivated in the culture microenvironment.
In contrast, we were inspired by coaxial electrospinning, which can fabricate nanofibers with core–shell structures for the controlled delivery of bioactive agents [Citation13]. Induction factors can be encapsulated into the scaffolds of tracheal patches and then sustainably released after implantation to guide the chondrogenic differentiation of seed cells, and thus, the engineering process can be improved consequently. In our pilot study, core–shell scaffolds encapsulating transforming growth factor β3 (TGF-β3) were fabricated, and bioactive TGF-β3 was released in vitro [Citation14]. Whether these scaffolds can be utilized to construct tracheal patches and optimize the engineering process still needs further exploration.
In this research, TGF-β3-encapsulating core–shell scaffolds with different compositional ratios were fabricated, and their characterizations were comparatively evaluated to determine the preferable constituent proportion for tracheal patches. Then, the chondrogenesis inducing potential and feasibility of restoring tracheal defects with the engineered patches were evaluated in rabbit models. Use this style when you need to begin a new paragraph.
Materials and methods
Animals
Adult nude mice (8 weeks old) were purchased from Lingchang Biotechnology Co., Ltd. (Shanghai, China). Adult New Zealand white rabbits (8 weeks old) were purchased from Songlian Laboratory Animal Company (Shanghai, China). All experimental protocols were approved by the Animal Care and Experiment Committee of Shanghai Jiao Tong University School of Medicine.
Reagents
PLCL with a molar ratio of 75% l-lactide, and collagen type I (molecular weight 0.8–1.0 × 105 Da) were purchased from Sigma-Aldrich (St Louis, MO). Recombinant human TGF-β3 and relevant ELISA kit, and primary antibody to cytokeratin 5/8 were purchased from R&D Systems (Minneapolis, MN). α-MEM, penicillin and streptomycin were purchased from HyClone (Logan, UT). Helios knockout serum replacement (KOSR) was purchased from AventaCell BioMedical Co., Ltd. (Atlanta, GA). Actin-stain 555 phalloidin was purchased from Cytoskeleton. Inc. (Denver, CO).
Scaffold fabrication
Different mass ratios of PLCL and collagen (PLCL:collagen 75:25, 50:50 and 25:75, respectively) were dissolved in 1,1,1,3,3,3-hexafluoro-2-propanol at a concentration of 12%. The suspensions were agitated overnight at room temperature. The original TGF-β3 powder was reconstituted at 20 μg/ml in sterile 4 mM hydrochloric acid containing 1 mg/ml BSA. Coaxial electrospinning was performed as previously described [Citation15]. Briefly, PLCL–collagen and TGF-β3 solutions were delivered into the coaxial outer and inner needles, respectively, before electrospinning. The fabrication was performed under ambient temperatures ranging from 19 °C to 23 °C. The applied voltage and the electrospinning distance were set at 14 kV and 13 cm. The flow rate of the PLCL–collagen solution and TGF-β3 solution was set at 1.0 ml/h and 0.2 ml/h, respectively. The formed nanofibers were collected by a grounded rotating plate covered with aluminum foil to ensure the collected nanofibers were uniformly distributed. Then scaffolds were cross-linked with glutaraldehyde steam to avoid dissolution of the collagen component and then preserved at −20 °C to maintain the bioactivity of TGF-β3 for further research.
Basic characterizations of scaffolds
The surface morphology of the scaffolds was observed using a scanning electron microscope (SEM, Phenom Pro G2, Philips, the Netherlands). Briefly, samples were critical-point dried, sputter coated with gold and observed. To verify the core–shell structure, the nanofibers were observed by transmission electron microscopy (TEM). FITC-BSA was used as the stabilizer to evaluate the distribution of TGF-β3 in the core part of nanofibers by laser scanning confocal microscopy (LSCM).
The hydrophilic performance of different scaffolds was evaluated by a contact angle test, as described previously [Citation10]. The mechanical properties were evaluated by a tensile strength tester (3345, Instron, Norwood, MA) at room temperature and 65% humidity. The samples were tailored into 30 × 10 mm patches and fixed by clamps. The tensile speed was set at 10 mm/min, and then the stretch ratio and stress intensity were recorded.
The release behavior of TGF-β3 in vitro
The release curve of TGF-β3 in vitro was determined by Elisa. Briefly, 50 mg of the scaffolds were immersed in 2 ml PBS and placed on a shaker (150 rpm) at 37 °C. All solutions were collected from each sample every 2 days and replaced with an equal volume of fresh PBS. The concentration of TGF-β3 in the collected solutions was measured using the Elisa kit according to the manufacturer's description. Then, a release curve was graphed according to the cumulative amount of TGF-β3 released at different time points.
The degradation of scaffolds in vitro
The morphological changes in the scaffolds were observed at different time points, to evaluate the degradation process in vitro. In detail, samples were obtained from membranes after immersion in PBS for 1 month and 2 months on a shaker (150 rpm) at 37 °C. After vacuum freeze-drying, the surface morphology of samples was observed by SEM as described above.
Isolation, expansion and identification of BMSCs in vitro
BMSCs from New Zealand white rabbits were isolated as previously described [Citation16]. Briefly, fresh bone marrow was aspirated, centrifuged and resuspended by α-MEM (supplemented with 5% KOSR, 100 U/mL penicillin and 100 U/mL streptomycin). Primary culture was conducted at 37 °C in a humidified atmosphere with 5% CO2, and the culture medium was replaced every 2 days. Subculture at a density of 2 × 104 cells/cm2 was initiated when primary cells reached over 80% confluence. BMSCs of passage 2–3 were used for further experiments.
The multipotency of the obtained BMSCs was identified by flow cytometry. Briefly, after nonspecific blocking, cells were resuspended and incubated in a dark and cool (4 °C) environment for 30 min with the following primary antibodies: anti-rabbit CD29 FITC, CD34 APC, CD44 PerCP, CD45 FITC, CD73 FITC and CD105 APC, respectively. Finally, the expression levels of surface markers on BMSCs were analyzed by flow cytometry (BD FACSCanto, San Jose, CA).
Biocompatibility of scaffolds
To investigate the cytocompatibility, BMSCs were seeded on scaffolds. Then proliferation was evaluated by the MTT assay, and cell adhesion was observed under SEM. First, scaffolds were tailored into round shapes (15 mm in diameter), sterilized under ethanol steam for 3 h, and washed in PBS to eliminate ethanol before cell seeding. Then, the membranes were spread at the bottom of 24-well culture plates. BMSCs were seeded on the scaffolds at a density of 2 × 104 cells/well.
The MTT assay was performed 1, 4 and 7 days after cell seeding. Briefly, BMSCs were incubated with MTT for 4 h at 37 °C. Then DMSO was added to dissolve the formed blue-purple crystal. Finally, absorbance at 492 nm was measured using a microplate reader (Multiskan MK3, Thermo, Waltham, MA).
Cellular adhesion to the scaffolds was observed under SEM 7 days after seeding. Briefly, BMSCs seeded on membranes were fixed with 4% paraformaldehyde, dehydrated with an ethanol gradient, and dried under an air blower. Finally, the samples were observed by SEM as described above. Besides, to depict the morphological feature of seeded cells, the cellular outlines were delineated by phalloidine, a fluorochrome which could bind specifically with cytoskeleton. Briefly, BMSCs were fixed, permeabilized, stained with phalloidine, counterstained with DAPI and mounted.
Evaluation of chondrogenesis-inducing potential
After determining the preferable proportion of PLCL/collagen for tracheal patches, the chondrogenesis inducing potential of the scaffolds was evaluated. Similar core–shell scaffolds with identical constituent proportions but without TGF-β3 were fabricated as negative controls. The scaffolds were seeded with BMSCs by the established sandwich model, and nude mice were selected as bioreactors [Citation17]. Briefly, suspensions of BMSCs with a density of 1 × 108 cells/mL were prepared; scaffolds were sterilized and tailored into a round shape (10 mm in diameter). The first sheet was spread at the bottom of 12-well culture plates and smeared with 8 μl of the cell suspension. Then, the second sheet was stacked above the first one and smeared with an equal volume of suspension. The stacking and smearing were repeated until 6 sheets were stacked.
The scaffolds were assigned to 4 groups: 1) TGF-β3-encapsulating scaffolds incubated in growth medium for 1 week in vitro; 2) negative scaffolds incubated in growth medium for 1 week in vitro; 3) negative scaffolds incubated in differentiation medium containing TGF-β3 for 1 week in vitro; and 4) negative scaffolds incubated in differentiation medium containing TGF-β3 for 4 weeks in vitro. Then all stacks were implanted subcutaneously into nude mice and harvested 28 days later ().
To confirm the chondrogenic differentiation of BMSCs, histological staining and immunohistochemistry assays (IHC) were performed. Briefly, samples were fixed, embedded and sectioned at 5 μm. Then sections were stained with hematoxylin and eosin (HE), safranin O and toluidine blue to confirm the microstructure of the obtained tissues. The expression levels of collagen type II, a hyaline chondrocyte specific marker, were also assessed by IHC. Briefly, sections were stained, counterstained, dehydrated, hyalinized and mounted.
In addition, glycosaminoglycan (GAG) quantitative analysis was performed to determine the secretion function of the formed chondrocytes as previously described [Citation17]. Samples were digested with papain solution and mixed with 1,9-dimethylmethylene solution. Then absorbance value at 520 nm was measured. A standard curve was plotted using chondroitin sulfate as a reference, and fresh rabbit auricular cartilage tissues were used as control.
Construction of engineered tracheal patches
The scaffolds were sterilized and tailored into squares of size 1 cm ×2 cm. Then tracheal patches were also constructed by the aforementioned sandwich model. Briefly, the first membrane was spread on the special 3D printed grooved PLCL molds with a 6-mm inner diameter (), and smeared evenly with 40 μl of cell suspension. Then the stacking and smearing were repeated until 6 membranes were stacked. All stacks were incubated in growth medium for 7 days, and then implanted subcutaneously into nude mice. The patches were harvested 4 weeks after implantation, and some of them were subjected to histological and mechanical evaluations. The constant compressive strain rate was set at 0.5 mm/min, and a stress–strain curve was generated until 80% of maximal deformation was achieved. The native tracheas of rabbits were selected as the control.
Reconstruction of the trachea with tissue engineered patches
After anesthesia and skin preparation, a longitudinal incision was made in the anterior cervical region of rabbits. Then paratracheal tissues were dissociated carefully to fully expose the trachea. A square region (10 mm × 5 mm) of tracheal antetheca was excised; meanwhile, engineered tracheal patches described above were tailored to the same size as the defect. In test groups (n = 6), the patches were anastomosed with the residual trachea to restore the tracheal integrity, and then the incisions were closed. In sham groups (n = 6), tracheal antetheca of the same size was excised and then anastomosed with residual trachea ().
All rabbits received postoperative antibiotics, and bronchoscope examinations were performed at 1 and 2 months post-operation to determine the healing state of the operation area. The rabbits were sacrificed 2 months after operation, and patches with adjacent tracheal tissues were obtained for histological examination. The re-epithelization was also evaluated by SEM and immunofluorescence of cytokeratin 5/8, a specific marker of tracheal epithelium.
Statistical analysis
All enumeration data collected were expressed as means ± standard deviation (SD). Statistical analyses were performed by a one-way analysis of variance (ANOVA) followed by the Bonferroni test using SPSS 19.0. Statistical differences were considered statistically significant at p < .05.
Results
Basic characterization of the different scaffolds
The surface morphology of the fabricated scaffolds was observed by SEM. As shown in , all surfaces of membranes with different ingredient proportions were smooth and consistent, and the nanofibers were homogeneous. In addition, interconnected pores formed by the crosslinking of nanofibers, which could provide cell adhesion sites resembling native ECM, were also observed. There was no significant morphological difference among the scaffolds with different ingredient proportions.
Figure 1. Surface morphological observations and contact angle test of fabricated nanofilms with different constitute proportions. (A, representative images of SEM; B, representative images of TEM; C, representative images of LSCM, green color: FITC-BSA in TGF-β3 solutions in the core part of nanofibers; and D, water contact angle test). LSCM: laser scanning electron confocal microscope; SEM: scanning electron microscope; TEM: transmission electron microscope.
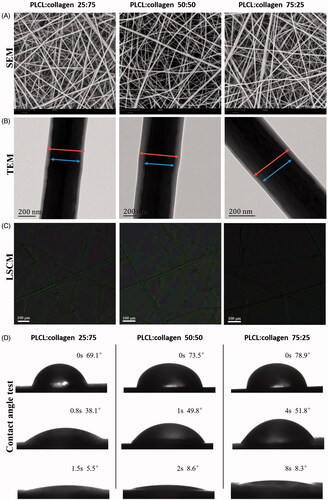
The core–shell structure of the nanofibers was confirmed by TEM and LSCM. As shown in ), the core–shell structure of different nanofibers was stable and smooth. No obvious defects in the shell part were observed, and the TGF-β3 solution was distributed homogeneously in the core part.
As the results of the water contact angle test revealed, droplets dropped on the scaffolds with a constituent ratio of PLCL:collagen 25:75 disappeared more quickly than on the scaffolds with other constituent ratios, indicating that scaffolds with higher collagen proportions possessed better hydrophilicity than scaffolds with lower collagen proportions ().
The mechanical properties of different scaffolds were evaluated comparatively and are presented in . It was seen that the tensile strength and elongation decreased with the increase of collagen composition. In particular, tensile strength decreased from 6.39 ± 0.27 MPa to 3.05 ± 0.54 MPa (p < .05) as the collagen proportion increased from 25% to 75%, while the elongation also dropped from 42.63 ± 0.52% to 22.62 ± 1.69% (p < .05), indicating that the tensile property decreased substantially with the increase of collagen in the composition.
Release behavior of TGF-β3 in vitro
As demonstrated by , the release behaviors of different scaffolds were all relatively stable, and no burst release was observed. The initial release was slightly higher and was followed by a relatively slow release, which might be caused by TGF-β3 appearing on the surface during electrospinning. The release times of different scaffolds were 52.80 ± 3.32 days (PLCL:collagen 75:25), 31.66 ± 2.58 days (PLCL:collagen 50:50) and 17.42 ± 2.23 days (PLCL:collagen 25:75) (p < .05).
Degradation of scaffolds in vitro
The morphology of the scaffolds with different degradation periods was evaluated by SEM and is shown in . Generally, the appearance of all scaffolds became blurred and rough with the extension of degradation period. Some of the nanofibers became slim and curly and even collapsed. More evident morphological alterations were observed in the scaffolds with PLCL:collagen 25:75 after 2 months of degradation in vitro, as most of the nanofibers in them were fractured. Overall, all scaffolds gradually degraded in vitro, but the scaffolds with PLCL/collagen proportion of 75:25 maintained better nanofibrous microstructures than the other scaffolds.
Isolation and identification of BMSCs
Before the proliferation study, the multipotency of BMSCs was identified by FCM. As presented in , the appearance of BMSCs under light microscope was typical, with a spindle-like morphology. In addition, the FCM analysis indicated that the BMSCs were positive for CD29, CD44, CD73 and CD105, whereas negative for CD34 and CD45 ().
Figure 3. Isolation and identification of BMSCs, and cytocompatibility of different nanofilms (A, representative images of BMSCs under light microscope; B, flow cytometry of specific multipotential stem cells; C, MTT assay of different nanofilms; and D, representative images of SEM and fluorescent staining after BMSCs were seeded on different nanofilms.). * indicates statistical difference compared with culture plate. Red: cytoskeleton, Blue: nucleus.
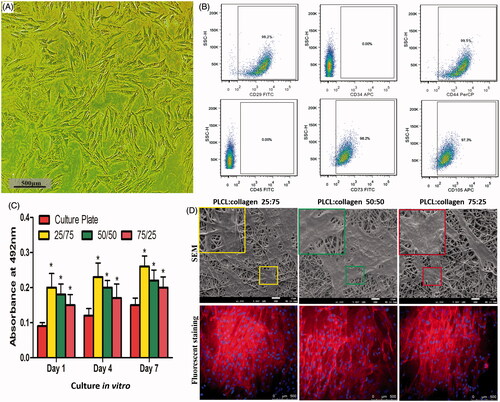
Biocompatibility of scaffolds
As shown in , all fabricated scaffolds provided sufficient binding sites for seed cells so that BMSCs could adhere, spread and proliferate on the scaffolds. After 7 days of cell culture, the BMSCs bonded with scaffolds closely, and the scaffolds were partially coated with proliferated BMSCs. The seed cells proliferated much faster on the different scaffolds than on the culture plates, exhibiting excellent biocompatibility. However, no significant differences in the MTT assay were found among different scaffolds, indicating that their biocompatibilities were almost identical.
Chondrogenesis inducing potential
After lateral comparison, the biocompatibility of the scaffolds with different compositional ratios was all satisfactory. However, scaffolds with the PLCL/collagen ratio of 75:25 were preferable for tissue engineered tracheal patches, due to their superior mechanical properties, ideal release behavior and proper degradation speed. Thus, they were selected for further research.
The histological evaluation following maturation in vivo for 28 days is presented in . As shown, typical mature hyaline chondrocytes and cartilage lacunas were observed between the scaffolds in group 1. Toluidine blue and safranin O staining results indicated that abundant ECM of hyaline cartilage accumulated and distributed homogenously around the formed chondrocytes after maturation. Besides, IHC staining results indicated that positive expression of collagen type II, further identified the chondrogenesis of BMSCs. In addition, there was no significant difference of GAG content between group 1 and rabbit auricular cartilage tissues (p > .05). These results above suggest that the chondrogenesis of BMSCs was successfully induced by the fabricated TGF-β3 encapsulating scaffolds, and the formed chondrocytes compared favorably with native, mature chondrocytes in auricular cartilage tissues.
Figure 4. Chondrogenesis inducing potential evaluation (A, Sandwich model nanofilms stacking and subcutaneous implantation in nude mice; B, list of different groups, and GAG contents of different groups after subcutaneous implantation; and C, representative images of HE, Toluidine blue and Safranin O stainings, and IHC of type II collagen). Scale bars: 200 μm. * indicates statistical difference between groups.
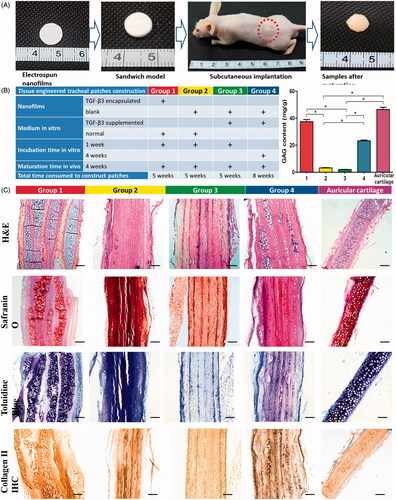
Additionally, a few mature hyaline chondrocytes and cartilage lacunas were also observed in group 4. But the number of formed chondrocytes was obviously smaller that of group 1, and the GAG content and collagen type II expression were also reduced.
In contrast, almost no typical hyaline chondrocytes or cartilage lacunas were observed in groups 2 and 3, and there was almost no GAG accumulation or collagen type II expression in these groups.
Histological and mechanical properties of tracheal patches
After subcutaneous incubation in nude mice, tracheal patches were harvested and evaluated by histological and mechanical examinations. As revealed by , most seed cells differentiated into mature chondrocytes as expected. The thickness of the tracheal patches and native rabbit tracheas were almost comparative (p > .05). The Young’s modulus of the constructed tracheal patches was slightly lower than that of native rabbit tracheas, but the difference was not statistically significant (p > .05).
Figure 5. A, Construction of engineered tracheal patches (I, construction of engineered patches in vitro; II, subcutaneous implantation in nude mice; III, gross observation and HE staining (Scale bars: 500 μm) of tracheal patches after maturation in vivo; IV, thickness and Young's modulus of tracheal patches and native tracheas.). B, Tracheal reconstruction with tissue engineered patches (I, artificially caused tracheal defects in rabbit model; II, tailored engineered tracheal patches; III, tracheal antetheca was excised and then anastomosed with residual trachea in sham group; and IV, tailored patch was anastomosed with residual trachea in test group.). C, Bronchoscope examination 1 month and 2 months after operation. D, Histological examinations 2 months post operation (I and II, representative images of HE staining (Scale bars: 500 μm); III and IV, representative immunofluorescent images of tracheal epithelial cells (Green: cytokeratin 5/8, Blue: nucleus, Scale bars: 250 μm)). E, SEM observation of the luminal surface of the defects 2 months after operation.
Dotted circular rings indicate tracheal reconstruction operation areas.
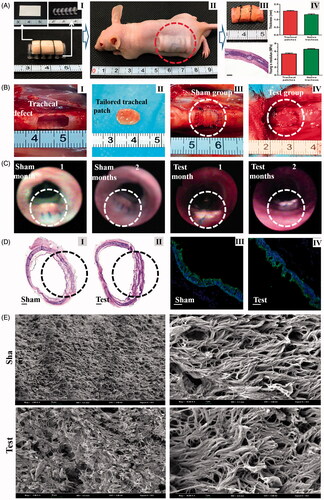
Restoration of tracheal defects with tissue engineered patches
Tracheal defects were successfully made and restored with engineered tracheal patches as expected (). After the restoring operation, neither air leakage nor collapse around engineered patches were observed. All rabbits in 2 groups survived well, and none rabbits showed any sign of stridor or dyspnea during recovery periods. The bronchoscope examination indicated that anastomotic wounds in the test and sham groups were almost completely healed 1 month after restoration (). In addition, no obvious inflammatory exudation, formation of granulation tissues, anastomotic leakage or stenosis were found in the operation areas 4 weeks post operation. The tracheal segment including the surgical area was obtained 2 months after operation, and then subjected to histological examination and SEM. As revealed in , the formed chondrocytes survived well in the tracheal patches, and the patches integrated tightly with the residual native tracheal tissues. In addition, SEM observation indicated that the luminal surface of trachea in the sham group were covered by clusters of typical hair-like ciliated cells, and similar cilia were also observed on the luminal surface of the engineered patch 2 months after operation. In addition, immunofluorescence indicated the cells in both groups were positive for cytokeratin 5/8, confirming the observed re-epithelialization ().
Discussion
In the present research, TGF-β3-encapsulating core–shell scaffolds with different proportion of PLCL/collagen were fabricated by coaxial electrospinning, and their basic characterizations were compared. The results indicated that scaffolds with a proportion of PLCL:collagen 75:25 were preferable for tissue engineered tracheal patches, due to their appropriate degradation speed and excellent biological and mechanical properties. After TGF-β3-encapsulating scaffolds were seeded with BMSCs by the sandwich model in vitro, and then implanted subcutaneously into nude mice for maturation in vivo, mature hyaline cartilage tissue originating from chondrogenic differentiation of BMSCs was found among the scaffolds. At last, engineered tracheal patches were successfully constructed and utilized to restore tracheal defects in rabbit models. Tracheal integrality was well maintained and re-epithelization also achieved as expected, 2 months post-operation.
Tissue engineering strategies based on electrospun scaffolds and MSCs provide restorative tracheal patches for patients with overlong tracheal stenosis. Tracheal patches can be constructed by the established sandwich model [Citation17]. Briefly, MSCs cells are seeded onto scaffolds, incubated in vitro with chondrogenic differentiation medium, and matured in vivo. However, the chondrogenic incubation in vitro usually takes considerable time, and the efficiency is unsatisfactory. Enlightened on the advantages of core–shell nanofibers, which can be used for the controlled delivery of drugs and biological factors, we envisioned fabricating novel scaffolds encapsulating chondrogenic induction factors to optimize the engineering of tracheal patches [Citation13]. In our pilot experiments, core–shell structured nanofilms encapsulating TGF-β3 were fabricated, and bioactive TGF-β3 was stably released to guide the chondrogenesis of seeded stem cells in vitro [Citation14].
To verify whether these scaffolds could be used for tracheal patches, this study was conducted. First, characterizations of scaffolds with different constituent ratios were comparatively evaluated to determine the preferable proportion of PLCL and collagen. It has been proven, by our previous investigations, that electrospinning with combinations of PLCL and collagen can fabricate nanofibers with better cells-scaffold interactions and improved mechanical properties [Citation18]. But the specific ratios for tracheal patches remain undetermined. The mechanical strength dramatically decreased with the increase of collagen proportion. However, mechanical properties are crucial for native trachea, since their main function is maintaining an open airway during breathing movements to avoid collapse caused by the inter-thoracic pressure [Citation19]. In addition, scaffolds with higher collagen proportions degrade much faster than those with lower collagen proportions. The release behavior of encapsulated bioagents in the core of the nanofibers is mainly affected by the degradation of the shell [Citation13,Citation20]. Our results regarding TGF-β3 release behavior were also consistent with the above conclusion. The scaffolds with PLCL:collagen 75:25 could stably and sustainably release bioactive TGF-β3 for more than 40 days, ensuring sufficient time for the chondrogenic differentiation of MSCs. Taken collectively, scaffolds with PLCL/collagen proportion of 75:25 were chosen for engineering tracheal patches.
The chondrogenesis inducing potential of TGF-β3-encapsulating scaffolds was examined. After incubation in vitro, the sandwiches were transplanted into bioreactors. The bioreactors, nude mice with cellular immunodeficiency, provide an ideal microenvironment containing the essential nutrient substances for maturation of engineered tissues/organs. During maturation, vascularization occurs and seed cells exert some cellular functions, such as the secretion of cytokines and synthesis of ECM [Citation21]. The efficiency of chondrogenic differentiation in vivo induced by the TGF-β3-encapsulating scaffolds was evidently superior to induction in vitro. A possible reason might be that after embedding, seed cells on the TGF-β3-encapsulating scaffolds proliferated rapidly with sufficient nutrients, while the secreted ECM provided support for three-dimensional cell growth. Then, plentiful MSCs differentiated into chondrocytes, induced by the sustained release of TGF-β3. However, when incubated in vitro, BMSCs were only able to grow and proliferate as a monolayer on the surface of the scaffolds; in addition, seed cells between the nanofilms were not fully exposed to the inducing factors in the medium, restricting the number of formed chondrocytes. Furthermore, construction of tracheal patches with TGF-β3-encapsulating nanofilms saves considerable time compared with conventional methods, which might be of vital importance for patients who require timely operation.
The feasibility of constructing tissue engineered patches for tracheal restoration was evaluated in rabbit models. In previously reported investigations, other tracheal patches were also constructed based on electrospun scaffolds [Citation6]. However, the patches were flat and planar, without steradian, so that they had to be implanted together with airway stents. In our investigation, tracheal patches were constructed on 3D-printed molds with an inner diameter similar to that of native rabbit tracheas. The molds were removed after maturation, and the engineered patches still possessed certain steradian configurations. Rabbits that received the operation survived well for 2 months, and bronchoscope examinations suggested that the luminal healing state was satisfactory, with no tracheal collapse or stenosis and a potent growth potential observed for the engineered patches. It has been reported that re-epithelialization of transplanted tracheal patches can be achieved by the migration of surrounding native epithelial cells [Citation22]. Our SEM observation also found that the luminal surface of the engineered patch was covered by airway epithelial tissues. Even though the partial epithelium was slightly sparser than native epithelium, the morphological characteristics of the cilia were similar to those observed on native tracheas.
Although the results are indeed encouraging, some limitations still exist. It is the nude mice, rather than tracheal patch recipients, that are chosen as bioreactor for maturation in vivo. Besides, our engineered patches are made of consecutive cartilaginous tissues instead of cartilaginous rings and connective tissues. Longer follow-up and longer segmental reconstruction with these tissue-engineered tracheal patches haven’t been investigated. Additional efforts in this area still need to be made in the future.
Conclusion
The TGF-β3-encapsulating core–shell scaffolds with a proportion of PLCL:collagen 75:25 fabricated by coaxial electrospinning were preferable for the engineering of tracheal patches. The tissue engineered patches based on TGF-β3-encapsulating scaffolds and BMSCs can be used for tracheal restoration.
Disclosure statement
No potential conflict of interest was reported by all authors.
Additional information
Funding
References
- Rubikas R, Matukaityte I, Jelisiejevas JJ, et al. Surgical treatment of non-malignant laryngotracheal stenosis. Eur Arch Otorhinolaryngol. 2014;271:2481–2487.
- Wang S, Zhang H, Zhu L, et al. Surgical management of congenital tracheal stenosis associated with tracheal bronchus and congenital heart disease. Eur J Cardiothorac Surg. 2016;49:1201–1206.
- Chen QK, Jiang GN, Ding JA, et al. Reconstruction of the lower trachea using a pedicled autologous bronchial flap. Ann Thorac Surg. 2010;89:e29–e30.
- Udelsman BV, Eaton J, Muniappan A, et al. Repair of large airway defects with bioprosthetic materials. J Thorac Cardiovasc Surg. 2016;152:1388–1397.
- Zang M, Chen K, Yu P. Reconstruction of large tracheal defects in a canine model: lessons learned. J Reconstr Microsurg. 2010;26:391–399.
- Ott LM, Zabel TA, Walker NK, et al. Mechanical evaluation of gradient electrospun scaffolds with 3D printed ring reinforcements for tracheal defect repair. Biomed Mater. 2016;11:025020.
- Ko E, Lee JS, Kim H, et al. Electrospun silk fibroin nanofibrous scaffolds with two-stage hydroxyapatite functionalization for enhancing the osteogenic differentiation of human adipose-derived mesenchymal stem cells. ACS Appl Mater Interfaces. 2017. doi: https://doi.org/10.1021/acsami.7b03328. [Epub ahead of print]
- Sharif S, Ai J, Azami M, et al. Collagen-coated nano-electrospun PCL seeded with human endometrial stem cells for skin tissue engineering applications. J Biomed Mater Res Part B Appl Biomater. 2017. doi: https://doi.org/10.1002/jbm.b.33966. [Epub ahead of print]
- He X, Fu W, Feng B, et al. Electrospun collagen-poly(L-lactic acid-co-ε-caprolactone) membranes for cartilage tissue engineering. Regen Med. 2013;8:425–436.
- He X, Fu W, Feng B, et al. Electrospun collagen/poly(L-lactic acid-co-epsilon-caprolactone) hybrid nanofibrous membranes combining with sandwich construction model for cartilage tissue engineering. J Nanosci Nanotechnol. 2013;13:3818–3825.
- Lee PT, Li WJ. Chondrogenesis of embryonic stem cell-derived mesenchymal stem cells induced by TGFbeta1 and BMP7 through increased TGFbeta receptor expression and endogenous TGFbeta1 production. J Cell Biochem. 2017;118:172–181.
- Chen J, Wang Y, Chen C, et al. Exogenous heparan sulfate enhances the TGF-beta3-induced chondrogenesis in human mesenchymal stem cells by activating TGF-beta/smad signaling. Stem Cells Int. 2016;2016:1520136.
- Romano L, Camposeo A, Manco R, et al. Core-shell electrospun fibers encapsulating chromophores or luminescent proteins for microscopically controlled molecular release. Mol Pharm. 2016;13:729–736.
- Wang J, Sun B, Tian L, et al. Evaluation of the potential of rhTGF- beta3 encapsulated P(LLA-CL)/collagen nanofibers for tracheal cartilage regeneration using mesenchymal stems cells derived from Wharton's jelly of human umbilical cord. Mater Sci Eng C Mater Biol Appl. 2017;70(Pt 1):637–645.
- Yin L, Yang S, He M, et al. Physicochemical and biological characteristics of BMP-2/IGF-1-loaded three-dimensional coaxial electrospun fibrous membranes for bone defect repair. J Mater Sci: Mater Med. 2017;28:94.
- Zhang W, Zhang F, Shi H, et al. Comparisons of rabbit bone marrow mesenchymal stem cell isolation and culture methods in vitro. PloS One. 2014;9:e88794
- He X, Feng B, Huang C, et al. Electrospun gelatin/polycaprolactone nanofibrous membranes combined with a coculture of bone marrow stromal cells and chondrocytes for cartilage engineering. Int J Nanomed. 2015;10:2089–2099.
- Fu W, Liu Z, Feng B, et al. Electrospun gelatin/PCL and collagen/PLCL scaffolds for vascular tissue engineering. Int J Nanomed. 2014;9:2335–2344.
- Tsukada H, O'Donnell CR, Garland R, et al. A novel animal model for hyperdynamic airway collapse. Chest. 2010;138:1322–1326.
- Hsu YH, Lin CT, Yu YH, et al. Dual delivery of active antibactericidal agents and bone morphogenetic protein at sustainable high concentrations using biodegradable sheath-core-structured drug-eluting nanofibers. Int J Nanomed. 2016;11:3927–3937.
- Xue J, Feng B, Zheng R, et al. Engineering ear-shaped cartilage using electrospun fibrous membranes of gelatin/polycaprolactone. Biomaterials. 2013;34:2624–2631.
- Nakaegawa Y, Nakamura R, Tada Y, et al. Effect of structural differences in collagen sponge scaffolds on tracheal epithelium regeneration. Ann Otol Rhinol Laryngol. 2016;125:115–122.