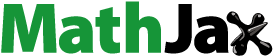
Abstract
This study aimed to develop porous microspheres with a suitable porous structure and mechanical property for cell delivery using a comparatively low molecular weight (MW) poly(lactide-co-glycolide) (PLGA) having a weak mechanical strength and fast degradation rate, which could be potentially used for treatment of corneal endothelial diseases. Porous microspheres of 30 kDa PLGA with different pore sizes were prepared by varying preparation conditions, and the microspheres with mean pore diameters approximately 0.5, 1, 2 and 3 times that of a single green fluorescent protein-expressing human embryonic kidney 293 cell, used as a model cell, were chosen for cell loading study. The microspheres with an average pore diameter two times greater than that of the single cell were found to be the most appropriate for efficient cell loading in the inner pore spaces, along with demonstrating a good mechanical property, injectability and biodegradability. To maximize the cell loading amount in the microspheres, the cell adhesive property of the microspheres and cell loading conditions were optimized, leading to approximately 4.2 times increase in the cell loading amount. The porous microspheres designed using the low MW PLGA hold promise as a delivery system of corneal endothelial cells for regeneration of the corneal endothelium.
Introduction
Cell therapy is drawing increasing interest owing to its considerable potential to treat wide ranges of degenerative diseases that could not be fully cured by existing medicines. For this purpose, therapeutic cells are transplanted to injured tissues, replace damaged or malfunctioning cells, and consequently recover the tissues. However, the vulnerable and sensitive nature of the cells often leads to low cell survival, delivery efficiency, and engraftment rate to host tissues, limiting their therapeutic effects [Citation1]. To address the problems, diverse cell delivery systems have been investigated, such as micro-particulate carriers [Citation2], hydrogels [Citation3] and films [Citation4].
Among the cell delivery systems, porous microspheres in particular have shown great promise because the pores allow physical spaces for cell loading and channels for supplying nutrients and oxygen [Citation5]. Porous microspheres are also able to cover and reside efficiently on defected tissues, thereby regenerating the tissues according to patient-specific anatomy (i.e. body structures) [Citation6]. In addition, they can be implanted in a minimally invasive manner via injection without requiring a serious surgical procedure [Citation7].
Despite the many advantages of porous microspheres as a cell delivery system, the comparatively small size of the microspheres has posed a challenge to establish a porous structure suitable for efficient cell loading. Most types of human cells are known to have mean diameters of approximately 15 μm in a suspended state [Citation8]. The pore diameters needed for loading therapeutic cells in the pore space of the microspheres, therefore, need to be considerably greater than those cells [Citation9,Citation10]. Furthermore, a high porosity with good interconnectivity among the pores is also essential to ensure more pores for cell loading in the limited volume of the microspheres. However, the large pore size and high porosity may render the porous microspheres mechanically weak, possibly resulting in the collapse of the microsphere skeletons, especially when physical stresses are exerted to the microspheres during handling, injection or implantation procedures. For this reason, microspheres for cell delivery should be carefully designed to possess large porous structures simultaneously with an appropriate mechanical strength.
Porous microspheres as a cell delivery system also have to exhibit a suitable biodegradation rate in the body depending on types of tissues and lesions. In our case, the ultimate goal was to develop porous microparticulate systems with large pores and high porosity that can deliver human corneal endothelial cells efficiently to degenerate corneal endothelium by magnetic guidance for treatment of corneal endothelial dysfunction. In this approach, it is expected that porous microspheres injected into the anterior chamber of the eye need to be gradually degraded in several weeks to deliver and integrate the corneal endothelial cells fully to the corneal endothelium, based on the general period of time taken for structural and functional recovery of the corneal endothelium reported in literatures on cell therapies for treatment of corneal endothelial dysfunction [Citation11–14]. To this end, a proper biodegradable material for preparing porous microspheres should be judiciously selected.
Among various biodegradable materials that have been explored to fabricate injectable or implantable microspheres, poly(lactide-co-glycolide) (PLGA) is greatly promising owing to its excellent safety, biocompatibility, and easy control of biodegradability [Citation1, Citation7]. To produce microspheres with the large porous structure and robust mechanical property, PLGA of a high molecular weight (MW) around and exceeding 100 kDa and of demonstrating increased crystal structures that can be seen with an unequal molar ratio of lactide (L) to glycolide (G) may be advantageous owing to its relatively great mechanical strength [Citation15]. However, such PLGA generally shows substantially long periods of biodegradation time more than several months up to a year [Citation15], which might be not appropriate for the purpose of our study. Thus, the design of porous microspheres as a cell carrier using PLGA of a low MW and an equal L/G ratio is necessary, although it would be difficult to establish large and strong pore structures using the low MW PLGA having a comparatively weak mechanical property.
In this paper, we therefore aimed to develop microspheres with large pore sizes and high porosity for efficient cell loading as well as sturdy mechanical property using PLGA of approximately 30 kDa and an equal L/G ratio as a preliminary study prior to investigating porous microspheres for cell-based therapy of corneal endothelial dysfunction. In addition, the cell adhesive property of porous PLGA microspheres prepared and cell loading conditions such as cell concentration, loading time and shaking speed were optimized to maximize the cell loading amount in the limited pore space of the microspheres, which is critical to transplant a sufficient amount of therapeutic cells to target tissues with minimal use of the porous carriers [Citation16]. For the cell loading study, green fluorescent protein (GFP)-expressing human embryonic kidney (HEK) 293 cells were used as a model therapeutic cell having similar size in diameter ∼15 μm [Citation17] to corneal endothelial cells [Citation18].
Materials and methods
Materials
Poly-l-lysine hydrobromide (70–150 kDa), PLGA (24–38 kDa and 100 kDa; L/G ratio = 50/50), and puromycin were purchased from Sigma-Aldrich Company (St. Louis, MO). Polyvinyl alcohol (66 kDa) was obtained from Duksan Pure Chemical Company (Seoul, Korea). Chloroform and ammonium bicarbonate were purchased from Samchun Chemicals (Yeosu, Korea). Dulbecco’s modified Eagle’s medium (DMEM) and foetal bovine serum (FBS) were obtained from WELGENE Inc. (Daegu, Korea). Penicillin/streptomycin was purchased from Life Technologies (Carlsbad, CA). EZ-Cytox cell viability assay kit was purchased from DoGenBio (Seoul, Korea).
Methods
Preparation of porous PLGA microspheres
Porous PLGA microspheres were prepared using PLGA of 30 kDa and an L/G ratio of 50/50 by double emulsion-solvent evaporation using ammonium bicarbonate as a porogen [Citation19], and different preparation conditions () were examined to control the pore formation.
Table 1. Preparation conditions of porous PLGA microspheres.
To assess the effect of PLGA concentration in the organic phase on the porous structure, PLGA was dissolved in chloroform at concentrations ranging from 30 g/L to 100 g/L. Ammonium bicarbonate aqueous solution (150 g/L) was added to the PLGA solutions at a volume ratio of the aqueous to organic phase of 30/70, followed by homogenization at 3000 rpm for 2 min using a homogenizer. The water-in-oil emulsion (3 mL) was then added to 1 g/L polyvinyl alcohol aqueous solution (150 mL) and the mixture was agitated at 600 rpm for 12 h using an overhead stirrer. The hardened porous PLGA microspheres with diameters of 150–300 μm were separated using sieves and washed with distilled water. The porous PLGA microspheres were then shaken in ethanolic sodium hydroxide composed of 99% 0.05 N NaOH aqueous solution and 1% absolute ethanol at 120 rpm for 40 min to open the pores [Citation20]. The porous PLGA microspheres were washed with distilled water and freeze-dried.
To investigate the influence of the volume ratio of the aqueous to the organic phase in the primary emulsion on the pore size of the microspheres, the above procedure was followed, except that 50 g/L PLGA chloroform solution was used as an organic phase and the volume ratio was varied from 20/80 to 40/60. To evaluate the impact of homogenization speed for preparing the primary emulsion, the homogenization speed was adjusted from 3000 rpm to 7000 rpm, with the PLGA concentration and volume ratio fixed at 50 g/L and 30/70, respectively.
Porous PLGA microspheres were also fabricated using PLGA of 100 kDa and L/G ratio of 50/50 as shown in . The pore opening procedure using the same ethanolic sodium hydroxide was performed for 1.5 h.
Observation of porous structure of microspheres
The porous structures of PLGA microspheres prepared were examined using a scanning electron microscope (SEM) (S-3000N, Hitachi, Tokyo, Japan). The microspheres were mounted on a metal stub, fixed using a carbon double-sided adhesive tape, and observed at an accelerating voltage of 2.0 kV.
Assessment of average pore size and porosity
The average pore size and porosity of PPM1, 3, 5, 6 and 9 were assessed with a mercury intrusion porosimeter (AutoPore IV 9500, Micromeritics, Norcross, GA). The porous microspheres (10 mg) were placed in a penetrometer with 5 mL bulb volume and 1.131 mL stem volume, and the intrusion chamber was filled with mercury. The microspheres were penetrated with mercury until maximal pressures optimized for each microsphere. The average pore size and porosity of the microspheres were then calculated.
Cell culture, lentiviral plasmid vector construction and lentivirus production
HEK293 cells and those transformed with large T antigen (HEK293T cells) were cultured in DMEM supplemented with 10% FBS, 50 U/mL penicillin and 50 μg/mL streptomycin in an incubator at 37 °C under 5% CO2. To construct a lentivirus-based GFP expression plasmid, the GFP gene was amplified using the following primers: forward: 5′-TGCTCTAGAGAAA-TGGCTAGCAAAGG-3′, reverse: 5′-CGCGGATCCTCAGTTGTACAGT-3′. The PCR products were inserted into pLVX-EF1a-IRES-Puro vector (Clontech Laboratories, Inc., Palo Alto, CA) using XbaI and BamHI. For virus production, lentiviral GFP plasmid vector was transfected into HEK293T cells together with pMD2G and psPAX2 vectors at a ratio of 3:2:1. Viral particles were harvested from the media after 48 h transfection and used to infect HEK293 cells. Infected HEK293 cells were selected on puromycin (16 μg/mL) for 2 weeks and verified for GFP expression.
Cell loading in porous PLGA microspheres with different pore sizes
PPM1, 3, 5, 6 and 9 (5 mg) suspended in phosphate-buffered saline (PBS) were placed in a 48-well plate, and PBS was removed. The microspheres were sterilized with ultraviolet (UV) irradiation for 30 min. GFP-expressing HEK293 cells (5 × 104) suspended in culture medium (500 μL) were added to the microspheres and incubated for 24 h at 37 °C under 5% CO2.
Evaluation of cell loading efficiency and cell loading amount in porous PLGA microspheres with different pore sizes
The loading efficiency and loading amount of GFP-expressing HEK293 cells in porous PLGA microspheres with different pore sizes were evaluated by water soluble tetrazolium (WST)-1 assay (EZ-Cytox cell viability assay kit, DoGenBio, Seoul, Korea). Culture medium was carefully removed from the 48-well plate, and the cell-loaded microspheres were rinsed with PBS. Five hundred microliters of WST-1 assay solution diluted 10 times with culture medium was added to the microspheres and incubated at 37 °C under 5% CO2 for 1 h. The absorbance at 450 nm was then measured using a microplate reader. The number of cells loaded in the porous PLGA microspheres was determined using a calibration curve generated using cells at known concentrations, and the cell loading efficiency and cell loading amount in the microspheres were calculated using the following equations:
(1)
(1)
(2)
(2)
where NLC indicates the number of GFP-expressing HEK293 cells loaded in the microspheres and NIC indicates the number of cells initially added to the microspheres. WPPM denotes the weight of the microspheres used (mg).
Confocal microscopy
Cell-loaded porous PLGA microspheres were placed in a confocal dish and were observed using a confocal laser-scanning microscope (CLSM) (Zeiss LSM 800, Carl Zeiss, Jena, Germany) at an excitation wavelength of 488 nm and detection wavelength of 400–700 nm. The cell-loaded microspheres were imaged using Plan-Apochromat 10 × /0.45 or 20 × /0.8 objectives. Z-stacks of the cell-loaded microspheres were obtained at 5-μm intervals and were reconstructed using the Zeiss image processing software (Oberkochen, Germany).
Measurement of mechanical strength of porous PLGA microspheres
Mechanical strength of PPM3 and 9 was evaluated using a texture analyser (TA Plus, Lloyd Instruments, Bognor Regis, UK) equipped with a 100 N load cell and a flat-tipped stainless steel probe of 5 mm in diameter. A monolayer of approximately 200 porous PLGA microspheres with diameters of 400–500 μm was fixed on a slide glass using double-sided adhesive tape. The probe compressed the monolayer at a rate of 0.002 mm/s. The mechanical strength of single microsphere was evaluated by dividing the load measured at 100-μm compression by the number of the microspheres tested.
Injectability testing
Injectability of PPM3 was evaluated as reported [Citation21], with a slight modification. Load profiles for injecting the PPM3 suspension were obtained using the texture analyser. PPM3 (5 mg) were suspended in distilled water (2 mL) and loaded in a 2-mL syringe equipped with 22- or 26-gauge needle. The syringe was tightly fixed in a clamp stand, with the needle positioned downward. The plunger end of the syringe was placed in contact with the stainless steel probe connected to a 10 N load cell. The force required to expel the content from the syringe was measured at a crosshead speed of 1 mm/s as a function of time. Injectability parameters, including plunger-stopper break-loose force (PBF), maximum force (Fmax) and dynamic glide force (DGF), were determined from the force–time plot [Citation21].
In vitro degradation study of porous PLGA microspheres
In vitro degradation study of PPM3 was performed in a simulated aqueous humour, a transparent fluid filling the anterior chamber of the eye, which was prepared as previously reported [Citation22]. PPM3 (10 mg) were added to the simulated aqueous humour (70 mL) in a glass vial, and incubated in a shaking water bath at 37 °C and 60 rpm. At predetermined time intervals (2, 4, 6, 9 and 12 weeks), PPM3 were washed with distilled water and freeze-dried, followed by measuring the remaining weight of the microspheres and observing the morphology of the microspheres using SEM.
Coating of surface of porous PLGA microsphere with polylysine
PPM3 was immersed and shaken in 0.01 N NaOH aqueous solution at 100 rpm for 30 min to dissociate the terminal carboxylic groups of PLGA and to promote the electrostatic interaction of the microspheres with polylysine exhibiting positive charges. The microspheres were then suspended in 1, 10 and 100 μg/mL polylysine PBS solutions were agitated in a shaking incubator at 120 rpm for 8 h. The effect of the polylysine concentration on the coating degree of polylysine on the surface of the microsphere skeletons was evaluated using a trypan blue assay as reported [Citation23] with a slight modification. In brief, the microspheres were separated from the polylysine solutions with a 100-mesh sieve. The solutions containing remnant polylysine and standard polylysine solutions were mixed with 1 mg/mL trypan blue solution at appropriate volume ratios. The mixtures were shaken for 12 h at 37 °C, cooled at room temperature, and centrifuged at 10,000 rpm for 30 min to sediment the precipitates composed of polylysine and trypan blue. The absorbance of trypan blue in the supernatants at 580 nm was then measured. Using a calibration curve obtained from trypan blue solutions incubated with polylysine standard solutions, the amounts of polylysine remaining in the coating solutions after the coating procedure were determined. The coating degree of polylysine on the surface of PPM3 was calculated according to the following equation:
(3)
(3)
where WIP is the weight of polylysine initially added to the coating solution and WRP is the weight of polylysine remaining in the supernatants after coating. WPPM denotes the weight of the porous PLGA microspheres used.
To evaluate the stability of the polylysine coated on the surface of the porous microspheres, PPM3 coated using a 1 μg/mL polylysine solution were immersed in distilled water and shaken at 80 rpm at 37 °C for seven days. The concentration of polylysine released from the microspheres in the medium was evaluated on day 1, day 4 and day 7 using the trypan blue assay as described above.
Evaluation of cell loading efficiency and cell loading amount under different cell loading conditions
Different cell loading conditions were examined to maximize the cell loading amount in PPM3. First, the effect of the polylysine coating degree on the cell loading amount was assessed. PPM3 was coated with polylysine as described above using 0.5–100 μg/mL polylysine solutions. The polylysine-coated porous microspheres (5 mg) were placed in a 48-well plate, and UV-irradiated for 30 min. GFP-expressing HEK293 cells (5 × 104) suspended in culture medium (500 μL) were added to the microspheres and incubated for 24 h in the CO2 incubator. Thereafter, the cell loading efficiency and loading amount were evaluated as aforementioned. After optimization of the polylysine coating degree, the influence of cell loading time on the cell loading efficiency and loading amount was evaluated. The above procedure was used, except that the microspheres were coated using a 1 μg/mL polylysine solution and cell loading time from was varied from 2 to 24 h. To investigate the impact of cell concentration on the cell loading efficiency and loading amount, 3 × 104 to 1.5 × 105 cells were added to each well of a 48-well plate and incubated for 9 h in the CO2 incubator. To examine the effect of shaking speed during cell loading on the cell loading efficiency and loading amount, 1.5 × 105 cells and the microspheres were placed in a 48-well plate and shaken at speeds of 80–130 rpm, using a shaker designed for use inside a CO2 incubator (NB101SRC, N-BIOTEK, Bucheon-si, Korea), for 9 h in the CO2 incubator.
Assessment of cell release from porous PLGA microspheres
The polylysine-coated porous microspheres (5 mg) were placed in a 48-well plate, followed by UV irradiation for 30 min. GFP-expressing HEK293 cells (1.5 × 105) suspended in culture medium (500 μL) were added to the microspheres and incubated for 24 h in the CO2 incubator. Unattached cells were removed by rinsing the porous microspheres with PBS, and the cell loading amount in the microspheres was evaluated using WST-1 assay as described above. The cell-loaded microspheres were loaded in a syringe and then injected into each well of a 48-well plate filled with culture medium (500 μL). The 48-well plate was then shaken at 80 rpm in the CO2 incubator. After 6, 12 and 24 h, the microspheres were carefully removed and the amount of cells released from the microspheres and attached to the bottom was assessed using WST-1 assay. The cells released from the microspheres were also observed using an inverted phase contrast microscope (Axiovert 40C, Carl Zeiss, Jena, Germany) and their image was obtained using an image processing software (Toup View, ToupTek Photonics Co., Ltd., Hangzhou, China).
Statistical analysis
All experiments were conducted in triplicate. Means were compared by one-way analysis of variance and Student’s t-test. p < .05 was considered significant.
Results
Control of microsphere pore size by varying preparation conditions
Effect of PLGA concentration on pore size
SEM observations revealed that the pore size of the microspheres was generally increased with decreasing PLGA concentration in chloroform (). In microspheres prepared using 3 g/L and 5 g/L PLGA solutions in the organic phase (PPM1 and 3), most of the pore diameters was larger than the diameter of a single GFP-expressing HEK293 cell in a suspended state (approximately 15 μm). However, the microspheres fabricated with 7 g/L and 10 g/L PLGA solutions showed comparatively small amounts of pores with diameters greater than 15 μm, which might be not proper for efficient cell loading. The PLGA concentration of 5 g/L was chosen for further studies as it was considered to be advantageous to obtain a stronger mechanical strength of the microspheres than 3 g/L PLGA.
Effect of volume ratio of aqueous to organic phase in primary emulsion on pore size
In general, the proportion of pores >15 μm in diameter was increased with increasing the volume fraction of the aqueous phase (). However, at the highest volume ratio of the aqueous to the organic phase, i.e. 40/60, most of the microspheres were found to be partially broken, which could be attributed to their weak mechanical property. Therefore, the volume ratio of 30/70 was selected.
Effect of homogenization speed on pore size
As presented in , the mean pore size and the fraction of pores with a diameter greater than 15 μm were decreased with increasing the homogenization speed. Therefore, the lowest homogenization speed of 3000 rpm was selected to be the most suitable for preparing porous PLGA microspheres with large pore sizes capable of accommodating cells.
Average pore size and porosity of porous PLGA microspheres
The mean pore size and porosity of PPM1, 3, 5, 6 and 9 are shown in . The average pore sizes of PPM1, 3, 5 and 6 were approximately 3, 2, 1 and 0.5 times greater than the diameter of single GFP-expressing HEK293 cell in a suspended state (∼15 μm), respectively. PPM1, 3, 5 and 6 also exhibited comparatively high porosities more than 90%. In the case of PPM9, which was prepared using 100 kDa PLGA for the comparison of mechanical strength with PPM3, it presented a mean pore size and porosity similar to those of PPM3 without statistical significance (p > .05).
Table 2. Average pore size and porosity of porous PLGA microspheres used for cell loading study to establish an optimize pore size for efficient cell loading (PPM1, 3, 5 and 6) along with porous microspheres prepared using PLGA of 100 kDa (PPM9) for comparison of mechanical strength with PPM3.
Loading efficiency, loading amount, and distribution of cells in PLGA microspheres with different pore sizes
The cell loading efficiency and loading amount in the microspheres were the greatest when the mean pore size was approximately two times greater (33.10 μm) than the diameter of the single tested cell in a suspended state (∼15 μm) as illustrated in . The cell loading efficiency and loading amount were significantly lower when the average pore diameter was approximately 0.5, 1 and 3 times that of the single cell. In images of cell-loaded porous PLGA microspheres taken at different depths (), PPM3 with a mean pore diameter two times larger than the single cell diameter carried the greatest amount of the cells, which was in good agreement with the results of the cell loading efficiency and loading amount analysis. The cells were observed in the inner pores of the microspheres when the average pore size was approximately 2–3 times greater than the single cell diameter (PPM1 and 3). However, the cells could not be infiltrated into the pores and remained attached on the microsphere surface in case of the microspheres with a mean pore diameter similar or smaller to the single cell diameter (PPM5 and 6).
Figure 4. Influence of the pore size of PLGA microspheres on (A) cell loading efficiency and (B) cell loading amount in the microspheres. *p < .05 and **p < .01, respectively, as compared with the cell loading efficiency and cell loading amount evaluated from the microspheres with an average pore size of approximately 33 μm.
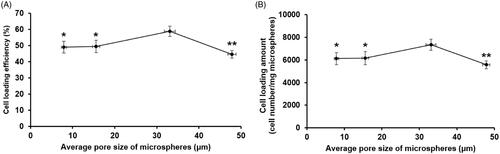
Figure 5. Cell distribution in PPM1, 3, 5 and 6 observed at different depths using a confocal laser scanning microscope. Fifty thousand cells were added to the microspheres (5 mg) contained in the cell culture media (500 μL) in each well of a 48-well plate, and they were then incubated for 24 h in a CO2 incubator.
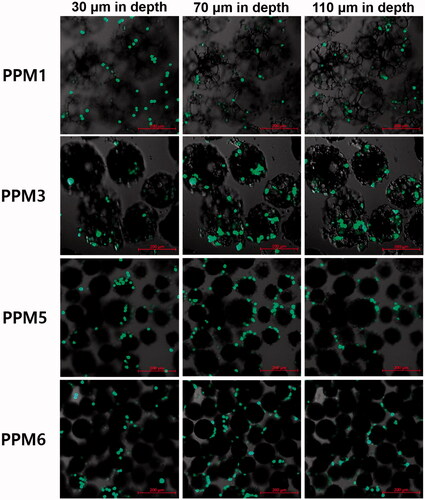
Mechanical strength of porous PLGA microspheres
PPM3 and 9, prepared using PLGA of approximately 30 kDa and 100 kDa, exhibited similar pore sizes and porosities as shown in and . Single PPM3 and 9 presented similar load profiles in the compression test (), indicating that the mechanical property of the microspheres was not considerably different. The load values evaluated from PPM3 and 9 at 100-μm compression were also not statistically different (p > .05) (). Thus, PPM3 and PPM9 had comparable mechanical strength.
Injectability of porous PLGA microspheres
displays the load profiles obtained during expelling PPM3 suspension through syringes with 22- and 26-gauge needles, which was initiated with showing the PBF followed by the DGF stage. For both needles, PBF and Fmax were the same (). The forces measured at the plateau after the PBF stage were not significantly changed and comparatively low. When comparing the morphology of PPM3 before and after injection, noticeable changes were not observed ().
Figure 7. Results of injectability study of PPM3: (A) profiles of load required to expel the PPM suspension from syringes with 22- and 26-gauge needles as a function of time; morphology of PPM3 observed (B) before and after the injectability test through needles of (C) 22 and (D) 26 gauge.
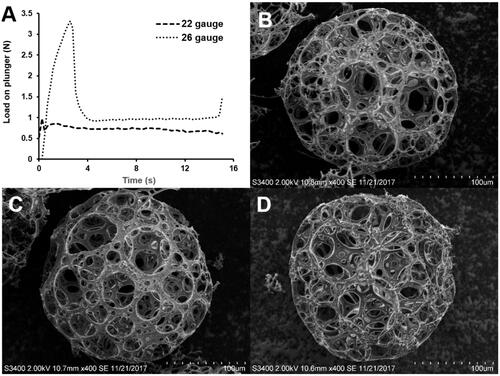
Table 3. Injectability parameters of PPM3: plunger-stopper break loose force (PBF), maximum force (Fmax) and dynamic glide force (DGF) [Citation17].
In vitro degradation of porous PLGA microspheres
PPM3 was gradually degraded throughout the tested period. As illustrated in , the weight of the microspheres was decreased to approximately 20% in 6 weeks, and at the end of the experiment almost all of the microspheres were degraded. The SEM images of the microspheres exhibited that considerable deformations of the microsphere skeletons were occurred from 4 weeks and then the microspheres were broken and disappeared as time progressed ().
Degree and stability of polylysine coating on surface of porous PLGA microspheres
As the porous PLGA microspheres were expected to interact weakly with the cells due to their hydrophobicity and lack of suitable functional groups for promoting the cell attachment [Citation7, Citation24], we selected polylysine, a polycationic peptide, as a cell binding agent to be coated on the surface of the microspheres to enhance the cell binding property of the microspheres. To coat polylysine on the surface of the microspheres at various degrees, the microspheres were shaken in 1–100 μg/mL polylysine solutions. As a result, the mass of polylysine coated on the surface of the microspheres per the mass of the microspheres used was significantly increased with increasing the polylysine concentration (). Thus, it was demonstrated that the coating degree of polylysine on the microsphere surface could be controlled with varying the polylysine concentration in coating solutions.
Figure 9. (A) The effect of polylysine concentration in coating solutions on coating degree of polylysine on surface of porous PLGA microspheres and (B) the stability of the polylysine coating on surface of the microspheres evaluated for seven days. The coating degree of polylysine was denoted as mass of polylysine coated on the surface of the microspheres per mass of the microspheres.
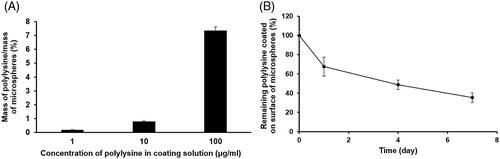
When the stability of the polylysine coating on the surface of the microspheres, which were coated with 1 μg/mL polylysine solution, was assessed for seven days, it was found that the polylysine was gradually released from the surface of the microspheres as presented in .
Cell loading efficiency and loading amount in porous PLGA microspheres assessed under different cell loading conditions
Various cell loading conditions were examined to maximize the cell loading efficiency and loading amount in PPM3. First, the effect of the polylysine coating degree on the cell loading efficiency and loading amount was studied. As illustrated in , PPM3 coated using a 1 μg/mL polylysine solution exhibited the highest cell loading efficiency and cell loading amount. When the polylysine concentration in the coating solutions was higher than 1 μg/mL, the cell loading efficiency and loading amount were generally decreased with increasing the polylysine concentration. In case of the polylysine concentration lower than 1 μg/mL, the cell loading efficiency and loading amount were also decreased. Thus, PPM3 coated using a 1 μg/mL polylysine solution was selected for further studies.
Figure 10. Cell loading efficiency and cell loading amount in porous PLGA microspheres with varying (A, B) concentration of polylysine in coating solutions, (C, D) period of cell loading time, (E, F) number of cells per well and (G, H) shaking speed during the cell loading procedure. *p < .05, **p < .01, ***p < .001 versus no polylysine coating in panels A and B, cell loading time of 2 h in panels C and D, cell loading efficiency for 30,000 cells in panel E, or static condition in panels G and H.
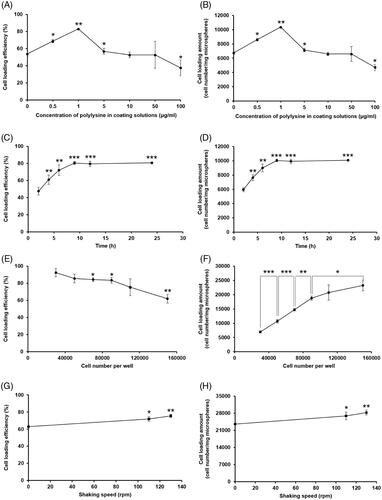
As for the effect of cell loading time, the cell loading efficiency and loading amount in the microspheres were gradually increased as the time progressed up to 9 h (). Thereafter, they did not significantly vary until the end of the experiment. Thus, the minimal period necessary for complete cell attachment to the polylysine-coated PPM3 was determined to be 9 h.
When the cell concentration was increased from 30,000 cells/well to 150,000 cells/well, the cell loading efficiency in the porous PLGA microspheres was considerably decreased (). However, the cell loading amount was increased with increasing the cell concentration, albeit at a gradually decreasing rate (). The CLSM images also revealed that the amount of cells loaded in the microspheres was largely increased with increasing the cell concentration ().
Figure 11. CLSM images of polylysine-coated PPM3 loaded with GFP-expressing HEK293 cells at concentrations of (A) 50,000, (B) 90,000 and (C) 150,000 cells per well. Before the observation, the incubation was conducted for 9 h after adding the cells to the microspheres at the different concentrations.
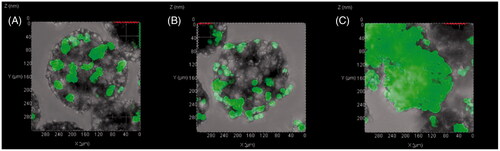
The effect of shaking speed during cell loading was then examined (). The cell loading efficiency and loading amount in the porous microspheres were largely increased under the shaking conditions compared to those assessed without shaking. In addition, the cell loading efficiency and loading amount in the microspheres were greater at a faster shaking speed.
In vitro cell release study
The proportion of cells released from porous PLGA microspheres was gradually increased as a function of time as illustrated in . The images obtained using an inverted phase contrast microscope also revealed that the amount of the cells released from the microspheres and attached to the bottom was increased as time progressed ().
Figure 12. Proportions of GFP-expressing HEK293 cells released from porous PLGA microspheres assessed at 6, 12 and 24 h after injecting the cell-loaded porous microspheres into a 48-well plate. For the cell loading in the microspheres, 1.5 × 105 cells were added to the microspheres (5 mg) contained in the cell culture media (500 μL) in each well of a 48-well plate, and they were then incubated for 24 h.
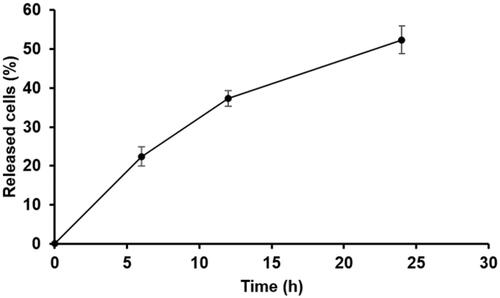
Discussion
One of the primary aims of the present study was to fabricate microspheres with a suitable pore size, porosity and mechanical strength for efficient cell loading using PLGA of a comparatively low MW (∼30 kDa). The reason for using the low MW PLGA to prepare the porous microspheres was because our ultimate goal was to develop porous microparticulate systems that can deliver human corneal endothelial cells efficiently to the corneal endothelium for treatment of corneal endothelial dysfunction. For this purpose, we supposed that the microspheres injected into the anterior chamber of the eye needed to be gradually degraded in several weeks to deliver and integrate the corneal endothelial cells fully to the corneal endothelium. The degradation time of the porous microspheres was tentatively determined based on previous studies on cell therapies for regenerating the corneal endothelium [Citation11–14]. In the previous studies, the structural and functional recovery of the corneal endothelium after injecting the corneal endothelial cells into the anterior chamber has taken generally 4 weeks or more, which might be because the cells were slowly integrated into the host tissue after being delivered to the surface of the corneal endothelium. Therefore, we selected the 30 kDa PLGA [Citation25], which was expected to be degraded in several weeks, for fabrication of the porous microspheres.
The 30 kDa PLGA was not advantageous for producing microspheres with a large pore size, high porosity and good mechanical strength because of its weak mechanical property compared to a high MW PLGA [Citation15]. Therefore, salient experimental conditions of the double emulsion-solvent evaporation method needed to be carefully set to prepare porous microspheres with the required characteristics. The experimental conditions included PLGA concentration in the organic phase [Citation26], volume ratio of the inner aqueous to the organic phase [Citation27], and homogenization speed for preparing the primary emulsion [Citation28], which have been known to affect the pore formation in the microspheres greatly. Through testing diverse preparation conditions of the porous microspheres, we succeeded in producing the microspheres with various pore sizes greater than the diameter of a single GFP-expressing HEK293 cell in suspended state (∼15 μm) at high porosities more than 90% using 30 kDa PLGA.
However, the pores formed in the microspheres prepared with 30 kDa PLGA were initially closed by thin walls composed of the polymer. To open and interconnect the pores for efficient cell loading, we needed to remove the thin walls selectively without causing significant damages to the whole structure of the microspheres. For this, we used ethanolic sodium hydroxide. Sodium hydroxide and ethanol can accelerate the hydrolysis of PLGA and slightly dissolve the polymer, respectively [Citation7]. We thus optimized the concentration of sodium hydroxide in distilled water and the volume ratio of the sodium hydroxide aqueous solution and ethanol, and using the ethanolic sodium hydroxide the pores were successfully opened and interconnected without generating considerable damages to the skeletons of the microspheres.
Among the porous PLGA microspheres prepared, we selected PPM1, 3, 5 and 6, with mean pore diameters of approximately 3, 2, 1 and 0.5 times that of the single tested cell in suspended state (∼15 μm), respectively, and the cells were loaded in the microspheres with different pore sizes to find an optimal pore size for efficient cell loading. The cell loading in the microspheres was evaluated as the cell loading efficiency and cell loading amount. The cell loading efficiency means the fraction of cells loaded in the porous microspheres of the initially added cells and therefore, a high cell loading efficiency implies an efficient use of cells. However, the practical amount of cells loaded in a certain amount of the microspheres could not be obtained by the cell loading efficiency. The information on the amount of cells loaded in the microspheres is indispensable to transplant a necessary number of cells to target tissues. Therefore, we assessed the cell loading amount in the microspheres denoted as the number of cells loaded in the microspheres per the weight of the microspheres. The cell loading amount also needed to be maximized to prevent an unnecessary increase in the usage of the porous microspheres.
From the cell loading study for establishing an optimal pore size for efficient cell loading, it was found that PPM3 with a mean pore diameter of ∼33 μm, approximately two times greater than the single cell diameter in suspended state, exhibited the highest cell loading efficiency and loading amount. This result might be attributed to their proper pore size for the cells to be infiltrated into the inner pores and large surface area of the skeletons on which the cells could be attached, which was revealed by the CLSM images showing the cell distribution in the microspheres. In case of PPM1 with an average pore diameter of ∼48 μm, approximately three times greater than the single cell diameter, they had a relatively small surface area of the skeletons and thereby fewer cells were able to be loaded in the inner pore space although their pore size was large enough for the cells to pass through the pores. As for PPM5 and 6, the cells could not be loaded in the inner pore space and only attached on the surface of the microspheres because the pore sizes were too small. Thus, the optimal pore diameter for efficient cell loading was determined to be ∼33 μm, approximately two times greater than the single cell diameter. Compared with previously investigated porous PLGA microspheres for cell delivery that had average pore diameters of approximately 8–14 μm [Citation1,Citation7], which are smaller than those of human corneal endothelial cells [Citation18], PPM3 with a mean pore diameter of ∼33 μm is expected to be able to load human corneal endothelial cells more efficiently in their pore space.
In addition to the appropriate porous structure for cell loading, an adequate mechanical property was essential for PPM3 to endure physical stresses generated in diverse situations such as handling, cell loading and injection procedures. We first compared the mechanical strength of PPM3 with that of PPM9, which were prepared using PLGA of 100 kDa and exhibited a mean pore size and porosity similar to those of PPM3, to examine the effect of using different MW PLGA on resulting mechanical property of the porous microspheres. Consequently, their mechanical strengths were found to be not significantly different although PPM3 were prepared using a considerably lower MW PLGA (∼30 kDa) than PPM9. The reason for this might be because the higher PLGA concentration (50 g/L) used for preparing PPM3 than PPM9 (35 g/L) compensated for the weak mechanical strength of the 30 kDa PLGA. PPM3 was also able to withstand the physical stresses that occurred during the injection procedure without being appreciably damaged. Thus, PPM3 were considered to have a suitable mechanical property as an injectable cell delivery system.
As an injectable cell delivery system, the porous PLGA microspheres should present a good injectability. However, we concerned about possible clogging of syringe needles by the microspheres during injection. The reason for this was because the microspheres had a relatively large mean particles size of ∼200 μm and thus could block the passage of syringe needles if the microspheres exhibit undesirable behaviours such as aggregation during injection. We therefore conducted the injectability testing of the microspheres. The injectability of the porous microspheres was evaluated with three different parameters such as PBF, Fmax and DGF [Citation21]. The PBF indicates the force needed to initiate the displacement of the syringe plunger, and Fmax means the greatest force measured during the injectability testing. In general, the microspheres are considered to have a good injectability when the PBF and Fmax are the same because it implies that the microspheres can be expelled from syringes without requiring greater forces than the PBF [Citation21]. The Fmax for manual injection also needs to be lower than 50 N in accordance with the regulations of International Organization for Standardization [Citation29]. The DGF denotes the force necessary to expel the contents from syringes after initiating the movement of plunger. Thus, a low DGF signifies a good injectability. The PBF and Fmax measured from PPM3 were the same and also significantly lower than 50 N. The DGF values of PPM3 were also considerably low [Citation21]. Therefore, the injectability of PPM3 was demonstrated to be appropriate.
From the in vitro degradation study of PPM3, as our purpose of using 30 kDa PLGA for preparation of the porous microspheres, most of the microspheres were degraded in weeks. In addition, considerable deformations of the microspheres were observed in 4 weeks, and therefore it is expected that most of cells loaded in the microspheres would be released in 4 weeks. In case of previously reported porous PLGA microspheres for cell delivery, they were fabricated using PLGA of a comparatively higher MW (∼50–80 kDa) [Citation1,Citation7] and supposed to exhibit significantly slower degradation rates than PPM3. Thus, PPM3 are considered to be more advantageous than the existing porous PLGA microspheres for transplantation of human corneal endothelial cells to the corneal endothelium, which may require biodegradation of the microspheres in several weeks.
To maximize the cell loading amount in the porous PLGA microspheres, the cell adhesive property of the microsphere surface needs to be enhanced to facilitate the cell attachment to the microspheres during the cell loading procedure, as PLGA cannot strongly interact with cells due to its hydrophobicity and lack of functional groups promoting the cell adhesion [Citation7,Citation24]. In this study, the microspheres were coated with polylysine, a polycationic peptide widely used as a cell binding agent because polylysine presents a predominantly protonated state at physiological pH owing to its comparatively high pKa value of ∼10 and thereby electrostatically attracts various types of cells [Citation30], regardless of the availability of receptors and complementary ligands. Furthermore, polylysine could be adsorbed to the surface of porous PLGA microspheres without requiring cytotoxic chemical reactions [Citation31]. Before employing polylysine, chitosan, a cationic polysaccharide with a good biocompatibility and biodegradability [Citation32], was examined as a cell adhesive agent to improve the cell binding property of porous PLGA microspheres. However, chitosan-coated porous PLGA microspheres did not show remarkable increases in the cell loading efficiency and cell loading amount (data not shown), which might be attributed to its relatively low pKa value (∼6.5) and consequent low density of positive charges under physiological pH environment [Citation32].
The coating degree of polylysine on the surface of microsphere skeletons needed to be optimized because an excessive or insufficient positive charges exhibited by polylysine may cause toxicity on cells or cannot efficiently attract cells, respectively [Citation33]. For this purpose, PPM3 were coated using solutions containing polylysine at different levels, and it was demonstrated that the polylysine coating degree could be controlled by the polylysine concentration in the coating solution used. In addition, it was found that polylysine coated on the surface of the microspheres was gradually released from the microspheres as time progressed, which may facilitate the release of cells from the porous microspheres. The microspheres coated using 1 μg/mL polylysine solution showed the highest cell loading efficiency and loading amount, which were significantly greater than those of non-coated PPM3. In case of the polylysine concentration in coating solutions lower or higher than 1 μg/mL, the cell loading efficiency and loading amount were largely lowered compared to those evaluated from the microspheres coated using 1 μg/mL polylysine solution.
To further increase the cell loading amount in the porous microspheres, the optimization of cell loading conditions was necessary. For cells to be loaded in the porous carriers, cells first have to settle on and attach to the skeletons, and this process generally takes hours, depending on the cell type and characteristics of the carriers such as the surface property and shape [Citation33]. Therefore, sufficient time is required for complete cell attachment, but the cell loading time also needs to be minimized to save time. We thus evaluated the cell loading efficiency and loading amount in the polylysinized PPM3 as a function of time to establish an optimal cell loading time. Consequently, the optimal cell loading time was determined to be 9 h as the cell loading efficiency and loading amount gradually increased until 9 h, but did not significantly change from 12 h onwards. The concentration of cells is also crucial for enhancing the cell loading amount. While a high cell concentration is needed to fill the pore space fully with cells, cell aggregation can occur at excessively high cell concentrations, preventing cells from infiltrating into the deep pores. Thus, we assessed the cell loading efficiency and loading amount in the polylysinized PPM3 at different cell concentrations. The cell loading efficiency was generally decreased with increasing cell concentration. This result may be ascribed to the limited cell accommodating capacity of the PPM3, judged from CLSM images revealing the cell distribution in the microspheres. As for the cell loading amount, it was increased as a function of cell concentration although the increase rate was gradually decreased, which might be also attributed to the limited pore space. Finally, the effect of shaking speed during cell loading procedure on the cell loading efficiency and loading amount was evaluated. Gentle shaking is known to promote the diffusion of cells into the deep pores of porous carriers [Citation34]. As a result, it was found that the cell loading efficiency and loading amount could be increased with increasing the shaking speed.
For the cells loaded in the porous microspheres to be delivered to a targeted tissue, the cells should be released from the microspheres after injection. From the result of the in vitro cell release study, it was found that the cells could be released from the microspheres and attached to the bottom of a 48-well plate used. It was supposed that the release of cells from the microspheres could be occurred because polylysine coated on the surface of the microspheres was gradually removed from the microspheres as demonstrated by the polylysine coating stability testing and thereby the interaction between the cells and the microspheres became weak as time progressed.
In this study, the porous PLGA microspheres were demonstrated to load cells efficiently in their inner pore space. However, additional functions need to be conferred on the microspheres to enhance the potential of the microspheres as a cell delivery system. In particular, targetability of the porous microspheres to specific tissues is required to transplant therapeutic cells efficiently to the tissues. For this, targeting ligands might be decorated on the surface of the porous microspheres, or materials that can manipulate the movement of the microspheres such as magnetic nanoparticles could be incorporated in the skeletons of the microspheres. In our case, the ultimate goal was to develop porous microparticulate systems that can be selectively moved to the corneal endothelium after being injected into the anterior chamber of the eye and thereby transplant human corneal endothelial cells efficiently to the tissue. To promote the movement of microspheres to the corneal endothelium in the anterior chamber, we currently investigate the magnetic guidance of superparamagnetic iron oxide nanoparticle-loaded porous PLGA microspheres to the corneal endothelium in in vitro and in vivo models along with evaluating the feasibility of selective delivery of human corneal endothelial cells to the corneal endothelium using the microspheres.
Conclusions
Injectable porous microspheres with a suitable porous structure, mechanical strength and biodegradability as a cell delivery system were developed using PLGA of approximately 30 kDa. The pore size and cell adhesive property of the porous PLGA microspheres as well as cell loading conditions were optimized, thereby considerably increasing the cell loading amount in the microspheres. The porous PLGA microspheres generated here hold promise as a cell delivery system for cell therapies to regenerate various types of tissues such as the corneal endothelium.
Disclosure statement
No potential conflict of interest was reported by the authors.
Additional information
Funding
References
- Lee YS, Lim KS, Oh JE, et al. Development of porous PLGA/PEI1.8k biodegradable microspheres for the delivery of mesenchymal stem cells (MSCs). J Control Release. 2015;205:128–133.
- Li YY, Diao HJ, Chik TK, et al. Delivering mesenchymal stem cells in collagen microsphere carriers to rabbit degenerative disc: reduced risk of osteophyte formation. Tissue Eng A. 2014;20:1379–1391.
- Bidarra SJ, Barrias CC, Granja PL. Injectable alginate hydrogels for cell delivery in tissue engineering. Acta Biomater. 2014;10:1646–1662.
- Hamajima S, Hayashi T, Sato Y, et al. Osteoanagenesis after transplantation of bone marrow-derived mesenchymal stem cells using polyvinylidene chloride film as a scaffold. Dent Mater J. 2011;30:707–716.
- Fang J, Zhang Y, Yan S, et al. Poly(l-glutamic acid)/chitosan polyelectrolyte complex porous microspheres as cell microcarriers for cartilage regeneration. Acta Biomater. 2014;10:276–288.
- Liao JF, Wang BY, Huang YX, et al. Injectable alginate hydrogel cross-linked by calcium gluconate loaded porous microspheres for cartilage tissue engineering. ACS Omega. 2017;2:443–454.
- Qutachi O, Vetsch JR, Gill D, et al. Injectable and porous PLGA microspheres that form highly porous scaffolds at body temperature. Acta Biomater. 2014;10:5090–5098.
- Newsholme EA, Leech AR. Functional biochemistry in health and disease. Chichester, West Sussex: Wiley-Blackwell; 2009.
- Yu M, Zhou KC, Zhang FQ, et al. Porous HA microspheres as drug delivery: effects of porosity and pore structure on drug loading and in vitro release. Ceram Int. 2014;40:12617–12621.
- Baeza A, Ruiz-Molina D, Vallet RM. Recent advances in porous nanoparticles for drug delivery in antitumoral applications: inorganic nanoparticles and nanoscale metal-organic frameworks. Expert Opin Drug Deliv. 2017;14:783–796.
- Mimura T, Yamagami S, Yokoo S, et al. Cultured human corneal endothelial cell transplantation with a collagen sheet in a rabbit model. Invest Ophthalmol Vis Sci. 2004;45:2992–2997.
- Shimmura S, Miyashita H, Konomi K, et al. Transplantation of corneal endothelium with Descemet's membrane using a hydroxyethyl methacrylate polymer as a carrier. Br J Ophthalmol. 2005;89:134–137.
- Mimura T, Yokoo S, Araie M, et al. Treatment of rabbit bullous keratopathy with precursors derived from cultured human corneal endothelium. Invest Ophthalmol Vis Sci. 2005;46:3637–3644.
- Hsu W-M, Chen K-H, Lai J-Y, et al. Transplantation of human corneal endothelial cells using functional biomaterials: poly(N-isopropylacrylamide) and gelatin. J Exp Clin Med. 2013;5:56–64.
- Makadia HK, Siegel SJ. Poly lactic-co-glycolic acid (PLGA) as biodegradable controlled drug delivery carrier. Polymers (Basel). 2011;3:1377–1397.
- Yassin MA, Leknes KN, Pedersen TO, et al. Cell seeding density is a critical determinant for copolymer scaffolds-induced bone regeneration. J Biomed Mater Res. 2015;103:3649–3658.
- Miura S, Teramura Y, Iwata H. Encapsulation of islets with ultra-thin polyion complex membrane through poly(ethylene glycol)-phospholipids anchored to cell membrane. Biomaterials. 2006;27:5828–5835.
- Yu WY, Sheridan C, Grierson I, et al. Progenitors for the corneal endothelium and trabecular meshwork: a potential source for personalized stem cell therapy in corneal endothelial diseases and glaucoma. J Biomed Biotechnol. 2011; Article ID 412743.
- Kim TK, Yoon JJ, Lee DS, et al. Gas foamed open porous biodegradable polymeric microspheres. Biomaterials. 2006;27:152–159.
- Bible E, Chau DY, Alexander MR, et al. Attachment of stem cells to scaffold particles for intra-cerebral transplantation. Nat Protoc. 2009;4:1440–1453.
- Cilurzo F, Selmin F, Minghetti P, et al. Injectability evaluation: an open issue. AAPS PharmSciTech. 2011;12:604–609.
- Giannola LI, De Caro V, Giandalia G, et al. Ocular gelling microspheres: in vitro precorneal retention time and drug permeation through reconstituted corneal epithelium. J Ocul Pharmacol Ther. 2008;24:186–196.
- Grotzky A, Manaka Y, Fornera S, et al. Quantification of α-polylysine: a comparison of four UV/vis spectrophotometric methods. Anal Methods. 2010;2:1448–1455.
- Bertram JP, Jay SM, Hynes SR, et al. Functionalized poly(lactic-co-glycolic acid) enhances drug delivery and provides chemical moieties for surface engineering while preserving biocompatibility. Acta Biomater. 2009;5:2860–2871.
- Yeo Y, Park KN. Control of encapsulation efficiency and initial burst in polymeric microparticle systems. Arch Pharm Res. 2004;27:1–12.
- Hong Y, Gao C, Shi Y, et al. Preparation of porous polylactide microspheres by emulsion-solvent evaporation based on solution induced phase separation. Polym Adv Technol. 2005;16:622–627.
- Ikem VO, Menner A, Bismarck A. High-porosity macroporous polymers synthesized from titania-particle-stabilized medium and high internal phase emulsions. Langmuir. 2010;26:8836–8841.
- Seekell RP, Lock AT, Peng YF, et al. Oxygen delivery using engineered microparticles. Proc Natl Acad Sci USA. 2016;113:12380–12385.
- Sterile hypodermic syringes for single use — Part 1: syringes for manual use. The International Organization for Standardization, ISO 7886-1. Geneva, Switzerland; 2017.
- Volodkin D, Ball V, Schaaf P, et al. Complexation of phosphocholine liposomes with polylysine. Stabilization by surface coverage versus aggregation. Biochim Biophys Acta. 2007;1768:280–290.
- Holmes B, Fang X, Zarate A, et al. Enhanced human bone marrow mesenchymal stem cell chondrogenic differentiation in electrospun constructs with carbon nanomaterials. Carbon. 2016;97:1–13.
- Nilsen-Nygaard J, Strand SP, Varum KM, et al. Chitosan: gels and interfacial properties. Polymers. 2015;7:552–579.
- Doagă IO, Savopol T, Neagu M, et al. The kinetics of cell adhesion to solid scaffolds: an experimental and theoretical approach. J Biol Phys. 2008;34:495–509.
- Leferink AM, Hendrikson WJ, Rouwkema J, et al. Increased cell seeding efficiency in bioplotted three-dimensional PEOT/PBT scaffolds. J Tissue Eng Regen Med. 2016;10:679–689.