Abstract
A promising strategy to accelerate bone generation is to deliver a combination of certain growth factors to the integration site via a controlled spatial and temporal delivery mode. Here, a composite hydrogel incorporating poly(lactide-co-glycolide) (PLGA) microspheres was accordingly prepared to load and deliver the osteogenic rhBMP-2 and angiogenic rhVEGF165 in the required manner. In addition, 2-N,6-O-sulphated chitosan (26SCS), which is a synergetic factor of growth factors, was incorporated in the composite hydrogel as well. The system showed a similar release behaviour of the two growth factors regardless of 26SCS inclusion. RhBMP-2 loaded in PLGA microspheres showed a sustained release over a period of 2 weeks, whereas rhVEGF165 loaded in hydrogel eluted almost completely from the hydrogel over the first 16 days. Both growth factors retained their efficacy, as quantified with relevant in vitro assays. Moreover, an enhanced cell response was achieved upon the delivery of dual growth factors, compared to that obtained with a single factor. Furthermore, in the presence of 26SCS, the system revealed significantly upregulated alkaline phosphatase activity, human umbilical vein endothelial cell proliferation, sprouting, nitric oxide secretion, and angiogenic gene expression. This study highlighted that the composite hydrogel incorporated with 26SCS appears to constitute a promising approach to deliver multiple growth factors. From our findings, we could also conclude that rhBMP-2 can promote angiogenesis and that the mechanism is worthy of further study in subsequent research.
Introduction
The repair of large bone defects and non-unions resulting from trauma, resection or pathology remains a major challenge for orthopaedic, reconstructive and maxillofacial surgeons [Citation1,Citation2]. Apart from providing osteoinductive activity, bone is a highly vascularized tissue reliant on the close spatial and temporal connection between blood vessels and bone cells to maintain skeletal integrity. Angiogenesis thus plays a pivotal role in skeletal development and bone fracture repair [Citation3]. Specifically, angiogenesis induces a functional vascular network within the defect site that is crucial for sufficient nutrient supply, transport of macromolecules, invasion of cells and maintenance of the appropriate metabolic microenvironment during bone repair [Citation4]. Therefore, developing strategies that could promote both angiogenesis and osteogenesis during the bone regeneration process is promising yet challenging.
Growth factors (GFs) are often used to transmit signals and modulate cellular activities during the process of bone repair [Citation5–7]. Bone morphology protein-2 (BMP-2), belonging to the transforming growth factor-β (TGF-β) superfamily, has been recognized as one of the most effective osteoinductive GFs. BMP-2 stimulates both osteoblast proliferation and differentiation [Citation8], recruits undifferentiated mesenchymal cells from peripheral tissues, and facilitates precursor cell differentiation into bone forming cells. Furthermore, recombinant human BMP-2 (InfuseTM) has been approved for the treatment of spinal fusion, acute tibial fractures and non-union bone defects. In addition, vascular endothelial growth factor (VEGF), a potent growth factor for driving endothelial behaviour and enhancing osteogenesis during early fracture repair and endochondral and intramembranous ossification [Citation3,Citation9,Citation10], has been shown to be a key regulator in bone repair as well. Accordingly, previous studies have focussed on the design of combinational controlled delivery systems for both BMP-2 and VEGF. In a representative in vivo study, Richard et al. demonstrated that co-delivery of BMP-2 and VEGF could enhance the repair and regeneration of critical-sized bone defects [Citation11]. Co-culture experiments revealed the existence of reciprocal communications between osteoblast-like cells and endothelial cells, which played a crucial role during angiogenesis and osteogenesis [Citation12–14]. Moreover, BMP-2 has been shown to upregulate the production of VEGF by inducing differentiation of preosteoblast-like cells [Citation15]. Thus, it may be deduced that co-delivery of BMP-2 and VEGF may facilitate the recruitment of cells to the implanted site to achieve consequent angiogenesis and bone regeneration. However, the challenge in using GFs is their inherent short biological half-life in vivo. Both BMP-2 and VEGF are apt to suffer enzymolysis or undergo physical and chemical denaturation. Previous pharmacokinetic studies indicated that the half-life of BMP-2 in primates is only 7 min; whereas that of intravenously injected VEGF is less than one hour [Citation16]. Hence, a controlled release system to prolong the duration time of GFs is required owing to their short biological half-lives, and that they do not always exhibit the expected efficacy in bone regeneration under physiological conditions in vivo. Furthermore, using higher dosage of GFs as compensation may also lead to contingent risk and undesirable side effects [Citation13]. Consequently, improving the bioactivity of GFs becomes increasingly necessary. One option is heparin/heparin sulphate, which is evidenced as having potential to interact with BMP-2 and VEGF165, resulting in enhanced bioactivity of the GFs during bone regeneration [Citation17–19]. Previous studies by our group demonstrated that 2-N,6-O-sulphated chitosan (26SCS), a heparin-like sulphonated polysaccharide, albeit with higher degrees of sulphation than any naturally sulphated polysaccharide, could enhance the BMP-2 activity in a dose-dependent profile both in vitro and in vivo [Citation20,Citation21]. Notably, the 26SCS-containing scaffold not only endowed an efficient loading and sustained release feature of VEGF, but also displayed an incremental vascularization capacity of endothelial cells [Citation22]. Although it is not explicit whether sulphated chitosan possesses a synergistic effect of proangiogenesis, it is reasonable to expect that incorporation of 26SCS might promote both BMP-2 and VEGF synchronously.
In the present study, a convenient and efficient strategy for a dual growth factor-loading system has been explored. Poly(lactide-co-glycolide) (PLGA) microspheres (PMs) were embedded into photopolymerizable gelatine (Gel) to generate complex hydrogels (Gel/PMs) for dual growth factor loading. Besides, 26SCS was doped into Gel to assist active release of the growth factor (GF). As expected, the hybrid delivery system could afford disparate spatio-temporal releasing profiles: one kind of GF captured in the hydrogel matrix would diffuse rapidly owing to the speedy swelling behaviour and degradation rate of the hydrogel; whereas the other kind of GF encapsulated in polymeric microspheres would exhibit a subdued releasing pattern owing to the lower wettability and degradation rate of the polyester encapsulation. Herein, an initial release of rhVEGF165 from Gel followed by the sustained release of rhBMP-2 from PMs was designed to better enhance bone regeneration. Moreover, we hypothesized that 26SCS discharged from the hydrogel would have positive effects on both rhBMP-2 and rhVEGF165. The physical properties of Gel/PMs and in vitro release kinetics of rhBMP-2 and rhVEGF165 were examined. Specifically, we focussed on investigating the reciprocal effects of combinational rhBMP-2, rhVEGF165 and 26SCS equipped in Gel/PMs. Alkaline phosphatase (ALP) activity was determined to assess the osteogenic differentiation of rhBMP-2 whereas behaviours of human umbilical vein endothelial cells (HUVECs) such as proliferation, sprouting, nitric oxide (NO) production and relative angiogenic gene expression were inspected to estimate the proangiogenic capacity of rhVEGF165. We hypothesized that the composite hydrogel incorporating rhBMP-2, rhVEGF165 and 26SCS not only achieved the spatial release of dual GFs but also endowed a more desirable cellular response owing to the synergistic effects among rhBMP-2, rhVEGF165, and 26SCS.
Experimental section
Materials
rhBMP-2 was a gift from Rebone Biomaterials Co. Ltd. (Shanghai, China). RhVEGF165 was obtained from Peprotech Inc. PLGA (LA:GA =50:50, Mw = 2 × 104 and 1 × 105) was purchased from Jinan Daigang Biomaterials Co., Ltd. Porcine type A gelatine (approximately 300 g Bloom, G1890), the metal-complex tris (2,20-bipyridyl) dichlororuthenium (II) hexahydrate ([RuII(bpy)3]2+), sodium persulphate (SPS), Human BMP-2 Elisa kit, Human VEGF165 Elisa kit and fluorescein isothiocyanate-phalloidin (FITC-Phalloidin) were purchased from Sigma-Aldrich (St. Louis, MO). 26SCS was prepared in our previous study [Citation20]. All cell-culture related reagents were available from Gibco (Grand Island, NY).
Preparation of composite hydrogel gel/PMs
According to the formulation listed in , different composite hydrogels with different concentrations of PMs or gelatine were developed. Briefly, a certain amount of PLGA (Mw =2 × 104) was dissolved in dichloromethane (CH2Cl2) forming 10% PLGA/CH2Cl2 solution while different concentration of gelatine/phosphate-buffered saline (PBS) solution was prepared. Then, PLGA/CH2Cl2 solution was slowly added into the gelatine/PBS solution to generate a PMs-containing complex and stirred at 1200 rpm in a 37 °C water bath for 60 min. Next, the stocking solution of [RuII(bpy)3]2+ and SPS in PBS were added into the PMs-containing complex to the concentration of 1 mM and 20 mM, respectively. After being dispensed into a Teflon mold, the complex precursors were irradiated for 60 s at room temperature with an LED dental curing lamp (460 nm, 850–1000 mW/cm2 at source, Woodpecker, LED.B, Henan, China) from a distance of 150 mm [Citation23,Citation24]. The composite hydrogel with 0 wt%, 5 wt%, 10 wt%, 15 wt% of PMs (g/g, PLGA/gelatine) was named as Gel, Gel/PMs-5, Gel/PMs-10 and Gel/PMs-15, respectively. In order to optimize the gelatine concentration, 50 mg/mL (low concentration) and 300 mg/mL (high concentration) of gelatine was used to prepare the 15 wt% of PMs-containing composite hydrogel and labelled as L-Gel/PMs-15 and H-Gel/PMs-15, respectively. To obtain the 26SCS-doped composite hydrogel (26SCS/Gel/PMs-15), 2 μg/mL 26SCS was added into PBS during gelatin/PBS solution preparation. We note that for further cell culture, sterile filtered components such as 26SCS or gamma-sterilized gelatine were used to fabricate the composite systems under sterile conditions.
Table 1. The formulation of different composite hydrogel.
Physical characterization
The macroscopic inspection of Gel/PMs was obtained using a digital camera (Nikon, Tokyo, Japan) and its sectional profiles were also investigated by scanning electron microscopy (SEM, S-3400, Hitachi, Tokyo, Japan). The samples were freeze-fractured in liquid nitrogen and then gold sputter-coated. PMs in the Gel/PMs complex system were collected by centrifugation and washed repeatedly after they were dispersed evenly in gelatine/PBS solution and before irradiation. The obtained PMs were morphologically examined by SEM. The uniformly dispersed PMs were sputter-coated with gold prior to observation. Particle size measurement of the collected PMs was carried out using a laser particle size analyzer (LS230, Beckman Coulter, Brea, CA). The sample was analyzed at 37 °C by performing three readings.
The elastic moduli of Gel, Gel/PMs-5, Gel/PMs-10 and Gel/PMs-15 containing 0, 5, 10 and 15 wt% of PMs, respectively, was determined by performing constant strain-rate compression tests using a Rheometrics Solid Analyzer (RSAII, Rheometrics Inc., Piscataway, NJ) equipped with a 10 N load cell. In brief, samples were tested in air immediately after removal from the mold. The unconfined compression tests were carried out on the sample disks (10 mm d × 5 mm h) at room temperature using a constant strain rate of 2 mm/min. The compressive modulus of the hydrogel was determined by calculating the slope of a linear region of the stress-strain curve limited to the linear first 10% strain of the plots. At least five replicates were carried out for each group and the results were expressed as mean ± standard deviation (mean ± SD).
Swelling test was performed on sample disks (10 mm d × 5 mm h) of Gel, Gel/PMs-5, Gel/PMs-10 and Gel/PMs-15 containing 0, 5, 10 and 15 wt% of PMs, respectively. Samples, in quintuplicate, were weighed immediately after crosslinking (W0), and then incubated in 10 ml PBS at 37 °C. At various time points, the discs were blotted using tissue paper to remove excess buffer, and then weighed (Wt). The degree of swelling (denoted as %S) was calculated from the formula: %S = [(Wt−W0)/W0] × 100. The results were expressed as mean ± SD.
The degradation rate of PMs released from the composite hydrogel was also investigated. PLGA with different molecular weight (2 × 104 and 1 × 105) was respectively used to develop the composite hydrogel according to the formulation of Gel/PMs-15 in . Then, the prepared composite hydrogel was incubated in 5 ml PBS at 37 °C with continuous shaking. At various time points, the free PMs released from the composite hydrogel were collected by centrifugation and examined on a Waters 515 gel permeation chromatograph (GPC) equipped with three Waters Styragel columns (HT2 + HT3 + HT4) at 35 °C, THF as the eluent (1.0 ml/min), polystyrene as the calibration standard, and Waters Millennium 32 as data processing software.
Cell culture
Mouse cranioaural osteoblastic progenitor MC3T3-E1 cells and human umbilical vein endothelial cells (HUVECs) were selected as the representative cell lines in this study to investigate the biocompatibility and efficacy of GFs released from Gel/PMs-15. MC3T3-E1 cells and HUVECs were grown in Dulbecco’s modified Eagle’s medium (DMEM), supplemented with 10% foetal bovine serum (FBS), penicillin 100 U/mL, streptomycin 100 μg/mL and cultured at 37 °C and 5% CO2 in a humidified incubator. Culture medium was replenished every 2–3 days and cells were subcultured when near 80% confluence with the use of 0.05% trypsin-ethylenediaminetetraacetic acid. Bone marrow mesenchymal stem cells (BMSCs) were used for the study of evaluating the in vitro osteogenesis induced by the released GFs from Gel/PMs-15 with different release profiles. Primary BMSCs were harvested from Sprague–Dawley rats (Shanghai SLAC Experimental Animal Center, Shanghai, People’s Republic of China) according to the previously described [Citation25]. All the procedures were approved by Animal Care and Use Committee of the Ninth People’s Hospital, which is affiliated with Shanghai Jiao Tong University School of Medicine. Briefly, bone marrow was flushed out with DMEM supplemented with 1% penicillin/streptomycin. After blood cells were removed by centrifugation, the collection was mixed with complete DMEM supplemented with 10% FBS and plated into a tissue culture flask. When large colonies formed and became confluent, the cells were trypsinized and passaged. BMSCs acquired from Passages two to three were used for our experiments.
Biocompatibility
Cell viability
The extracts of Gel and Gel/PMs-15 samples were prepared in advance under sterile conditions. Briefly, the Gel and Gel/PMs-15 samples were fabricated according to the formulations in . Samples were immersed in 2 ml culture medium at 37 °C for 1, 3, 7 and 14 d, respectively. At predetermined time points, the extracts were collected and stocked at −80 °C for further experiments. All samples were tested in triplicate.
The obtained extracts were examined by MTS assay to evaluate the cell viability. Briefly, after MC3T3-E1 cells at a density of 5000 cells/well were evenly seeded and attached onto a 96-well culture plate, 200 μL extract was added to each well and incubated for 48 h. Standard culture medium was used as a positive control. Then, the medium was replaced with fresh medium containing the tetrazolium dye MTS according to the manufacturer’s procedure. After incubating at 37 °C for 1.5 h, solution absorbance was determined at 490 nm using a microplate reader (SPECTR Amax 384, Molecular Devices, Sunnyvale, CA). Each sample was assayed in triplicate and the results were expressed in percentage of control (scored as 100% viability) as mean ± SD.
Cell adhesion
MC3T3-E1 cells were seeded at a density of 1 × 104 cells/cm2 onto 200 μL Gel/PMs-15 in a glass bottom cell culture dish (glass diameter: 10 mm). After 24 h incubation, media were removed and the cells were fixed with 1% glutaraldehyde and washed with PBS. Morphology of the adherent cells was explored using FITC-labelled phalloidin staining for cytoskeletal F-actin fibres and DAPI for nuclei.
In vitro GFs loading and release kinetics
During preparation of the composite hydrogel, GF such as rhBMP-2 or rhVEGF165 could be loaded into either PMs or gelatine hydrogel matrix to realize different GF release profiles. Meanwhile, molecular weight of PLGA closely affects degradation rate of PMs which is critical to the release of the involved GF. Here, we chose Gel/PMs-15 in as the GF delivery system. According to , we developed four types of GF release profiles based on the formulation of Gel/PMs-15 by modulating the molecular weight of PLGA (Group 1), GF loading position (Group 2 and 3) and 26SCS doping (Group 4). In brief, a certain amount of GF1 (1.5 μg rhBMP-2 or 15 ng rhVEGF165) was added into 150 μL PLGA/CH2Cl2 solution (10%, 15 mg PLGA, w/v) and mixed by ultrasonic. Meanwhile, GF2 (10 ng rhVEGF165 or 100 ng rhBMP-2) was loaded into 1 ml gelatine/PBS solution (100 mg/mL). Then, the GF1 loading complex was dropped into the GF2 loading gelatine/PBS solution followed by irradiation, thus forming a dual GFs loading composite hydrogel. For investigation of 26SCS doping effect on GF release (Group 4 in ), 2 μg/mL 26SCS was additionally added into gelatine/PBS solution (100 mg/mL) as well. The subsequent steps were similar to the preparation of Gel/PMs-15.
Table 2. The details of the composite hydrogel gel/PMs-15 with different GF release profiles.
The release kinetics of dual GFs from the above prepared system (Group 1–4) were inspected over a period of 30 days. Samples were placed in test vials and immersed in 3 ml PBS (pH =7.4), at 37 °C with constant agitation at 15 rpm. The buffer was collected (300 μL) at scheduled incubation times, lyophilized, and stored at −80 °C. An equal volume of fresh PBS was then replenished to the vial. At the end of the experiment, all collected samples were thawed and quantitatively analyzed using a Human BMP-2 ELISA Kit and Human VEGF165 ELISA kit. Triplicate parallel analyses were conducted for each time point with mean ± SD values reported. Cumulative release is expressed as a percentage of total loaded protein.
In vitro osteogenesis induced by the composite hydrogel containing GFs
The capability of the composite hydrogels containing GFs (as shown in ), which could provide different release profiles of rhBMP-2 and rhVEGF165, to induce osteogenesis in vitro were measured by determining their ability to induce alkaline phosphatase (ALP) enzymatic activity and calcium deposition in BMSCs. The composite hydrogel Gel/PMs-15 with no growth factor loading labelled as “No loading” was used as a control group. Briefly, BMSCs were seeded into 6-well culture plate at a density of 5 × 105 cells/well, followed by co-culture with a transwell system (Corning, New York, NY) loaded with the sample of each group. Each well contained 3 ml of culture medium in total. Half of the culture medium was replaced every 3 days. On Day 7, each plate was rinsed three times with PBS and fixed with 4% paraformaldehyde for 15 min. After being washed twice with distilled water, the cells were subjected to ALP staining (Beyotime, Shanghai, People’s Republic of China) according to the manufacture’s instruction. Stained cells were examined under a microscope (Eclipse Ti, Nikon). An ALP activity quantitative assay was also performed at the same time according to standard procedures [Citation26]. The relative ALP activity of each group was assessed as an OD value at 405 nm per milligram of total protein and then normalized to the fold changes by using the “No loading” group as the reference value (set to 1) and expressed as the mean ± SD. In addition, on Days 21, fixed cells were stained with 1% Alizarin red (Sigma-Aldrich, St. Louis, MO) for 10 min. The cells were then rinsed with water and viewed with an inverted light microscope (Eclipse Ti, Nikon). For the quantification analysis, the stained samples were desorbed using 10% cetylpyridinium chloride (Sigma, St. Louis, MO). Absorbance values at 590 nm were recorded. Intracellular total protein content was determined by use of the microBCA protein assay kit (Thermo, Waltham, MA). The relative formation of calcium deposition of each group was semi-quantitatively assessed as an OD value at 590 nm per milligram of total protein and then normalized to the fold changes by using the “No loading” group as the reference value (set to 1) and expressed as the mean ± SD. All the experiments were performed in triplicate.
In vitro RhBMP-2 activity assay
Firstly, we prepared the composite hydrogel of Gel/PMs-15 and 26SCS/Gel/PMs-15 according to the formulations listed in and simultaneously loaded GFs into the composite hydrogel following a consistent proportion as 100 ng rhBMP-2/mg PLGA and 10 ng rhVEGF165/mL gelatine/PBS solutions. The different groups with different loading cargos at the specified location designed in were respectively named as 0 (negative control, no loading), B (rhBMP-2 loaded in PMs), V (rhVEGF165 loaded in Gel), B/V (rhBMP-2 loaded in PMs and rhVEGF165 loaded in Gel) and B/V/S (rhBMP-2 loaded in PMs, rhVEGF165 and 26SCS loaded in Gel).
Table 3. The composite hydrogel gel/PMs-15 with different loading cargo used for extracts preparation.
Each sample mentioned above was immersed in 2 ml culture medium at 37 °C. At predetermined time points of 3 d and 10 d, the extract was collected under sterile conditions and centrifuged. Then the supernatant was collected and stocked at −80 °C for further experiments. All samples were analyzed in triplicate.
The bioactivity of rhBMP-2 released from the hybrid Gel/PMs hydrogel was estimated by determining its osteogenic ability to induce ALP enzymatic activity in MC3T3-E1 cells. Three steps were involved: (I) After the extracts of the B/V group at 3 d and 10 d were collected, the concentrations of rhBMP-2 and rhVEGF165 in the extracts were detected by ELISA using Human BMP-2 and VEGF165 Elisa Kits, respectively; (II) MC3T3-E1 cells were plated in 24-well plates (1 × 104 cells/well) and allowed to attach overnight. The culture medium was replenished the next day with the medium pre-collected at 3 d and 10 d, respectively. Culture medium with similar concentrations of rhBMP-2 and rhVEGF165, corresponding to those calculated from step (I), was used as the positive control (labelled as the B/V positive control); (III) ALP activity was measured according to standard procedures after 5 days in MC3T3-E1 cultures using standard kits and normalized to the total protein content [Citation27]. Each group was assayed in triplicate and the results were expressed as mean ± SD.
Bioactivity of the released RhVEGF165
The extracts of various samples were prepared in advance as described above. A series of experiments was then performed as follows.
HUVEC proliferation
To perform the HUVEC proliferation assay, the specific steps were as follows. (I) Grouping and the concentration of rhBMP-2 and rhVEGF165 in the extract were determined as described above; (II) HUVECs were placed in 96-well plates (5000 cells/well) and allowed to attach overnight. The culture medium was replenished the next day with the medium pre-collected at 3 d and 10 d, respectively. Culture medium with similar concentrations of rhBMP-2 and rhVEGF165, corresponding to those calculated from step (I), was used as the positive control (labelled as the B/V positive control); (III) After 2-day cell culture, the cell viability was determined using the same procedure as described above. All the experiments were performed in triplicate. The results were expressed in percentage of control (standard culture medium, scored as 100% viability) as mean ± SD.
In vitro sprouting assay
The in vitro sprouting analysis was conducted as previously reported [Citation28,Citation29]. Growth factor reduced Matrigel (BD Biosciences, Franklin Lakes, NJ) was thawed at 4 °C and 100 μL of the fully thawed Matrigel was added into each well of a glass bottom cell culture dish (glass diameter: 15 mm) on ice. The dish was then transferred to a cell culture incubator at 37 °C for 30 min to allow the Matrigel to gel. After HUVECs were cultured for 7 days with the various extracts collected at 10 days, cells were detached by trypsin and then seeded on the gel at a density of 105 cells/dish. After incubation for 4 h at 37 °C in a cell culture incubator, the dishes were observed under confocal laser scanning microscopy (CLSM, LEICA, Wetzlar, Germany). At least five fields were acquired for each substrate and the total capillary tube length and number of branch points per field were counted by a blinded observer using NIH Image J 1.45 software (Bethesda, MD).
Evaluation of NO production
Production of NO in HUVECs was evaluated using the NO-specific fluorescent dye 3-amino, 4-aminomethyl-2′, 7′-difluorescein, diacetate (DAF-FM DA, Beyotime, Haimen, China) as described previously [Citation30]. Cells were seeded at a density of 2 × 104 per glass bottom cell culture dish (glass diameter: 15 mm) and attached overnight. Then, the medium was replaced by the 10-day extracts of various samples. After 2 days, cells were treated with diluted DAF-FM DA at 37 °C in darkness for 20 min. The fluorescence in cells was qualitatively analyzed under CLSM. All the experiments were performed in triplicate.
Quantitative real-time reverse transcription-polymerase chain reaction (qRT-PCR)
qRT-PCR was performed using a Bio-Rad real-time PCR system (Bio-Rad, Hercules, CA). Briefly, HUVECs were incubated for 7 days with various extracts collected at 10 days. Then, total RNA was extracted from the culture using RNAiso Plus (TaKaRa, Tokyo, Japan) and 1 μg of total RNA was used for cDNA synthesis with a PrimeScript RT reagent Kit (TaKaRa), according to the manufacturer’s instructions. qPCR was performed with a hot-start denaturation step at 95 °C for 30 s and then fluorescence intensity was recorded during 40 cycles at 95 °C for 5 s and 60 °C for 34 s. The forward and reverse primer sequences used are listed in . Glyceraldehyde-3-phosphatedehydrogenase (GAPDH), a template-free negative control, was included in each experiment. All reactions were run in triplicate for each sample and each gene. △CT was used to calculate the differences between target and control CT values for each sample: △CT = CT (target)−CT (control). The comparative expression level (fold change) was obtained by transforming the logarithmic values into absolute values using 2−△CT.
Table 4. Quantitative real-time PCR primer sets.
In vivo ectopic bone formation
Fifty microliters of the different GF-loaded composite hydrogels were respectively studied for in vivo ectopic bone formation. The GF-loading positions in the composite hydrogels were as same as Group B, B/V and B/V/S shown in . Group 0 and V were not selected for in vivo evaluation of bone regeneration since they are lacking osteoinductivity without rhBMP-2. The GF and 26SCS loading amount in the implants were adopted according to our previous study [Citation31,Citation32] as described in the following: Group B (5 μg rhBMP-2 loaded in PMs), Group B/V (5 μg rhBMP-2 loaded in PMs and 3 μg rhVEGF165 loaded in Gel), Group B/V/S (5 μg rhBMP-2 loaded in PMs, 3 μg rhVEGF165 and 5 μg 26SCS loaded in Gel). All procedures were carried out following approval of the Institutional Animal Care and Use Committee and followed the procedure for Animal Experimental Ethical Inspection of the Ninth People’s Hospital, which is affiliated with Shanghai Jiao Tong University School of Medicine.
Twenty-four male KM mice (Silaike Inc. Shanghai, China) with an average weight of 25 g were used and randomly divided into three groups of eight for each group of implant material. Implants were placed in the right leg muscle pouch of the mouse. Animals were sacrificed at 4 weeks and the implants were removed to investigate ectopic bone formation. Five explants from each group were detached from the surrounding soft tissue and weighed for both wet bone and ash content (incinerated at 800 °C in a muffle furnace for 4 h). The other three bony explants from each group with surrounding tissues were assigned for histological analysis. After fixation with 4% neutral buffered formalin for 24 h, the extracted bones were decalcified in 12.5% EDTA, dehydrated in a graded series of alcohol and embedded in paraffin. Serial sections were treated with haematoxylin/eosin (HE) and Masson’s trichrome staining. On the other hand, immunohistochemistry was performed. Briefly, the sections were deparaffinized and incubated with primary antibodies against CD31 (1:25 dilution; Abcam, Inc., Cambridge, UK) to identify blood vessels. ABC complex (Vector Laboratories, Inc., Peterborough, UK) was applied to the sections following incubation with a biotinylated secondary antibody (Dako Corporation, Carpinteria, CA) and DAB substrate (DAKO, Cambridge, UK) was used to stain the slides. The sections were then treated with haematoxylin and mounted. Finally, all the sections were visualized under a light microscope (TE2000U, Nikon, Japan).
Statistical analysis
All quantitative data were expressed as mean ± SD and were analyzed with Origin 8.0 (OriginLab Corporation, Northampton, MA). Statistical comparisons were carried out using analysis of variance (ANOVA). Statistical significance was attained at greater than 95% confidence level (p < .05).
Results and discussion
Physical properties of the hybrid delivery system
Bone formation is a highly dynamic process regulated by a variety of GFs. Both osteogenesis and vascular supply are considered as major challenges in bone regeneration. A hybrid microsphere-containing hydrogel system was designed in this study to provide disparate depots for dual growth factor loading and different releasing features. Particulates and hydrogels both constitute promising vehicles that can protect GFs from a loss of bioactivity and facilitate their therapeutic efficacy [Citation33]. However, as a single particulate vehicle can readily agglomerate and migrate but cannot provide support for cells or tissue regeneration, incorporating particles into a scaffold represents an optimized choice for many researchers [Citation34–36]. Conversely, the results of recent studies regarding the synergistic effects of 26SCS on BMP-2 and VEGF provide an impetus for integration. Thus, in the present study 26SCS was incorporated into a hydrogel network and considered along with rhBMP-2 and rhVEGF165. The hybrid Gel/PMs hydrogel was prepared by moderate photopolymerization of gelatine. Gelatine concentrations, content of PMs, as well as agitation rates comprised vital parameters influencing the dispersity and encapsulation efficiency of the microspheres (data not shown). Morphological observations of the hybrid hydrogels under optimized conditions are presented in . The macroscopic images captured by digital camera (Nikon) prior to and following freeze-drying shown in illustrate the homogeneous Gel/PMs hydrogel, in which the dark brown colour implies the crosslinking of tyrosine residues. In a representative SEM analysis of the cross-sections of the hybrid hydrogel Gel/PMs-15, porous microstructure could be observed (pore size of approximately 100 μm), containing PMs embedded on the walls of pores (). Analysis of the re-collected PMs using SEM and a laser particle size analyzer showed the particles to have a regular spherical shape () and average size of 15–25 μm (). With gelatin concentration decreasing to 50 mg/mL (L-Gel/PMs-15), the distribution of PMs in the composite hydrogel became not uniform and the porous structure of the composite hydrogel was destroyed (Figure S1). With gelatin concentration increasing to 300 mg/mL (H-Gel/PMs-15), the PMs could not generate since the viscosity of the hydrogel was too high.
Figure 1. Morphological characterization of the representative composite hydrogel Gel/PMs-15. (A,B) Gross view of Gel/PMs-15 before and after lyophilization; (C,D) The cross section morphology of Gel/PMs-15 obtained by SEM, D is amplified versions of designated sites; (E) The morphology and (F) Size distribution of the freeze-dried PMs.
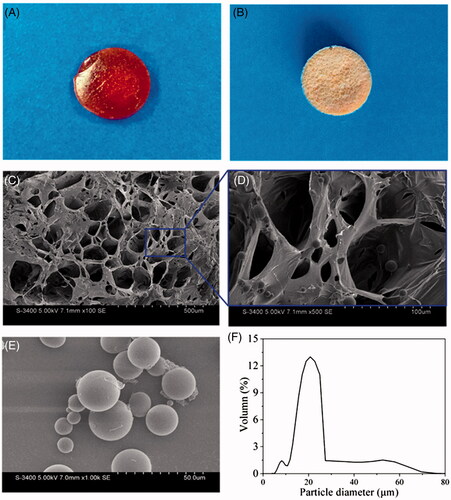
In order to deliver GFs to a wound site for healing purposes, several characteristics that implantable devices must possess should be taken into account when designing of a delivery vehicle, among which the mechanical congruency is an important aspect. For instance, the sufficient elasticity would make the implanted material conform to the size and shape of the wound and endow temporary mechanical support to withstand in vivo forces [Citation5]. Especially, as a possible candidate for bone tissue engineering materials, poor mechanical properties will limit the use of hydrogels for load-bearing applications such as the replacement of damaged or diseased tissues [Citation37]. Therefore, further improvement in mechanical properties of hydrogel is deemed necessary. In addition, the dynamic mechanical environment of the delivery site can also affect the release rate of GFs [Citation38,Citation39]. As a result, the mechanical congruency should also be optimized according to the close relationship between mechanical properties of the delivery system and external mechanical stimulus. In the present study, for improving the mechanical properties of the composite hydrogels, PMs not only act as one of the delivery vehicles, but also supply reinforcement. The influence of PMs content on the mechanical properties of the complex hydrogels was examined using constant strain-rate compression tests. depicts the typical stress − strain behaviour of Gel/PMs hydrogels with a series of PMs contents. The corresponding compressive moduli of complex hydrogels were increased significantly along with the improvement of PMs content, as displayed in . In particular, the 15 wt% PMs (Gel/PMs-15) exhibited a threefold increase in modulus (p < .001). The strengthening property might be attributed to the formation of physical inter-chain hydrogen bonds between the gelatine chain and the PLGA. In addition, the embedded polymeric microparticles could serve as cavity filling agents for energy adsorption, which resulted in the ability to endure deformation. showed swelling behaviour of the composite hydrogel with different PMs content in 24 h. Generally, all the selected hydrogel showed increasing trend in swelling over time, since the three-dimensional network structure of hydrogel could be filled with PBS solution which induced the increase of gel quality and swelling ratio. With addition of PMs, swelling ratio of the composite hydrogel decreased gradually, which indicates that PMs could partly inhibit swelling of the hydrogel and slow down its degradation rate. The degradation rate of PMs directly affects the release rate of the encapsulated GF. is the change curve of molecular weight of PMs released from the composite hydrogel. PMs could degrade over time. After 14-d incubation, the molecular weight of PMs with initial molecular weight of 1 × 105 reduced to 4 × 104, while PMs with initial molecular weight of 2 × 104 reduced to 1.2 × 104. Although PMs composed of PLGA with large molecular weight (1 × 105) showed a sharper recession curve, its molecular weight was still much larger than the PMs with small molecular weight (2 × 104) after the same degradation time.
Figure 2. (A) Representative stress − strain curves and (B) compressive modulus of the composite hydrogels with different content of PMs. *p < .01, **p < .001, compared to the group without microspheres; (C) the effect of PMs content on the swelling profile of the composite hydrogel; (D) dependence of molecular weight of the released PMs on degradation time during in vitro degradation in PBS solution at 37 °C.
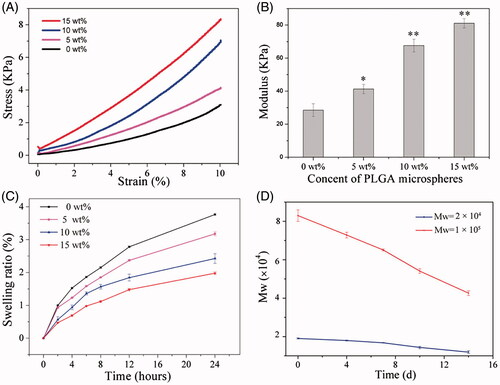
Cell affinity exploration
The cytotoxicity of pure photopolymerizable hydrogel (Gel) and the representative complex hydrogel (Gel/PMs-15) was evaluated by measuring the mitochondrial metabolic activity of MC3T3-E1 cultured on tissue culture plastic in the presence of the collected extracts using a standard MTS assay (). Both groups exhibited excellent cell viability, ranging from 88% (1 d extracts) to 113% (14 d extracts). Assessment of the adhesion of MC3T3-E1 was performed at 24 h after seeding on the surface of the Gel/PMs-15. As shown in , cells displayed an elongated shape with outstretched filopodia-like extensions. These suggested that the complex hydrogel was noncytotoxic and supported the cell viability and cell adhesion. Gelatine is known to be a suitable biomaterial [Citation24] and PLGA was approved by the Food and Drug Administration (FDA). Furthermore, the photocrosslinking reagents including [RuII(bpy)3]2+ and SPS have been demonstrated to show no significant cytotoxic effects at the concentrations present in the photopolymerized material in vitro [Citation23,Citation40]. It has also been independently reported in research on ruthenium-labelled polysaccharide-based hydrogels that the ruthenium complex did not cause any cytotoxicity [Citation41].
Figure 3. (A) Cell cytotocity of pure photopolymerizable hydrogel (Gel) and the complex hydrogel (Gel/PM-15). Standard culture medium was used as a positive control, #p < .05, *p < .01; (B) Cell adhesion of MC3T3-E1 cells at 12 h after seeding on the surface of the Gel/PM-15 under confocal laser scanning microscopy (200× and 600×). DAPI staining for nuclei and FITC- phalloidin staining for cytoskeletal F-actin fibres.
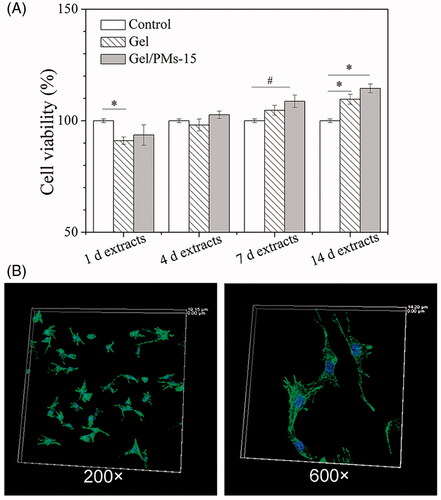
In vitro releasing behaviours of the loaded GFs
Mounting evidence supports that developing versatile therapeutic formulations for controlling the local dose and spatiotemporal release of both osteogenesis and angiogenesis GFs to sites of defective tissue are essential to promote bone regeneration. The current studies have shown several dual delivery systems of BMP-2 and VEGF which are favourable for osteogenesis and vascularization. Sukul et al. [Citation42] demonstrated the potential of a sustained release of BMP-2 and VEGF from nano-cellulose loaded in sponge biphasic calcium phosphate to induce the improved bone regeneration. It’s reported that BMP-2 and VEGF were together released from the scaffold with similar kinetic rates since they were both embedded in nano-cellulose simultaneously. With the in-depth studies, it’s found that expressions of BMP-2 and VEGF are with temporal sequences [Citation43] during the natural bone regeneration process. Additionally, the bioactivity of BMP-2 and VEGF is concentration and time-dependent [Citation44,Citation45]. Therefore, new advanced biomaterials capable of releasing BMP-2 and VEGF in a similar temporal release profile concordant with their natural expression might be beneficial for bone regeneration. Recently, some sustained delivery systems with capacity of sequential release of BMP-2 and VEGF have been developed and investigated for an improved performance in augmenting bone formation [Citation46–48]. For example, Egri et al. [Citation49] loaded BMP-2 into the poly-L-lactide (PLA)-polyethylene glycol (PEG)-PLA triblock copolymers scaffold for a slow constant release depending on the copolymer degradation and loaded VEGF onto the surface of pores inside the scaffold for a fast release in about 1 week relying on the surface dissolution. Barati et al. [Citation50] prepared a patterned hydrogel incorporated with different nanogels (NGs), which were based on PEG macromers chain-extended with short lactide (L) and glycolide (G) segments for grafting and timed-release of BMP-2 and VEGF. The release profiles of BMP-2 and VEGF were regulated by PEG molecular weight, LG segment length and L/G ratio, which were key items with close connections to the physicochemical properties of NGs. It’s worth mentioning that the majority of the current dual delivery systems including the abovementioned ones are preforming to be a specific appearance resulting in difficulties for filling irregular-shaped defects or invasive surgery [Citation51]. In the present study, the composite hydrogel Gel/PMs could be used not only as a preformed hydrogel, but also as injectable scaffolds. It could be photocrosslinked to form gel in situ by irradiation of LED dental curing lamp. Besides, it’s a facile method in this study to generate the microspheres by simply dropping PLGA solution in to the pre-gel solution. By this way, there’s no need to prepare the microspheres in advance and it’s easy to get the composite hydrogel with ability of releasing GFs in varying profiles by altering loading position. However, there are also some challenges of Gel/PMs prepared in this study. Due to the high water content of the gelatine hydrogel, although the mechanical strength was improved by the incorporation of PMs, it’s still limited when used under mechanically challenging conditions, as found in the load-bearing bone and cartilage tissues [Citation52].
In our developed composite hydrogel, the releasing rates of GFs relied mainly on the crosslinking density of the hydrogel network, as well as the molecular weight of the polymeric microspheres, which could be regulated expediently. Furthermore, a versatile releasing mode was obtained that could be manipulated by altering the location of the GFs. In addition, the effects of 26SCS were also inspected. showed various release profiles of rhBMP-2 and rhVEGF165 from the different composite hydrogel Gel/PMs-15 discribed in . When altering the molecular weight of PLGA thereby changing the degradation time of the PMs, the release behaviours of rhBMP-2 capsuled in PMs varied while rhVEGF165 capsuled in Gel showed similar release profiles (comparing ). Both in the composite hydrogel Group 1 and Group 2 described in , rhVEGF165 demonstrated a rapid release lasting for 16 days with a cumulative release amount over 80%. In Group 1, PMs composed of PLGA with a large molecular weight of 1 × 105, rhBMP-2 showed a slow release to 20% of total loading amount for 30 days. The majority of the released rhBMP-2 occurred at the early release stage while there is nearly no release at the later release stage. Since the release rate of rhBMP-2 encapsulated in PMs closely depended on degradation of PLGA and the large molecular weight (1 × 105) degraded rather slowly, the burst release of rhBMP-2 at the early stage was mainly due to the not encapsulated part. In Group 2, PMs composed of PLGA with a relative small molecular weight of 2 × 104, rhBMP-2 showed a fast release over 60% of total loading amount for the first 14 days and then a sustained release to 80% after 30 days. It could be seen that PMs generated by relatively small molecular weight of PLGA (2 × 104) were easy to degrade and release the encapsulated GF. Considering its mechanical strength and dispersity properties, Gel/PMs-15 using PLGA with molecular weight of 2 × 104 was thus selected for further examination unless otherwise noted. In , the location of rhVEGF165 and rhBMP-2 was swapped compared to the loading mode in Group 2 in . By this way, we can get a rapid release of rhBMP-2 and sustained release of rhVEGF165. Actually, independent on the loaded GFs, Gel/PMs could provide a rapid release through the hedrogel matrix and a sustained release through the interior PMs. This combined release kinetics occurred mainly because of the disparity between the two different carriers: GF incorporated in the gelatine matrix had a pulsatile diffusing model owing to the hydrophilic and swelling feature of the hydrogel; whereas GF encapsulated in PLGA microspheres exhibited a continuous delivery pattern because of its hydrophobic property and comparative slow degradation rate of the polyester barrier. Thus, it can be observed that the gelatine hydrogel combined with PMs constituted a suitable platform for dual growth factor delivery.
Figure 4. Release kinetics of rhBMP-2 and rhVEGF165 from the different composite hydrogel Gel/PMs-15 discribed in : (A) Group 1; (B) Group 2; (C) Group 3; (D) Group 4 (Dot line: without dopping 26SCS, same data in B; solid line: with dopping 26SCS).
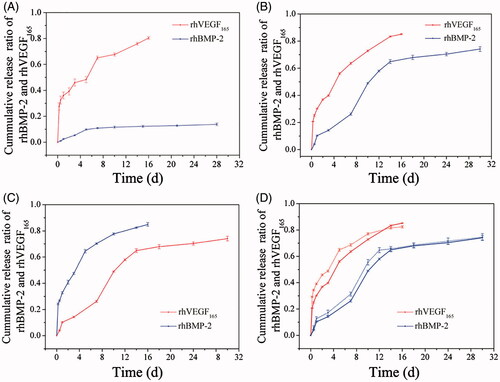
The effect of doping 26SCS on the release patterns of rhBMP-2 and rhVEGF165 from Gel/PMs-15 and 26SCS/Gel/PMs-15 were compared as shown in . Although similar release tendencies were obtained, the 26SCS-entrapped groups exhibited a slight downward trend compared to those without 26SCS. Consistent with our previous reports [Citation53,Citation54], 26SCS contained more N- and O-sulphated groups, which could bind to either BMP-2 or VEGF via spatially matching electrostatic interactions. Thus, decelerated releasing curves could be acquired in 26SCS-entrapped hybrid systems.
In vitro osteogenesis induced by the composite hydrogel containing GFs
In vitro osteogenesis induced by the composite hydrogels containing GFs was, respectively, measured at 7 days and 21 days for ALP activity and calcium nodule formation assays. For a short-term observation at 7 days, Group 3 in , which displayed a rapid release of rhBMP-2 and a slow release of rhVEGF165 exhibited the largest area of ALP-positive staining and the strongest ALP activity compared to the other groups (). With the synergy effect of 26SCS, Group 4 demonstrated significant increase in ALP activity compared with Group 2. Nevertheless, for a long-term observation to 21 days, qualitative and quantitative analysis of mineralization assay () indicated that Group 2 and 4 providing an initial release of rhVEGF165 from the Gel and a sustained release of rhBMP-2 from PMs significantly promoted osteogenic differentiation of BMSCs compared to the other groups. Similarly, Group 4 showed a stronger promotion in calcium nodule formation than Group 2 due to the contributing synergistic effects of 26SCS. By the way, both results of ALP activity and calcium nodule formation investigations suggested that molecular weight of the PMs could significantly affect the release rate of the embedded growth factor and subsequently alter its effect.
Figure 5. In vitro osteogenesis induced by the GFs-loaded composite hydrogels shown in . The composite hydrogel Gel/PMs-15 with no growth factor loading labelled as “No loading” was used as a control group. (A) ALP staining (100×) and the quantitative assay after 7 days; (B) Alizarin Red-S staining (100×) and the quantitative assay after 21 days. * and # Indicate statistical significance compared to Group “No loading” and 1, respectively (p < .05); ★ is significant differences between the indicated groups (p < .05).
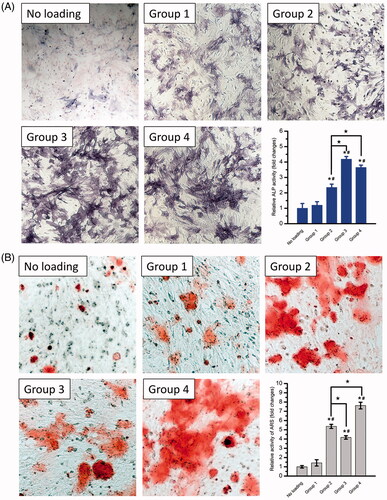
During the natural bone regeneration process, VEGF is expressed in the early phases of bone formation while BMP expression comes later [Citation43]. Previous studies also showed that normal VEGF expression is upregulated within the first 10 days of bone fracture with a peak around days 5–10 [Citation55,Citation56]. The early VEGF release likely preceded BMP-2-induced osteogenesis [Citation43]. Accordingly, in the present study, as well as considering the actual effect of the different time profiles of the released GFs on the in vitro osteogenesis, an initial release of rhVEGF165 from the Gel followed by the sustained release of rhBMP-2 from PMs (Group 2 and 4 in ) was designed as the ideal release profile of GFs to mimic the natural process for a better bone regeneration.
In vitro bioactivity of released rhBMP-2
Firstly, the released concentrations of rhBMP-2 and rhVEGF165 in the collected medium were determined at different time (rhBMP-2: 0.15 ± 0.02 μg/mL at day 3 and 0.55 ± 0.03 μg/mL at day 10; rhVEGF165: 4.51 ± 0.08 ng/mL at day 3 and 7.87 ± 0.11 ng/mL at day 10). Culture medium with similar concentrations of fresh GFs, corresponding to that calculated from above steps, was used as a B/V positive control to compare the bioactivity of GFs for the subsequent examinations.
We exposed the MC3T3-E1 cells to different release media (0, B, V, B/V/S and B/V positive control). In vitro bioactivity of the released rhBMP-2 from various carriers was quantitatively estimated by ALP assay, which generally served as a standard early marker of bone differentiation induction from progenitor cells. As apparent in , the released rhBMP-2 collected at day 3 and 10 induced significantly increased ALP activity (p < .05) when compared to the “0” group. No distinct differences were observed among groups B, B/V and B/V positive control, implying the maintenance of bioactivity from the releasing systems. Notably, the released rhBMP-2 in group B/V/S revealed a significant increment in ALP level compared to the B/V group (p < .05). This was inconsistent with the above-mentioned releasing curves wherein a lower amount of rhBMP-2 was detected in the 26SCS-containing group. The divergence was most likely due to the contributing synergistic effects of the 26SCS. We have observed in our previous work [Citation20,Citation31] that the presence of low doses of 26SCS could significantly enhance ALP activity and mineralization of BMP-2 in vitro, as well as orthotopic bone formation in vivo. It was presumed that an antagonistic protein such as noggin might fail to block the function of BMP-2 in the presence of 26SCS [Citation20]. Thus, it is considered reasonable that the rhBMP-2 released from the 26SCS-doping B/V/S group displayed the highest ALP activity, implying its improved capacity of inducing osteoblastic differentiation.
Figure 6. In vitro bioactivity of the released GFs. In advance, the extracts of the different composite hydrogels as shown in were collected at predetermined time points and subsequently used for in vitro study. The concentrations of GFs in the extracts collected from the B/V group were detected by ELISA using Human BMP-2 and VEGF165 Elisa Kits, respectively. Culture medium with similar concentrations of GFs, corresponding to that calculated from above steps, was used as a B/V positive control. (A) The effect of the released GFs on ALP activity. MC3T3-E1 cells were cultured with the 3d and 10d collected medium for 5 days. *p < .05 is significant difference to the corresponding 0 and V group; #p < .05 denote significant difference between the designated groups. (B) Effects of the released GFs on cell viability of HUVECs. HUVECs were cultured with the 3d and 10d collected medium for 2 days. *p < .05, compared to the corresponding 0 and B group; #p < .05, compared to the B/V group.
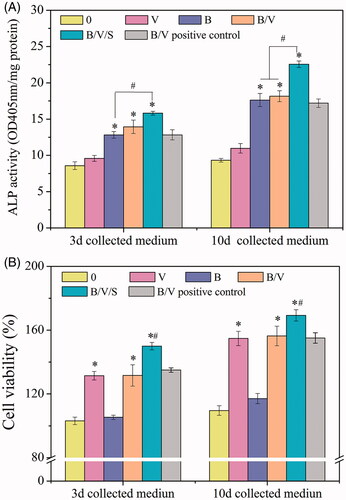
In vitro bioactivity of released rhVEGF165
Cell viability
A similar procedure was performed using HUVECs to evaluate the angiogenesis activity of the released rhVEGF165 in vitro. Cells were exposed to different release media (0, B, V, B/V/S, and B/V positive control). VEGF is regarded as a critical regulatory molecule in cell proliferation. Compared to the groups without rhVEGF165 in the released media (“0” and B), other groups (V, B/V, B/V/S, and B/V positive control) had enhancement effects on the cell viability of HUVECs (), demonstrating the positive effect of rhVEGF165 on cell viability. Similar cell viability effects were observed among groups V, B/V and B/V positive control, indicating that released rhVEGF165 did not lose its original bioactivity. Furthermore, with the 26SCS-incorporation in group B/V/S, there was a significant increase in cell viability comparing to group B/V.
Capillary tube formation
The capillary tube network formation is an important process during angiogenesis. The effects of various released media on the capillary tube forming capability of HUVECs were investigated under CLSM and quantified by a blinded observer in terms of total capillary tube length and number of branch points per field. As shown in , HUVECs cultured in growth factor-blank medium (Group 0) did not form tubes after 4 h on growth factor-reduced matrigel. In contrast, vascular tubes were obviously formed in a capillary-like network in other growth factor-loading groups, and successively increased with respect to the total capillary tube length and number of branch points per field (p < .05) with the released media of B, V, B/V, and B/V/S (). Notably, both total capillary tube length and number of branch points per field of B/V were increased by 1.5-fold and 1.35-fold, respectively, compared to the V group, implying the potential revascularization of rhBMP-2. As expected, a powerful spouting capacity was exhibited in the B/V/S group, which represented approximately 2.2- and 2.8-fold (p < .05) augmentation compared to the V group, demonstrating the synergetic effects among 26SCS, rhVEGF165 and rhBMP-2.
Figure 7. Effects of rhBMP-2, rhVEGF165 and 26SCS in collected medium at day 10 on HUVEC sprouting. Microscopy images of tube network formation under CLSM (A); Total capillary tube length (B) and number of branch points (C) per field in the different released medium. *p < .05 is significant difference to the corresponding 0 group; #p < .05 denote significant difference between the designated groups.
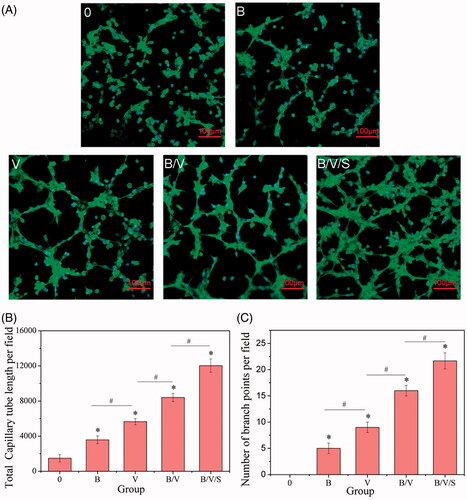
NO is an important signalling pathway in VEGF-induced angiogenesis. RhVEGF165 binding with surface receptors of endothelial cells can activate nitric oxide synthase and secret NO, thus promoting the growth and differentiation of endothelial cells. depicts intracellular NO production assayed by the fluorescence intensity of DAF-FM DA under CLSM. After being incubated in different extracts (“0”, B, V, B/V or B/V/S) for 2 days, incrementally higher levels of NO secretion were obtained for the V, B/V and B/V/S groups in comparison with the control (“0”) and B group. As deduced above, rhBMP-2 may have the potential to promote the secretion of NO. Furthermore, 26SCS was verified to have synergetic effects with rhBMP-2 or rhVEGF165, resulting in marked activity of HUVECs and increased NO signal intensity.
Relative gene expression of endothelial markers
Gene expressions of endothelial markers Flt-1, KDR, CD31 and vWF were assayed by qRT-PCR at day 7 after HUVECs were cultured with different extracts collected at 10 days. Flt-1 and KDR are the two tyrosine kinase receptors of VEGF; i.e. VEGFR-1 and VEGFR-2. Specifically, KDR is the main mediator of the angiogenic, mitogenic and permeability-enhancing effects of VEGF [Citation57]. PECAM, or CD31, is an adhesion molecule expressed by vascular endothelial cells, platelets, monocytes, neutrophils and naive T lymphocytes where it mediates the interactions of these cells during the process of trans-endothelial cell migration, thrombosis, wound healing and angiogenesis [Citation58]. Additionally, vWF, a large multimeric glycoprotein present in blood plasma, is produced constitutively as ultra-large vWF in the endothelium and expressed specifically during angiogenesis [Citation59]. As illustrated in , compared to the control group (“0”), upregulated gene expressions of Flt-1, KDR, CD31 and vWF were observed in the rhVEGF165-loaded groups V, B/V and B/V/S (p < .05), confirming the bioactivity of released rhVEGF165. In particular, significant augmentation was observed for the 26SCS-doping B/V/S group, suggesting that 26SCS might improve the binding affinity of rhVEGF165 and its receptors. With respect to the single rhBMP-2 group (B), only CD31 expression increased obviously, whereas little change was determined in the other gene expression levels.
Ectopic bone formation in the mouse thigh muscle
We evaluated in vivo ectopic bone formation induced by the implants of the GF-loaded composite hydrogel “B”, “B/V” and “B/V/S” placed in the thigh muscle pouches of mice 4 weeks post-implantation, respectively. Overall, new bone formation was found in each group (). In wet state, the weight of the newly formed ectopic bones induced by multiple factor delivery system (Group B/V and B/V/S) were significantly elevated (p < .05, 0.701 ± 0.031 g and 0.840 ± 0.067 g, respectively) compared with the single rhBMP-2 delivery system (Group B, 0.518 ± 0.061 g). With the synergistic effect of 26SCS, rhBMP-2, and rhVEGF165 released from the composite hydrogel “B/V/S” induced further augmented ectopic bone (p < .05) compared to Group B/V. On the other hand, after incinerated to ash, both Group B/V and B/V/S showed significant differences (p < .05) in bone ash content (0.060 ± 0.005 g and 0.065 ± 0.007 g) to Group B (0.041 ± 0.006 g), while there was no significant difference in ash content between Group B/V and B/V/S.
Figure 10. Ectopic bone formation induced by the GF-loaded composite hydrogels as shown in at 4 weeks post-implantation; (A) Wet and ash weight of ectopic bone. *p < .05 is significant difference to the corresponding Group B; #p < .05 is significant difference to the corresponding Group B/V; (B) Histology and immunohistochemistry analysis of ectopic bone sections. Images in the first row are stained by Haematoxylin/eosin (HE) staining in 20×; Images in the middle row are stained by Masson’s trichrome staining in 100×; Images in the last row are stained by immunohistochemical CD31 staining in 400×.
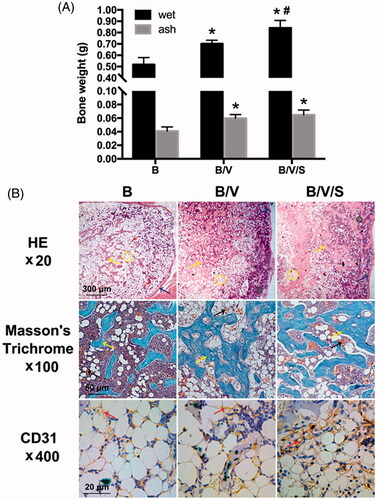
Histological analysis of the ectopic bone samples at 4 weeks were also investigated and shown in . In HE staining (the first row of ), we could find the tissue in all explanted groups with new bone formation containing lamellar bone (blue arrow), bone trabeculae (yellow arrow) and medulla ossium flava (yellow circle). Comparing these three groups, the Group B/V/S showed a greater area of bone trabeculae, stained a pink colour surrounded by the rich medulla ossium rubra (green circle), while the Group B showed a greater area of medulla ossium flava mainly composed of fat cells. Besides, the Group B/V/S displayed a most integrated lamellar bone shell outside and dense bone trabeculae inside. In masson’s trichrome staining (the middle row of ), the Group B/V and B/V/S showed more intensive and connective bone trabeculae (yellow arrow) stained a blue colour, which were smaller and more dissociative in the Group B. In comparison to the Group B and B/V, the Group B/V/S showed more notable blood capillaries (black arrows) in the medulla ossium rubra (green circle) surrounding the dense bone trabeculae. Furthermore, an integrated bone tissue structure had formed with a defined architecture of mature bone trabeculae, medulla ossium flava, and medulla ossium rubra in the Group B/V/S. In immunohistochemistry staining of CD31, a greater area of positive CD31 staining in the new bone matrix again confirmed the formation of blood vessels (red arrow) stained with brown colour in the Group B/V/S at 4 weeks (the last row of ) compared to the Group B and B/V. It’s worth mentioning that, with the presence of 26SCS, the in vivo new bone formation and vascularization induced by rhBMP-2 and rhVEGF165 were apparently improved.
In summary, based on the above results, we found that the selected sequential release of rhBMP-2 and rhVEGF165 accompanied by 26SCS from the composite hydrogel could not only provide an enhanced bone regeneration, but also demonstrate remarkable advantages in pro-angiogenesis. Here, we hypothesized the possible reasons. Rapid release of rhVEGF165 from the hybrid hydrogel can exert its effects on HUVECs by binding to its VEGFR tyrosine kinase receptors, leading to the upregulated gene expression of Flt-1 and KDR (). Then, the relevant cellular responses including proliferation (), capillary tube formation (), NO secretion (), and relative gene expression of endothelial markers (), are stimulated to increase and expand the vascular network. On the other hand, in addition to its well-known osteoinductive capability, rhBMP-2 also acts as a pro-angiogenic cytokine for endothelial cells during fracture repair. Treatment of HUVECs with rhBMP-2 thus induces tube formation (), NO secretion (), and CD31 expression (). Nevertheless, rhBMP-2 had little effect on HUVEC proliferation () and receptor binding of rhVEGF165 (), which was consistent with the results of a previous study demonstrating that HUVECs express the type I BMP receptors ALK2, ALK3 and ALK6 and the type II receptor BMPR-II, which are required to transmit BMP-2-induced cell signalling [Citation60,Citation61].
It is worth noting that 26SCS in this sequential release system is pretty necessary. With the addition of 26SCS, both in vitro osteogenesis and angiogenesis, and in vivo ectopic bone formation induced by the GFs loaded system were dominantly augmented. According to our previous study, 26SCS, a chitosan-derivative polysaccharide, can enhance the bioactivity of BMP-2 through a synergistic binding and block the antagonist action of noggin [Citation20]. Within a certain concentration, 26SCS can directly promote the proliferation and capillary tube formation of HUVECs [Citation21]. Besides, there may be a synergistic effect between 26SCS and rhVEGF165 depending on its 2-N- and 6-O-sulphation group [Citation19], which may prolong the half-life, regulate the affinity between rhVEGF165 and its receptors, and improve rhVEGF165-mediated angiogenesis. Although, 26SCS didn’t dramatically affect the sequential release of GFs from the composite hydrogel, its synergic characteristic made the scaffolds containing a combination of rhBMP-2, rhVEGF165 and 26SCS more effective and promising for bone regeneration in clinic.
Conclusions
The utility of rhBMP-2 and rhVEGF165 has been well documented in mediating interactions between host tissue and implants. In this study, we have presented a hybrid hydrogel that is able to deliver independently tunable amounts of active rhBMP-2 and rhVEGF165 over different timeframes and induce successive responses of cells (. It is important to note that 26SCS can have synergistic effects on both rhBMP-2 and rhVEGF165, inducing more prominent effects on pre-osteoblast cells and endothelial cells. The delivery of rhBMP-2 and rhVEGF165 changed the microenvironment significantly compared to single GFs, especially upon incorporation of 26SCS. Notably, fabrication of the hybrid hydrogel is facile and convenient, and the inclusive GFs can be flexibly regulated with modulated release according to the various requirements of tissue repair. In summary, we envisage that the synergistic action among 26SCS, rhBMP-2 and rhVEGF165 represents a promising protocol to take advantage of multiple GFs. Such a strategy will afford a versatile platform to develop novel scaffolds for tissue regeneration.
Disclosure statement
No potential conflict of interest was reported by the authors.
Additional information
Funding
References
- Bolland BJRF, Tilley S, New AMR, et al. Adult mesenchymal stem cells and impaction grafting: a new clinical paradigm shift. Expert Rev Med Devices. 2007;4:393–404.
- Han T-Y, Liu X-W, Liang N, et al. In vitro effects of recombinant adenovirus-mediated bone morphogenetic protein 2/vascular endothelial growth factor 165 on osteogenic differentiation of bone marrow mesenchymal stem. Cells. Artif Cells Nanomed Biotechnol. 2017;45:108–114.
- Carano RAD, Filvaroff EH. Angiogenesis and bone repair. Drug Discov Today. 2003;8:980–989.
- Li J, Zhang Y-P, Kirsner RS. Angiogenesis in wound repair: angiogenic growth factors and the extracellular matrix. Microsc Res Tech. 2003;60:107–114.
- Chen F-M, Zhang M, Wu Z-F. Toward delivery of multiple growth factors in tissue engineering. Biomaterials. 2010;31:6279–6308.
- Luginbuehl V, Meinel L, Merkle HP, et al. Localized delivery of growth factors for bone repair. Eur J Pharm Biopharm. 2004;58:197–208.
- Chen F-M, An Y, Zhang R, et al. New insights into and novel applications of release technology for periodontal reconstructive therapies. J Control Release. 2011;149:92–110.
- Bandyopadhyay A, Yadav PS, Prashar P. BMP signaling in development and diseases: a pharmacological perspective. Biochem Pharmacol. 2013;85:857–864.
- Leach JK, Kaigler D, Wang Z, et al. Coating of VEGF-releasing scaffolds with bioactive glass for angiogenesis and bone regeneration. Biomaterials. 2006;27:3249–3255.
- Patel ZS, Ueda H, Yamamoto M, et al. In vitro and in vivo release of vascular endothelial growth factor from gelatin microparticles and biodegradable composite scaffolds. Pharm Res. 2008;25:2370–2378.
- Kanczler JM, Ginty PJ, White L, et al. The effect of the delivery of vascular endothelial growth factor and bone morphogenic protein-2 to osteoprogenitor cell populations on bone formation. Biomaterials. 2010;31:1242–1250.
- Wang DS, Miura M, Demura H, et al. Anabolic effects of 1,25-dihydroxyvitamin D3 on osteoblasts are enhanced by vascular endothelial growth factor produced by osteoblasts and by growth factors produced by endothelial cells. Endocrinology. 1997;138:2953–2962.
- Kaigler D, Krebsbach PH, West ER, et al. Endothelial cell modulation of bone marrow stromal cell osteogenic potential. FASEB J. 2005;19:665–667.
- Tsai T-L, Wang B, Squire MW, et al. Endothelial cells direct human mesenchymal stem cells for osteo- and chondro-lineage differentiation through endothelin-1 and AKT signaling. Stem Cell Res Ther. 2015;6:88.
- Deckers MML, van Bezooijen RL, van der Horst G, et al. Bone morphogenetic proteins stimulate angiogenesis through osteoblast-derived vascular endothelial growth factor a. Endocrinology. 2002;143:1545–1553.
- Rennel ES, Hamdollah-Zadeh MA, Wheatley ER, et al. Recombinant human VEGF165b protein is an effective anti-cancer agent in mice. Eur J Cancer. 2008;44:1883–1894.
- Nakamura S, Kubo T, Ijima H. Heparin-conjugated gelatin as a growth factor immobilization scaffold. J Biosci Bioeng. 2013;115:562–567.
- Zhao B, Katagiri T, Toyoda H, et al. Heparin potentiates the in vivo ectopic bone formation induced by bone morphogenetic protein-2. J Biol Chem. 2006;281:23246–23253.
- Robinson CJ, Mulloy B, Gallagher JT, et al. VEGF165-binding sites within heparan sulfate encompass two highly sulfated domains and can be liberated by K5 lyase. J Biol Chem. 2006;281:1731–1740.
- Zhou H, Qian J, Wang J, et al. Enhanced bioactivity of bone morphogenetic protein-2 with low dose of 2-N, 6-O-sulfated chitosan in vitro and in vivo. Biomaterials. 2009;30:1715–1724.
- Cao L, Wang J, Hou J, et al. Vascularization and bone regeneration in a critical sized defect using 2-N,6-O-sulfated chitosan nanoparticles incorporating BMP-2. Biomaterials. 2014;35:684–698.
- Yu Y, Chen J, Chen R, et al. Enhancement of VEGF-mediated angiogenesis by 2-N,6-O-sulfated chitosan-coated hierarchical PLGA scaffolds. ACS Appl Mater Interfaces. 2015;7:9982–9990.
- Elvin CM, Brownlee AG, Huson MG, et al. The development of photochemically crosslinked native fibrinogen as a rapidly formed and mechanically strong surgical tissue sealant. Biomaterials 2009;30:2059–2065.
- Elvin CM, Vuocolo T, Brownlee AG, et al. A highly elastic tissue sealant based on photopolymerised gelatin. Biomaterials. 2010;31:8323–8331.
- Liu C, Liu D, Wang Y, et al. Glycol chitosan/oxidized hyaluronic acid hydrogels functionalized with cartilage extracellular matrix particles and incorporating bmscs for cartilage repair. Artif Cells Nanomed Biotechnol. 2018; 1–12. Available from: 10.1080/21691401.2018.1434662
- Wang Q, Cao L, Liu Y, et al. Evaluation of synergistic osteogenesis between icariin and BMP2 through a micro/meso hierarchical porous delivery system. IJN. 2017;12:7721–7735.
- Zhang J, Zhou H, Yang K, et al. RhBMP-2-loaded calcium silicate/calcium phosphate cement scaffold with hierarchically porous structure for enhanced bone tissue regeneration. Biomaterials. 2013;34:9381–9392.
- Hu X, Neoh KG, Zhang J, et al. Immobilization strategy for optimizing VEGF'S concurrent bioactivity towards endothelial cells and osteoblasts on implant surfaces. Biomaterials. 2012;33:8082–8093.
- Arnaoutova I, Kleinman HK. In vitro angiogenesis: endothelial cell tube formation on gelled basement membrane extract. Nat Protoc. 2010;5:628–635.
- Iantorno M, Chen H, Kim J-A, et al. Ghrelin has novel vascular actions that mimic PI 3-kinase-dependent actions of insulin to stimulate production of NO from endothelial cells. Am J Physiol. Endocrinol. Metab. 2007;292:E756–E764.
- Cao L, Werkmeister JA, Wang J, et al. Bone regeneration using photocrosslinked hydrogel incorporating rhBMP-2 loaded 2-N, 6-O-sulfated chitosan nanoparticles. Biomaterials. 2014;35:2730–2742.
- Zhang W, Wang X, Wang S, et al. The use of injectable sonication-induced silk hydrogel for VEGF(165) and BMP-2 delivery for elevation of the maxillary sinus floor. Biomaterials. 2011;32:9415–9424.
- Tayalia P, Mooney DJ. Controlled growth factor delivery for tissue engineering. Adv Mater Weinheim. 2009;21:3269–3285.
- Kim S, Kang Y, Krueger CA, et al. Sequential delivery of BMP-2 and IGF-1 using a chitosan gel with gelatin microspheres enhances early osteoblastic differentiation. Acta Biomaterialia. 2012;8:1768–1777.
- Chen F-M, Chen R, Wang X-J, et al. In vitro cellular responses to scaffolds containing two microencapulated growth factors. Biomaterials. 2009;30:5215–5224.
- Tang G, Zhang H, Zhao Y, et al. Prolonged release from PLGA/HAp scaffolds containing drug-loaded plga/gelatin composite microspheres. J Mater Sci: Mater Med. 2012;23:419–429.
- Luo Y, Zhang K, Wei Q, et al. Poly(MAA-Co-an) hydrogels with improved mechanical properties for theophylline controlled delivery. Acta Biomaterialia. 2009;5:316–327.
- Lee K, Silva EA, Mooney DJ. Growth factor delivery-based tissue engineering: general approaches and a review of recent developments. R Soc Interface. 2011;8:153–170.
- Lee KY, Peters MC, Anderson KW, et al. Controlled growth factor release from synthetic extracellular matrices. Nature. 2000;408:998–1000.
- Elvin CM, Danon SJ, Brownlee AG, et al. Evaluation of photo-crosslinked fibrinogen as a rapid and strong tissue adhesive. J Biomed Mater Res. 2010;93:687–695.
- Laïb S, Fellah BH, Fatimi A, et al. The in vivo degradation of a ruthenium labelled polysaccharide-based hydrogel for bone tissue engineering. Biomaterials. 2009;30:1568–1577.
- Sukul M, Nguyen TBL, Min Y-K, et al. Effect of local sustainable release of BMP2-VEGF from nano-cellulose loaded in sponge biphasic calcium phosphate on bone regeneration. Tissue Eng Part A. 2015;21:1822–1836.
- Kempen DHR, Lu L, Heijink A, et al. Effect of local sequential VEGF and BMP-2 delivery on ectopic and orthotopic bone regeneration. Biomaterials. 2009;30:2816–2825.
- Park J-C, Kim JC, Kim B-K, et al. Dose- and time-dependent effects of recombinant human bone morphogenetic protein-2 on the osteogenic and adipogenic potentials of alveolar bone-derived stromal cells. J Periodont Res. 2012;47:645–654.
- Li P, Bai Y, Yin G, et al. Synergistic and sequential effects of BMP-2, bFGF and VEGF on osteogenic differentiation of rat osteoblasts. J Bone Miner Metab. 2014;32:627–635.
- Geuze RE, Theyse LFH, Kempen DHR, et al. A differential effect of bone morphogenetic protein-2 and vascular endothelial growth factor release timing on osteogenesis at ectopic and orthotopic sites in a large-animal model. Tissue Eng Part A. 2012;18:2052–2062.
- Çakır-Özkan N, Eğri S, Bekar E, et al. The use of sequential VEGF- and BMP2-releasing biodegradable scaffolds in rabbit mandibular defects. J Oral Maxillofac Surg. 2017;75:221.e1–221.e14.
- Sharmin F, McDermott C, Lieberman J, et al. Dual growth factor delivery from biofunctionalized allografts: sequential VEGF and BMP-2 release to stimulate allograft remodeling. J Orthop Res. 2017;35:1086–1095.
- Eğri S, Eczacıoğlu N. Sequential VEGF and BMP-2 releasing PLA-PEG-PLA scaffolds for bone tissue engineering: i. design and in vitro tests. Artif Cells Nanomed Biotechnol. 2017;45:321–329.
- Barati D, Shariati SRP, Moeinzadeh S, et al. Spatiotemporal release of BMP-2 and VEGF enhances osteogenic and vasculogenic differentiation of human mesenchymal stem cells and endothelial colony-forming cells co-encapsulated in a patterned hydrogel. J Control Release. 2016; 223:126–136.
- Nahideh A, Effat A, Roya S, et al. Nanocomposite hydrogels for cartilage tissue engineering: a review. Artif Cells Nanomed Biotechnol. 2017;0:1–7.
- Boere KWM, Visser J, Seyednejad H, et al. Covalent attachment of a three-dimensionally printed thermoplast to a gelatin hydrogel for mechanically enhanced cartilage constructs. Acta Biomaterialia. 2014;10:2602–2611.
- Kong X, Wang J, Cao L, et al. Enhanced osteogenesis of bone morphology protein-2 in 2-N,6-O-sulfated chitosan immobilized PLGA scaffolds. Colloids Surf B Biointerfaces. 2014;122:359–367.
- Yao C, Roderfeld M, Rath T, et al. The impact of proteinase-induced matrix degradation on the release of VEGF from heparinized collagen matrices. Biomaterials. 2006;27:1608–1616.
- Pufe T, Wildemann B, Petersen W, et al. Quantitative measurement of the splice variants 120 and 164 of the angiogenic peptide vascular endothelial growth factor in the time flow of fracture healing: a study in the rat. Cell Tissue Res. 2002;309:387–392.
- Uchida S, Sakai A, Kudo H, et al. Vascular endothelial growth factor is expressed along with its receptors during the healing process of bone and bone marrow after drill-hole injury in rats. Bone. 2003;32:491–501.
- Shalaby F, Rossant J, Yamaguchi TP, et al. Failure of blood-island formation and vasculogenesis in flk-1-deficient mice. Nature. 1995;376:62–66.
- Piali L, Hammel P, Uherek C, et al. CD31/PECAM-1 is a ligand for alpha v beta 3 integrin involved in adhesion of leukocytes to endothelium. J Cell Biol. 1995;130:451–460.
- Sadler JE. Biochemistry and genetics of Von Willebrand factor. Annu Rev Biochem. 1998;67:395–424.
- Langenfeld EM, Langenfeld J. Bone morphogenetic protein-2 stimulates angiogenesis in developing tumors. Mol Cancer Res. 2004;2:141–149.
- Raida M, Clement JH, Leek RD, et al. Bone morphogenetic protein 2 (BMP-2) and induction of tumor angiogenesis. J Cancer Res Clin Oncol. 2005;131:741–750.