Abstract
About 40% of the world’s population lives in malaria zones where it presents a challenging health problem. Malaria treatment and prevention have been hindered by drug resistance. Bisphosphonates have been found to be active against Trypanosoma cruzi and Plasmodium falciparum that cause Chaga’s disease and malaria respectively. However, bisphosphonates have a shortcoming of being rapidly removed from the bloodstream through the kidneys before reaching the target sites due to their low molecular weight. In the current study, increased bisphosphonates’ efficacy for malaria treatment was attempted by conjugating bisphosphonates onto carbon nanospheres (CNSs). The synthesis of the target compounds was confirmed by SEM, TEM, EDX, FTIR, Raman and TGA. The target CNSs containing bisphosphonates were evaluated for antimalarial activity against a chloroquine-resistant strain of P. falciparum. From the free bisphosphonates to the conjugates, the results obtained revealed that there were improvements in percentage parasite kill (from –10.71% to 18%, –18.93% to 28.09% and 10.47% to 28.33% for alendronate, pamidronate and neridronate, respectively). The haemolysis assays revealed that the synthesized compound did not have a toxic impact on healthy red blood cells. The results indicate that bisphosphonates conjugated CNSs are said to be promising P. falciparum blood stage inhibitors.
Introduction
The scourge that is inflicted by malaria has seen to researchers developing several formulations that are aimed at targeting the malaria parasite, Plasmodium. These include drugs such as primaquine, artimisinin based combination of therapies, chloroquine, pyrimethamine-sulfadoxine. In light of the evolution of drug resistance of malaria causing parasites as well as the toxicity of the listed drugs, researchers were redirected to focus onto developing effective treatment regimens [Citation1]. Amongst these effective drug formulations are a group of active compounds referred to as bisphosphonates.
Bisphosphonates are organic analogues of inorganic pyrophosphates, yet unlike pyrophosphates, can withstand enzymatic hydrolysis [Citation2,Citation3]. Their structure is comprised of phosphorus to carbon to phosphorus bond (P–C–P) that enables them to withstand enzymatic hydrolysis, whereas pyrophosphates have phosphorus to oxygen to phosphorus bond (P–O–P). Bisphosphonates have shown great promise in treating bone metastasis where their ability to bind onto bone mineral prevents bone resorption and subsequent degradation [Citation4]. Biphosphonates have therefore been established as osteoclast bone resorption inhibitors. Additionally, bisphosphonates have been found to be effective against the in vitro growth of the parasitic protozoa, Trypanosoma cruzi and P. falciparum that cause Chaga’s disease and malaria, respectively. The activity of bisphosphonates against these parasites is made possible by the fact that the enzyme Plasmodium geranylgeranyl diphosphate synthase (PGGPPS) can be inhibited by bisphosphonates [Citation5].
During malaria transmission, an infected female Anopheles mosquito injects an individual with sporozoites. Once the parasites enter the blood stream transform into exoerythrocytic forms (liver stage). After a series of nuclear divisions, sporozoites are transformed to merozoites that enter the blood stream and invade the erythrocytes (erythrocytic stage). Thus, forms of treatment are either targeted to liver stage or erythrocytic stage growth. Earlier studies by Martin et al. [Citation6] regarding malaria, sleeping sickness, Chaga’s disease showed a significant activity of nitrogen containing bisphosphonates against erythrocytic forms. Nitrogen containing bisphosphonates’ activity against malaria was however lower than that of simple alkyl containing bisphosphonates. It is apparent that designing effective nitrogen containing bisphosphonates that specifically targets P. falciparum can significantly increase the activity of bisphosphonates against erythrocytic forms. Although the treatment process using bisphosphonates may appear successful, their effectiveness is compromised by their poor bioavailability due to their low molecular weight, where approximately 3–7% is metabolized by the body and the rest is excreted before reaching the target sites [Citation6]. This reduces their efficacy [Citation7]. However to attain increased efficacy, bisphosphonates are overdosed, which adversely leads to systemic toxicity.
Based on the fact that bisphosphonates are rapidly cleared from the blood stream, addressing this shortcoming could significantly improve the activity of bisphosphonates against the erythrocytic forms. In recent years, the use of nanotechnology has been explored as a way of reducing the shortcomings of current drugs by creating new conjugates with promising and improved pharmacological profiles and modalities [Citation8]. One example of such a proposal is the application of carbon nanomaterials [Citation9,Citation10]. This follows similar examples of alternative technologies (polymers, liposomes), that are now found in the market, which include different drug products indicated for a variety of diseases such as cancer and other infectious diseases [Citation11].
One way to ensure improved bioavailability of bisphosphonates is by providing an all-intact delivery systems that is anticipated to ensure that an increased concentration of the bisphosphonates reaches its target sites by delaying renal clearance. Carbon nanospheres (CNSs) have been suggested and explored as multipurpose innovative carriers for drug delivery [Citation12]. Their unique physicochemical structures enable non-covalent and covalent introduction of several biological moieties and allow for rational design of novel candidate nanoscale constructs for the delivery of a wide range of therapeutic agents [Citation13]. This implies that CNS can also be functionalized for the anchoring of several bioactive moieties designed for novel therapeutic or diagnostics [Citation14].
By conjugation onto CNS, the molecular weight of the bisphosphonates will be increased, thus delaying renal clearance [Citation15]. Herein, CNS synthesis, oxidation and CNS-bisphosphonates conjugation were proven successful by characterization techniques comprising of the SEM, TEM, IR, EDX, Raman and TGA [Citation16]. The bisphosphonate and respective conjugates were then tested for comparative antimalarial activity.
Experimental procedures
Synthesis of carbon nanospheres
Carbon nanospheres were synthesized by direct pyrolysis (subsequent precipitation) of acetylene (C2H2) in an inert atmosphere (argon) via chemical vapour deposition (CVD) method. The synthesis was carried out in a CVD reactor. The CVD configuration is composed of a vertical quartz reactor loaded in a tubular furnace. Argon flow displaced air, thus creating an inert atmosphere in the chamber [Citation17]. The CNSs were grown by diffusion of acetylene within the chamber. When the furnace reached 1000 °C under argon flow, the quartz reactor was fed with acetylene. The flow of both the feed stock (acetylene) and Ar was optimized until the desired size had been attained. After a few minutes, black soot from the direct pyrolysis of acetylene was generated and carried upwards to the upper end of the quartz tube. After 30 min, the soot was collected at the cyclone.
Purification of carbon nanospheres
As prepared, CNSs were refluxed in toluene for 48 h using a Soxhlet extractor to remove aromatic hydrocarbons and amorphous carbon. After 48 h, the CNSs were air dried then oxidized by a wet chemical method [Citation18].
Oxidation of carbon nanospheres
The oxidation method used in this work involved the addition of 0.100 g of purified CNS in a mixture of nitric acid (69%, 9 ml) and sulphuric acid (98%, 3 ml) [Citation19,Citation20]. The mixture was sonicated for 3 min then refluxed for 30 min at 100 °C as illustrated in Scheme 1. The oxidized CNS was filtered, then washed with distilled water and dried under vacuum at 40 °C. Thereafter a back titration was performed to determine the concentration of carboxylic acid (COOH) on the CNS. Oxidized CNS (0.010 g) was added to a NaOH solution (0.0040 N, 25 ml) and stirred for 24 h. The mixture was titrated with a solution of HCl (0.0040 N) to determine excess NaOH in the solution and the concentration of the carboxylates on CNS [Citation21].
Synthesis of bisphosphonates
Three bisphosphonates pamidronate, alendronate and neridronate were synthesized from the corresponding carboxylic acids (Scheme 2). A general method reported by Kieczykowski et al. [Citation22] was employed for the synthesis of the three bisphosphonates. In a typical reaction, 4-aminobutyric acid, 6-aminohexanoic acid or β-alanine (24.2 mmol), phosphorous acid (2.0 g, 24.2 mmol) and 11 ml methane sulphonic acid were put in a 100 ml three-necked round bottom flask fitted with a caustic scrubber. The system was flushed with Ar and heated to 65 °C. PCl3 (4.3 ml, 48.4 mmol) was then added drop wise whilst maintaining the temperature at 65 °C and the reaction was left to reflux for 24 h. The solution was cooled to room temperature and quenched with 90 ml of ice cold water. The pH was adjusted to 4.3 with NaOH solution. A white precipitate was formed and filtered off. The precipitate was air and vacuum dried at 40 °C.
Carbon nanospheres conjugation
Bisphosphonates were conjugated onto CNS by first functionalizing oxidized CNS to acyl chloride entities. Oxidized CNS (0.100 g) was stirred in 150 ml of a 20:1 mixture of thionyl chloride and N, N dimethyl formamide (DMF) at 70 °C for 24 h (Scheme 3).
After acyl chlorination, the CNS were filtered, washed with anhydrous tetrahydrofuran (THF) and dried under vacuum at room temperature for 30 min [Citation23]. 0.00625 g neridronate (dissolved in dry THF) and 0.09375 g glucosamine (dissolved in dry THF to effect dissolution of CNS) were added onto the solid material. The mixture was then left to reflux for 48 h, then filtered and left to dry under vacuum at 40 °C. The dried solid was then analysed to verify the conjugation
In vitro antimalarial assays
SYBR Green I antimalarial assay
A chloroquine-resistant strain of P. falciparum (FCR3) was maintained and used to test the antimalarial properties of the novel compounds. The thin blood smears (fixed with methanol and air dried) were prepared daily in order to examine the culture, check the growth conditions and determine the percentage parasitaemia and stage. The incomplete hypoxanthine positive culture medium was prepared as previously reported [Citation24,Citation25]. After exposure to the CNS bisphosphonate conjugates, the % parasite growth was measured using SYBR Green I to stain the nucleic acids.
Haemolysis assay
To establish whether bisphosphonates CNS conjugates have a toxic effect on red blood cells and to account for a false positive inhibitory activity, the method as described by Hayat et al. was used [Citation26]. With the assistance of a phlebotomy technician, blood was drawn from healthy volunteers into anti-coagulant blood tubes that contain citrate phosphate dextrose-1 and samples no older than three days were used to ensure optimal red blood cell membrane integrity. The plate was incubated at room temperature (approximately 25 °C) for 10 min and the absorbance was then read at 412 nm on the Lab systems iEMS Reader MF.
Results and discussion
Synthesis of carbon nanospheres
The lower molecular weight and lower bioavailability of bisphosphonates have led researchers to investigate on the conjugation of the bisphosphonates onto drug delivery systems as a way to curb these short comings. Examples of drug delivery systems included nanoparticles, liposomes and microspheres. For effective passive targeting of drug delivery systems, the size should be between 10 and 100 nm [Citation27]. If they are smaller than 10 nm, they are eliminated by the liver and if they are larger than 100 nm they are eliminated by the kidneys. Carbon nanomaterials have shown a great promise as potential drug delivery vehicles. The size of CNSs synthesized via pyrolysis method was controlled by gas flow rates. As Ar gas flow rate increased, the size of the spheres became smaller. The ideal CNS size, that is, 100 nm was attained after several trials of optimizing acetylene and Ar flow. When prepared, CNS contained some impurities, such as amorphous carbon, that would compromise their biological applications. It was therefore crucial that these impurities were removed. In the current project, CNSs were purified by extraction using a Soxhlet extractor in the presence of toluene. Purified CNS were oxidized using a mixture of concentrated nitric acid and sulphuric acid (3:1), which introduced hydroxyl and carboxylic groups on the surface of CNS. The concentration of carboxyl groups was determined by back titration. To account for the hydroxyl of the carboxyl group being a poor leaving group, the oxidized CNS were acylated and the carboxylic groups converted to acyl chloride which is a better leaving group. In the search of a solubilizing functionalized CNS, solubilizing moieties such as DEEA, DEP, EA and glucosamine were used. Only glucosamine was found to impart a good solubility to CNS. After determining the optimal solubilizing group, CNS conjugates were prepared using a one pot method. Both bisphosphonate and glucosamine dissolved in DMF, were added to solid CNS and refluxed for a period of time. This process yielded the target conjugates (Scheme 4). Characterization techniques used to ascertain the successful synthesis of the CNSs included: SEM, TEM, Raman, FTIR and the TGA.
Scanning electron microscopy
Drug delivery systems come in different forms and shapes, with the spherical drug delivery systems being preferred over their counterparts. Spherical drug delivery systems are preferred because they have a simpler geometry, a consistent effective size and a uniform surface chemistry. In the current study, spherical structures were observed in SEM images of as prepared CNS depicted in , thus confirming successful synthesis of CNS [Citation28]. Hence 100 nm, which is ideal for this work, was attained at 80 ml/min (Ar) and 20 ml/min (C2H2) as shown in .
Table 1. Carbon nanosphere size distribution at different C2H2 and Ar flow rates.
Transmission electron microscopy
TEM images of as prepared CNSs showed CNSs as well as other smaller spherical structures (shown by the red arrow in ). The smaller spherical structures present in the as prepared CNS could be attributed to hydrocarbons and amorphous carbon [Citation29]. These hydrocarbons and amorphous carbon were removed during the Soxhlet extraction of the as prepared CNS in toluene to leave only spherical pristine CNS, as shown in . TEM image of the soot analysed showed that their diameter distribution range lies between 80 nm and 120 nm (as seen in the histogram in ). It can be seen in this graph that the desired size is present in high amounts (28%) compared to the non-desired sizes.
Raman spectroscopy
The Raman spectra () for as prepared CNS depicted two bands at 1322 cm−1 and 1592 cm−1 that are designated to the D and the G bands of graphite, respectively. The two bands are attributed to the defects and disorder induced modes, thus confirming that CNSs were successfully synthesized. The intensity ratio of the as prepared CNS, ID/IG=1.18, thus reflected a low degree of graphitization and the presence of disordered carbon [Citation30].
Fourier-transform infrared spectroscopy
The FTIR spectrum for as prepared CNSs depicted the presence of a hydroxyl group at 3440 cm−1. The hydroxyl group indicated that water was adsorbed on the surface of the CNSs. The presence of the hydroxyl groups might have also resulted from the CNSs coming into contact with the ambient air. The absorption peaks at 2917, 1728, 1629, 1458, 1098 and 800 cm−1 corresponded to C–H stretch, C=O stretch, C=C stretch, C–H stretch, CO and C–H stretch, respectively. The absorption peaks mentioned above are typical of CNSs [Citation31].
Thermogravimetric analysis
When CNSs are heated in air, the sample is stable below 600 °C. Maximum weight loss for as prepared CNS as depicted by was recorded at 630 °C and is clearly shown by the derivative weight loss versus temperature graph in . This maximum weight loss is attributed to the decomposition of the carbon skeleton and is indicative of lattice defects that enable oxygen to easily permeate the spheres. The carbon skeleton confirms that the CNS was successfully synthesized [Citation32].
Purification of as prepared carbon nanospheres
As prepared CNS contained some impurities, such as amorphous carbon, that would otherwise compromise their biological applications as these impurities may induce cytotoxicity. It is therefore crucial that these impurities are removed. In the current work, CNS were purified by extraction using a Soxhlet extractor in the presence of toluene [Citation33,Citation34]. These hydrocarbons and amorphous carbon were removed during the Soxhlet extraction of the as prepared CNS in toluene to leave only spherical pristine of CNS as shown in .
Oxidation of purified carbon nanospheres
As prepared CNS tend to be hydrophobic, it is therefore necessary to ensure that they are functionalized to render them hydrophilic. Functionalization also makes the CNS to have a lower toxicity. Oxidation allows for further surface modification to enhance conjugation of biological moieties [Citation35]. Several oxidation methods with distinct reaction conditions have been investigated and were found to yield different results. In the current work, a mixture of nitric acid and sulphuric acid treatment under reflux conditions was used to endow the CNS with carboxylic and hydroxylic acid groups. In addition to these functional groups, defects were also increased on the walls of the CNS as induced by the harsh acidic conditions. Hence, in future works, only nitric acid will be used as an oxidation agent.
Several characterization techniques were used to confirm successful oxidation which included the SEM, TEM, Raman and the TGA.
Scanning electron microscopy
SEM image in shows that the spherical structure of the CNS was maintained even after oxidation. This signifies that the CNSs are ideal drug delivery systems [Citation36].
Transmission electron microscopy
The spherical structure of the CNS was maintained for oxidized CNS () and conjugated CNS () despite the CNS having to be subjected to harsh conditions. Purified CNSs were oxidized using a mixture of concentrated nitric acid and sulphuric acid (3:1). This process introduced hydroxyl and carboxylic groups on the surface of CNS. The concentration of carboxyl groups was determined by back titration. As the hydroxyl of the carboxyl group is a poor leaving group, the oxidized CNS were acylated and the carboxylic groups converted to acyl chloride which is a better leaving group [Citation28,Citation37].
Raman spectroscopy
The Raman spectra for oxidized CNS showed an increase in the D band intensity which is attributed to the defects induced by the acid mixture on the CNS surface. The ID/IG band increased to 1.27 after functionalization. Additionally, there is a shift of the D band towards the right (1339 cm−1) to further confirm a change in the structural bonding as effected by oxidation [Citation38].
Fourier-transform infrared spectroscopy
There was no significant difference between the peaks depicted by the spectra of the as prepared CNS and the FTIR peaks of the oxidized CNS. However, there was an increased intensity of some of the peaks of oxidized CNSs and these include the O–H peak, C=O stretch and C–O stretch respectively as portrayed by IR spectra in [Citation39]. The increased intensity can be attributed to the successful incorporation of carboxylic acid (COOH) and hydroxyl (OH) functional groups after oxidation. The increased intensity correlated with the concentration of the acidic sites as observed after back titration. Results obtained from back titration technique using NaOH alluded to the increased intensity as demonstrated by an increase in the concentration of acidic surface sites from 2.76 mmol/g on the as prepared CNS to 5.08 mmol/g on the oxidized CNS.
Thermogravimetric analysis
Decomposition at 588 °C and 786 °C was observed for oxidized CNS. Weight losses at the respective temperatures can be attributed to the carbon framework and hydroxyl and carboxylic acid group decomposition, thus confirming that oxidation had occurred [Citation37,Citation40].
Solubilization of oxidized carbon nanospheres
Carbon nanospheres are macromolecules that constitute of a thousand carbon atoms in an electronically aromatic system, therefore, they tend to be insoluble in all solvents. This is an unfavourable condition particularly when the CNSs are to be utilized for biomedical application. Solubilizing the CNS renders them to be biocompatible [Citation41]. This translates to that once bisphosphonates have reached their target site the CNS can be excreted through the kidneys, thus preventing accumulation of the CNS in the blood system which may lead to cytotoxicity.
In the search for solubilizing the functionalized CNS, solubilizing moieties such as DEEA, DEP, EA and glucosamine were used. Only glucosamine was found to impact a good solubility to CNS. After determination of the better solubilizing group, CNS conjugates were prepared using a one pot method. Both bisphosphonate and glucosamine dissolved in DMF were added to solid CNS and refluxed for a period of time. This process afforded the target conjugates. CNS conjugated GLA was deemed soluble in water, based on the fact that it did not contain any precipitate upon prolonged standing [Citation42]. Solubility was measured as a function of temperature. As expected, solubility increased with increase in temperature as seen in . As more CNS–GLA conjugate was dissolved, the solubility limit was reached.
Bisphosphonates synthesis
There are two major methods for bisphosphonates synthesis, that is, the direct method and indirect method. In the direct synthesis method, a carboxylic acid or acid halide reacts with phosphorous acid or phosphorous trichloride, followed by hydrolysis. In the indirect method, an initial Arbuzov reaction gives an α-ketophosphonate from an acid halide and a dialkyl phosphite followed by a second step whereby dialkyl phosphate is added to the carbonyl group of the α ketophosphonate to produce the bisphosphonate [Citation43]. The direct method was applied in the current work, with the carboxylic acids being β-alanine, 4-amino butyric acid and 6-amino caproic acid for pamidronate, alendronate and neridronate, respectively. Characterization techniques used to confirm the synthesized bisphosphonates included the nuclear magnetic resonance (NMR) and Fourier-transform infrared spectroscopy (FTIR).
Nuclear magnetic resonance spectroscopy
The NMR spectroscopy was used to ascertain the successful synthesis of BPs (pamidronate, alendronate and neridronate). The target BPs were confirmed by the characteristic NMR shifts. In the 31P NMR of pamidronate, alendronate and neridronate, it is noteworthy to mention that the two phosphorous atoms appeared as a single signal at a range of δ 16.99, 18.54 and 18.31 ppm, respectively. This is due to the two atoms being magnetically equivalent as they were bonded to the same carbon atom [Citation44]. 13C NMR further suggested that the synthesis of the three bisphosphonates salts was a success as proven by the appearance of the carbon triplet at a range of δ 74.67–74.08 ppm (JPC 132.5–160 Hz) [Citation45]. This is in support with the fact that the proposed structures consisted of two phosphonates groups attached to the same carbon (P-COH-P). Below is a summary of NMR shift for the three bisphosphonates namely: pamidronate, alendronate and neridronate, respectively.
Pamidronate (β-alanine): yield of 5.48 g, 35% 1HNMR (δ 3.185 (t, NH2CH2 2 H 3JH-H=8.5 Hz) δ 2.151 (m, CH2CH2, 2 H, H-2), 13C NMR (δ 74.67 (t, 2JC-P=137) δ 36.17, δ 32.80 (t, 2JC-P=7.6), 31P NMR (D2O, 300 MHz) 16.99 ppm.
Alandronate (4-aminobutyric acid): yield of 5.1 g (85%); H NMR (D2O, 300 MHz) δ 3.416 (t, NH2CH2, 2H) JH-H=7.4 Hz, δ 0.961 (t, 4 H, JH-H=7.2 Hz), 13C NMR (300 MHz) σ 74.35 (t2, JC-P=160), 38.48, 33.79, 23.88 (t2, JC-P=6.36), P NMR (D2O, 300 MHz) 18.54 ppm.
Neridronate (6-amino caproic acid): yield of 3.99 g, 42% 1H NMR σ 2.172 (t, NH2CH2, 2H, JH-H=6.3 Hz), σ 1.85–1.75 (2H, m), σ 1.46–1.37 (4H, m), σ 1.25–1.13 (2H, m), 13C NMR σ 74.08 (t, JCP = 132.5), 48.33, 39.26, 33.04, 27.22, 20.51 (t, JC-Pn=6.3), 31P NMR (D2O, 300 MHz) 18.31 ppm.
Bisphosphonates conjugation
Bisphosphonates are a class of bioactive compounds which have a high affinity for bone tissue.
However, their efficiency is compromised by the rapid clearance from the blood so that only about 1% of the active compound reaches target organs [Citation46]. One way to address the shortcoming of low molecular weight is to link bisphosphonates covalently or non-covalently onto ideal drug delivery systems. In the current work, bisphosphonates were conjugated covalently onto CNSs. Several characterization techniques were used to ascertain the success of conjugation.
These included the SEM, TEM, Raman, FTIR and the TGA.
Scanning electron microscopy
The spherical shape of the CNSs as shown by the SEM in was maintained even after bisphosphonates conjugation. This signifies that the CNS was an ideal drug delivery system that can withstand harsh acidic conditions [Citation47].
Transmission electron microscopy
TEM images in also showed that the structural integrity of the CNS was left unaltered by harsh condition thus rendering the CNS as ideal drug delivery vehicles [Citation48].
Raman spectroscopy
There was a shift of the D band of the bisphosphonates conjugated CNS towards the left, relative to the D band of the oxidized CNS (1313–1324 cm−1) as depicted by the Raman spectra in . This confirms that there was functionalization that occurred after bisphosphonates conjugation where ID/IG was found to be 1.17 [Citation48].
Fourier-transform infrared spectroscopy
There was an appearance of phosphoryl bonds (P=O and P–O) at 1027.18 cm−1 and 920 cm−1 in the FTIR spectra confirming that bisphosphonates conjugation was a success [Citation47]. The spectrum of bisphosphonates conjugation of CNS further depicted the presence of amide bonds (N–H and N–C) at 1432 cm−1 and 1389 cm−1, respectively. This further proved that the bisphosphonates were linked via an amide linker.
Thermogravimetric analysis
The TGA plot for BPs conjugated CNS in depicts a weight loss at around 254 °C. This temperature correlated with bisphosphonates’ melting point. The decomposition of bisphosphonates was further confirmed by the FTIR spectra in that portrays a phosphoryl (P=O) peak at 1286 cm−1. 433 °C depicted the decomposition of an amide group as confirmed by the FTIR peak at 2337 cm−1. Weight loss at 540 °C also depicted the decomposition of the carbon framework (C=C) as shown by the peak at 2336 cm−1 [Citation49].
SYBR Green I antimalarial assay
The SYBR Green I antimalarial assay was used to determine the in vitro antimalarial activity of bisphosphonates conjugated CNS by monitoring the change in parasitaemia compared to the untreated control. A substantial decrease in % parasite growth was observed when the malaria parasites were exposed to the bisphosphonate conjugates compared to the free bisphosphonates ().
Figure 9. Parasite growth observed when P. falciparum was exposed to 50 µg/ml of free bisphosphonates and bisphosphonate conjugated CNS.
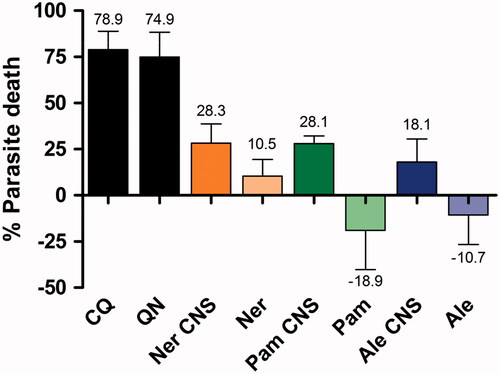
The percentage parasite death increased when the free drug was conjugated to CNS. The alendronate CNS conjugate induced 18.1% parasite death in comparison to the free drug alendronate where latter did not kill the parasite, but appeared to stimulate parasite growth by 10.71%. A similar trend was observed with pamidronate, where the pamidronate CNS conjugate accounted for 28.1% parasite death, and the free pamidronate stimulated 18.9% parasite growth (). As such, it was concluded that the free drugs were inactive in inhibiting parasite growth. This lack of inhibitory activity was also noted by Ghosh et al. for several nitrogen-containing bisphosphonates including risedronate or pamidronate [Citation50]. It has been noted before that the small, very hydrophilic bisphosphonates do not penetrate the red blood cell [Citation51], at least in part, due to the poor transport properties attributed to the presence of a charged nitrogen atom in the alkyl/aryl side chain [Citation1,Citation50]. In comparison, the free drug of neridronate inhibited 10.5% parasite growth, but this was 2.7-fold less effective than the neridronate CNS conjugate (). As such, of the three free bisphosphonates evaluated, neridronate was the most effective. It could be proposed that the CNS conjugate allowed for an increased concentration of neridronate to accumulate within the parasite and erythrocytic host [Citation1,Citation50].
The more lipophilic BPs have been shown to accumulate in acidocalcisomes to elicit its mechanism of action by inhibiting the isoprenoid pathway [Citation1]. These nitrogen-containing BPs have been proposed to inhibit the Plasmodium enzymes in this pathway, such as geranylgeranyl diphosphate synthase (GGPPS), farnesylpyrophosphate (FPP) synthase [Citation1,Citation50] or the prenyl diphosphate synthases. It has also been shown that although several nitrogen-containing bisphosphonates were potent FPP synthase inhibitors, such as pamidronate, they possessed little or no in vitro intracellular activity due to their poor ability to permeate through the four cellular membranes of the red blood cell and parasite to accumulate in the parasitic food vacuole [Citation50].
The increased percentage death of P. falciparum signified an improved BPs’ efficacy after conjugation onto CNS compared to a free drug. This could be attributed to the temporal protection of BPs from premature enzymatic degradation or facilitated intra-erythrocytic entry to be able to concentrate in the endocytotic or parasitic food vacuole [Citation16]. Neridronate showed the highest percentage kill compared to alendronate and pamidronate; which could be attributed to neridronates’ structure, since it contains five carbons that cause little activity hindrance due to the carbon nanomaterials.
Haemolysis assay
The haemolysis assay was used to determine whether the BPs and CNS conjugates had a toxic effect on red blood cells by compromising the red blood cell-host membrane integrity. The lack of haemolysis as indicated by the low haemoglobin in the supernatant (0.29–1.43%) indicated that the CNS and BPs did not affect the integrity and permeability of the red blood cell membrane of the human host, as compared to quinine (1.53%). This observation is supported by that of Felix and Fleisch, who noted that small, very hydrophilic bisphosphonates, such as etidronate and clodronate, do not readily move across red cell membranes [Citation51]. This lack of haemolysis also indicates that there was a direct effect by the BPs and CNS on the intra-erythrocytic parasite and not via host cell destruction. As such, this haemolytic assay demonstrated that the conjugates had negligible in vitro toxicity. CNS are therefore said to be promising as a drug delivery system for increased treatment efficacy and minimal side effects to normal tissue [Citation45].
Conclusions
Carbon nanospheres were successfully synthesized via the direct pyrolysis method and the structures confirmed by the SEM, TEM, FTIR, Raman, EDX and the TGA. Functionalization (oxidation and bisphosphonates conjugation) of CNS was ascertained through: back titration, FTIR, EDX, Raman and TGA. Alendronate, neridronate and pamidronate were synthesized via the direct pathway and then characterized using the NMR. Bisphosphonates were then conjugated onto CNS as potential drug delivery systems. Carbon nanospheres were rendered soluble by reacting them with glucose amine. There was an increased percentage kill of Plasmodium falciparum after conjugation of bisphosphonates onto CNS, thus signifying increased bisphosphonates efficacy. This translates to that the efficiency of bisphosphonates is increased when conjugated to the CNS compared to a free drug.
Disclosure statement
The authors confirm that there is no conflict of interest.
Additional information
Funding
References
- Singh AP, Zhang Y, No J-H, et al. Lipophilic bisphosphonates are potent inhibitors of Plasmodium liver-stage growth. Antimicrob Agents Chemother. 2010;54:2987–2993.
- Singh T, Kaur V, Kumar M, et al. The critical role of bisphosphonates to target bone cancer metastasis: an overview. J Drug Target. 2015;23:1–15.
- Le Goff B, Heymann D. Pharmacodynamics of bisphosphonates in arthritis. Expert Rev Clin Pharmacol. 2011;4:633–641.
- Abdou WM, Shaddy AA. The development of bisphosphonates for therapeutic uses, and bisphosphonate structure–activity consideration. ARKIVOC. 2008;2009:143.
- Mouridsen HT, Lønning P, Beckmann MW, et al. Use of aromatase inhibitors and bisphosphonates as an anticancer therapy in postmenopausal breast cancer. Expert Rev Anticancer Ther. 2010;10:1825–1836.
- Martin MB, Grimley JS, Lewis JC, et al. Bisphosphonates inhibit the growth of Trypanosoma brucei, Trypanosoma cruzi, Leishmania donovani, Toxoplasma gondii, and Plasmodium falciparum: a potential route to chemotherapy. J Med Chem. 2001;44:909–916.
- Isla D, Afonso R, Bosch-Barrera J, et al. Zoledronic acid in lung cancer with bone metastases: a review. Expert Rev Anticancer Ther. 2013;13:421–426.
- Parveen S, Misra R, Sahoo SK. Nanoparticles: a boon to drug delivery, therapeutics, diagnostics and imaging. Nanomed: Nanotechnol Biol Med. 2012;8:147–166.
- Yoosefian M, Rahmanifar E, Etminan N. Nanocarrier for levodopa Parkinson therapeutic drug; comprehensive benserazide analysis. Artif Cells Nanomed Biotechnol. 2018;1–13. Doi:10.1080/21691401.2018.1430583
- Farvadi F, Tamaddon A, Sobhani Z, et al. Polyionic complex of single-walled carbon nanotubes and PEG-grafted-hyperbranched polyethyleneimine (PEG-PEI-SWNT) for an improved doxorubicin loading and delivery: development and in vitro characterization. Artif Cells Nanomed Biotechnol. 2017;45:855–863.
- te Kulve H, Rip A. Economic and societal dimensions of nanotechnology-enabled drug delivery. Expert Opin Drug Deliv. 2013;10:611–622.
- Fazil M, Baboota S, Sahni JK, et al. Bisphosphonates: therapeutics potential and recent advances in drug delivery. Drug Deliv. 2015;22:1–9.
- Haley B, Frenkel E. Nanoparticles for drug delivery in cancer treatment. Urol Oncol Semin O I. 2008;26(1):57–64.
- Tessonnier J-P, Rosenthal D, Hansen TW, et al. Analysis of the structure and chemical properties of some commercial carbon nanostructures. Carbon. 2009;47:1779–1798.
- Karimi M, Solati N, Ghasemi A, et al. Carbon nanotubes part II: a remarkable carrier for drug and gene delivery. Expert Opin Drug Deliv. 2015;12:1089–1105.
- Wang J, Hu Z, Xu J, et al. Therapeutic applications of low-toxicity spherical nanocarbon materials. NPG Asia Mater. 2014;6:e84.
- MacKenzie K, Dunens O, Harris AT. A review of carbon nanotube purification by microwave assisted acid digestion. Sep Purif Technol. 2009;66:209–222.
- Mhlanga S, Coville N, Iyuke S, et al. Controlled syntheses of carbon spheres in a swirled floating catalytic chemical vapour deposition vertical reactor. J Exp Nanosci. 2010;5:40–51.
- Rahmam S, Mohamed N, Sufian S. Effect of acid treatment on the multiwalled carbon nanotubes. Mater Res Innovat. 2014;18:S6-196–S6-9.
- Sharma S, Mehra NK, Jain K, et al. Effect of functionalization on drug delivery potential of carbon nanotubes. Artif Cells Nanomed Biotechnol. 2016;44:1851–1860.
- Sadegh Vishkaei M, Yunus R, Mohd Salleh MA, et al. Innovative method to produce high-purity graphitic carbon nanospheres. Fullerenes Nanotubes Carbon Nanostruct. 2012;20:109–118.
- Kieczykowski GR, Jobson RB, Melillo DG, et al. Preparation of (4-amino-1-hydroxybutylidene) bisphosphonic acid sodium salt, MK-217 (alendronate sodium). An improved procedure for the preparation of 1-hydroxy-1, 1-bisphosphonic acids. J Org Chem. 1995;60:8310–8312.
- Singh P, Campidelli S, Giordani S, et al. Organic functionalisation and characterisation of single-walled carbon nanotubes. Chem Soc Rev. 2009;38:2214–2230.
- Lambros C, Vanderberg JP. Synchronization of Plasmodium falciparum erythrocytic stages in culture. J Parasitol. 1979;65:418–420.
- Johnson JD, Dennull RA, Gerena L, et al. Assessment and continued validation of the malaria SYBR Green I-based fluorescence assay for use in malaria drug screening. Antimicrob Agents Chemother. 2007;51:1926–1933.
- Hayat F, Moseley E, Salahuddin A, et al. Antiprotozoal activity of chloroquinoline based chalcones. Eur J Med Chem. 2011;46:1897–1905.
- Prakash S, Malhotra M, Shao W, et al. Polymeric nanohybrids and functionalized carbon nanotubes as drug delivery carriers for cancer therapy. Adv Drug Deliv Rev. 2011;63:1340–1351.
- Sobkowicz MJ, Dorgan JR, Gneshin KW, et al. Controlled dispersion of carbon nanospheres through surface functionalization. Carbon. 2009;47:622–628.
- Nieto-Márquez A, Romero R, Romero A, et al. Carbon nanospheres: synthesis, physicochemical properties and applications. J Mater Chem. 2011;21:1664–1672.
- Hou P-X, Liu C, Cheng H-M. Purification of carbon nanotubes. Carbon. 2008;46:2003–2025.
- Mirershadi S, Mortazavi S, Reyhani A, et al. Effective condition for purification of multi-walled carbon nanotubes by nitric acid. Synth React Inorg Metal-Organ Nano-Met Chem. 2009;39:204–208.
- Martincic M, Tobias G. Filled carbon nanotubes in biomedical imaging and drug delivery. Expert Opin Drug Deliv. 2015;12:563–581.
- Vittorio O, Raffa V, Cuschieri A. Influence of purity and surface oxidation on cytotoxicity of multiwalled carbon nanotubes with human neuroblastoma cells. Nanomed: Nanotechnol Biol Med. 2009;5:424–431.
- Chomoucka J, Drbohlavova J, Huska D, et al. Magnetic nanoparticles and targeted drug delivering. Pharmacol Res. 2010;62:144–149.
- кирпач кО, пОлункін ЄВ. The modification of carbon nanospheres with oxygen-containing groups by the oxidation in nitric acid. Naukovi Visti NTUU KPI. 2015;6:102–108.
- Lecouvey M, Leroux Y. Synthesis of 1‐hydroxy‐1, 1‐bisphosphonates. Heteroatom Chem. 2000;11:556–561.
- Scheibe B, Borowiak-Palen E, Kalenczuk RJ. Oxidation and reduction of multiwalled carbon nanotubes—preparation and characterization. Mater Charact. 2010;61:185–191.
- Schierz A, Zänker H. Aqueous suspensions of carbon nanotubes: surface oxidation, colloidal stability and uranium sorption. Environ Pollut. 2009;157:1088–1094.
- Marega R, Accorsi G, Meneghetti M, et al. Cap removal and shortening of double-walled and very-thin multi-walled carbon nanotubes under mild oxidative conditions. Carbon. 2009;47:675–682.
- Aghabozorg HR, Safari KS, Rashidi AM. Solubility of functionalized carbon nanotubes in different solvents. J Appl Chem Res. 2010;3(12):29–33
- Liu Z, Sun X, Nakayama-Ratchford N, et al. Supramolecular chemistry on water-soluble carbon nanotubes for drug loading and delivery. ACS Nano. 2007;1:50–56.
- Alanne A-L, Hyvönen H, Lahtinen M, et al. Systematic study of the physicochemical properties of a homologous series of aminobisphosphonates. Molecules. 2012;17:10928–10945.
- Zhu M, Shi J, He Q, et al. An emulsification–solvent evaporation route to mesoporous bioactive glass microspheres for bisphosphonate drug delivery. J Mater Sci. 2012;47:2256–2263.
- Mukaya H, Neuse E, Van Zyl R, et al. Synthesis and preliminary bio-evaluation of polyaspartamide Co-conjugates of p-amino-salicylic acid chelated platinum (II) and ferrocene complexes. J Inorg Organomet Polym. 2015;25:367–375.
- Vashist SK, Zheng D, Pastorin G, et al. Delivery of drugs and biomolecules using carbon nanotubes. Carbon. 2011;49:4077–4097.
- Mukaya HE, Mbianda XY. Macromolecular co-conjugate of ferrocene and bisphosphonate: synthesis, characterization and kinetic drug release study. J Inorg Organomet Polym. 2015;25:411–418.
- Mkhabela V, Mishra A, Mbianda X. Thermal and mechanical properties of phosphorylated multiwalled carbon nanotube/polyvinyl chloride composites. Carbon. 2011;49:610–617.
- Zhang T, Xu M, He L, et al. Synthesis, characterization and cytotoxicity of phosphoryl choline-grafted water-soluble carbon nanotubes. Carbon. 2008;46:1782–1791.
- Jones CF, Grainger DW. In vitro assessments of nanomaterial toxicity. Adv Drug Deliv Rev. 2009;61:438–456.
- Ghosh S, Chan JM, Lea CR, et al. Effects of bisphosphonates on the growth of Entamoeba histolytica and Plasmodium species in vitro and in vivo. J Med Chem. 2004;47:175–187.
- Felix R, Fleisch H. The effect of pyrophosphate and diphosphonates on calcium transport in red cells. Experientia. 1977;33:1003–1005.