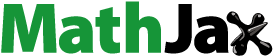
Abstract
Prostate cancer is the most common non-skin cancer among men. Though statins are mainly used as antihyperlipidemic drugs, many studies have reported their proapoptotic and antimetastatic activities on prostate cancer. However, the poor solubility and insufficient delivery of statins in tumor site limit their anticancer activity. The present study introduces an efficient hybrid drug delivery system for the treatment of prostate cancer. The system involves the chemical conjugation of Simvastatin (SMV), a statin compound, to acid-terminated poly(D, L-lactic-co-glycolic acid), PLGA chains followed by its conversion into nanoparticles (NPs), with in situ physical incorporation of more SMV and superparamagnetic iron oxide nanoparticles (SPIONS) into the PLGA NPs. The PLGA-based hybrid nanocarrier system has been designed in such a way to evade the low bioavailability of SMV, confer sustained release of both encapsulated and chemically conjugated SMV, as well as enhancing the anti-cancer effect of the formula via the magnetic targeting with the aid of the encapsulated SPIONS. Magnetism, morphological and physicochemical characterizations, as well as in-vitro release studies were performed. Besides, cytotoxicity on human prostate cancer cell line (PC-3) was evaluated using MTT assay, cell cycle arrest analysis, annexin V/propidium iodide apoptosis assay and ELISA immunoassay for apoptotic enzyme. Optimum PLGA-based hybrid nanocarrier significantly improved the SMV anticancer activity against human prostate cancer cell line through both apoptosis mechanism and retardation of G2-M phase of cell cycle. Also, the up-regulation of the Caspase 3 was aligned with cytotoxicity study’s findings.
Introduction
Prostate cancer is considered as the most common non-skin cancer among men. Approximately, one in seven men in the USA suffers from prostate cancer, which makes it the third leading cause of cancer death in men [Citation1]. Statins are well known for their inhibitory effect against 3-hydroxy 3-methylglutaryl Coenzyme-A reductase (HMGCR) which inhibits cholesterol synthesis [Citation2]. In addition, statins reduce the levels of molecules required for cholesterol synthesis, such as mevalonate, geranylgeranyl pyrophosphate, and farnesyl pyrophosphate that are necessary for critical cellular functions such as protein synthesis, cell signaling, and cell cycle progression [Citation3]. It is worth mentioning that the interruption of these processes in cancer cells by statins decreases cancer initiation, growth and metastasis [Citation4]. Many studies have shown that statins reduce the risk of different types of cancer [Citation5] such as hepatocellular carcinoma [Citation6], gastrointestinal cancer [Citation7], lung cancer [Citation8], breast cancer [Citation9] and prostate cancer [Citation10].
Simvastatin (SMV) is a statin compound, produced synthetically as a fermentation product of Aspergillus terreus [Citation11]. Possibly, the most remarkable property of SMV is the modulation of inflammation, which may contribute to the chemoprevention of prostate cancer [Citation12]. Recently, some groups have consistently concluded that statins work efficiently against advanced prostate cancer [Citation10]. In contrary, some studies proposed that statins can increase the risk of some types of cancers [Citation13]. The main reason for these controversial findings could be attributed to the type of the statin used; so that hydrophilic and lipophilic statins have different influences on extra-hepatic tissues [Citation14]. Several studies concluded that lipophilic statins have anticancer actions [Citation15] but not all statins [Citation16]. Moreover, the type of the cancer should also be considered [Citation17]. Park et al work concluded that SMV induces apoptosis in prostate cancer cells by inhibiting nuclear factor-кB signal pathway [Citation3]. However, SMV is one of the most hydrophobic drugs with a low systemic bioavailability and extensive first pass hepatic metabolism [Citation18]. Hence, the utilization of polymeric nanoparticles (NPs)-based smart drug delivery systems has therefore been proposed as a principal solution to such limitations [Citation19]. These smart polymeric NPs carriers are continuously being implemented to improve the bioavailability and effectiveness of various drugs, reduce their undesirable side-effects and, most importantly, allow programmed sustained drug release [Citation20–22].
Poly (D, L-lactic-co-glycolic acid), PLGA is one of the most widely used biocompatible and biodegradable polymers in drug delivery systems. [Citation23, Citation24] PLGA has many interesting characteristics such as the ease of controlling its hydrophilicity/hydrophobicity affinities via changing the ratio between the hydrophobic monomer (lactic acid) and the hydrophilic monomer (glycolic acid) and hence changing the release time and biodegradation rate in accordance with the anticipated loaded target. [Citation25]
Recently, Fe3O4 nanoparticles have gained much attention because of their distinctive physicochemical properties, and their wide range of applications that include, for instance, cell imaging, thermotherapy of cancer and controlled drug delivery. The main advantages behind the magnetic-based drug delivery using Fe3O4 nanoparticles involve the enhanced therapeutic efficacy along with reduction of the adverse side effects of the drugs. [Citation26]
The present study introduces an efficient multitask hybrid drug delivery system for the treatment of prostate cancer. The system involves the chemical conjugation of SMV to acid-terminated PLGA (75:25) chains followed by its conversion into NPs, with in situ physical incorporation of more SMV and superparamagnetic iron oxide nanoparticles (SPIONS) into the PLGA NPs. The developed PLGA-based carrier system has been designed in such a way, to overcome the low bioavailability of SMV through nanoformulation, provide sustained release of both the encapsulated and the chemically conjugated SMV, provide improved good anticancer effect for the nanocarrier itself through its chemical conjugation to SMV, along with enhancing the anti-cancer effect of the formula via the magnetic targeting through the encapsulated SPIONS. Besides, the system exhibited a sustained drug release for a month upon modifying the surface of the carrier NPs by lecithin-coating. In addition, the cytotoxicity of the developed formulation was measured and compared with free SMV. Furthermore, cell cycle and caspase3 as apoptotic enzyme were conducted to evaluate the cytotoxicity mechanism.
Materials and methods
Materials
The acid-terminated PLGA co-polymer (PLGA-COOH) (75:25) was provided by PURAC, Holland. Lecithin was purchased from Thermo Fischer, Germany. Simvastatin (SMV) was obtained as a gift from T3A Co., Egypt. Poly(vinyl alcohol) (PVA), dichloromethane (DCM), thionyl chloride (SOCl2), ethanolamine, iron(II) chloride (FeCl2), and iron(III) chloride (FeCl3) anhydrous, DMEM (Invitrogen/Life Technologies), FBS (Hyclone), insulin (Sigma), penicillin-streptomycin, trypsin, EDTA, 3–(4,5,-dimethylthiazole-2-yl)-2,5-di-phenyl tetrazolium bromide (MTT), annexin-V FITC apoptosis detection kit, and invitrogen EIA kit Human caspase3 were all purchased from Sigma Aldrich, Germany. Human prostate cancer cell line, PC-3 (ATCC® CRL-1435™) was obtained from American Type Culture Collection.
Synthesis and characterization of SPIONs
Appropriate amounts of FeCl3 and FeCl2 (molar ratio Fe3+:Fe2+ of 2:1) were dissolved in 25 ml de-oxygenated distilled water. In this protocol, ethanolamine was used as the base. 3% ethanolamine solution was added dropwise to the reaction mixture under nitrogen purging, and at 60 °C with stirring to increase the pH to 11. After agitation for 3 h, the products were separated from reaction mixture by an external magnet, washed with distilled water and dried at 50 °C to obtain SPIONS. The morphology and size of the prepared SPIONS were determined by high resolution transmission electron microscope HR-TEM (JEM-2100F; JEOL, USA), and zetasizer (Nano-ZS, Malvern, UK) while their magnetic properties were assessed using vibrating sample magnetometer (VSM).
Chemical conjugation of SMV to PLGA-COOH
Thionyl chloride (15 ml) were added to a conical flask containing PLGA-COOH solution (100 mg dissolved in 5 ml of DCM) with stirring for 2 h at 50 °C, the excess liquids and the resulting gases were all evaporated. The product was re-dissolved in a small portion of DCM and reacted directly with an appropriate amount of SMV for 2 h. DCM was evaporated, and the product was purified few times via dissolution in DCM and precipitation with cold methanol. The conjugation process was confirmed by Fourier-transform infrared (FTIR) spectroscopy (Thermo Scientific, USA).
Preparation of the polymeric nanocarriers
The magnetic polymeric nanocarriers herein refer to either SMV physical loaded and unloaded in PLGA-SMV chemical conjugate. All of them were prepared using the emulsion-solvent evaporation method, as reported elsewhere [Citation27], with some modifications. NPs were prepared by dissolving 100 mg PLGA-COO-SMV conjugate in 5 ml DCM followed by dropping the resulting solution into 25 ml of a 3% PVA solution containing 10 mg SPIONS and appropriate amounts of free drug (SMV). The homogenization speed was increased gradually during addition from 5000 to 15,000 rpm for 2 min. The mixture was then on/off sonicated for 5 min in an ice bath. The emulsion was kept under mechanical stirring in a fume hood for 6 h to evaporate the residual DCM. Trying to reach the optimum conditions of poly dispersity index (PDI), particle size, charge and drug encapsulation efficiency, the drug-to-polymer (w:w) ratios were tried as 1:5, 1:4, 1:3, 1:2, 1:1 (Referred as F NPs), and 0:1 (PLGA-SMV NPs). The prepared NPs were separated by centrifugation at 20,000 rpm with cooling for 10 min and washed several times with de-ionized water, and freeze-dried. The magnetic PLGA NPs were prepared, as a reference, in the same manner but using PLGA-COOH 75:25 instead of PLGA-COO-SMV. Selected NPs were further coated with lecithin to enhance the SMV release using the previously reported thin film hydration method. The ratio between PLGA and lecithin was fixed at 1:1. [Citation28]
Characterization of the prepared SMV-loaded magnetic PLGA NPs
Particle size, polydispersity index (PDI) and zeta potential of the prepared NPs were determined using Zeta sizer (Nano-ZS, Malvern, UK), where the diluted samples were run in triplicates. The morphology and the lecithin-coating of the prepared formula (SMPS) were investigated using HR-TEM (JEM-2100F; JEOL, USA). Furthermore, the magnetic properties of the whole final formula (Lecithin-coated SMPS) were measured using VSM. The physical loading of SMV into the polymeric NPs was confirmed using (FTIR) spectroscopy (Thermo Scientific, USA). Thermal behavior of the free drug (SMV), the free PLGA, and the SMV-loaded NPs, was monitored using differential scanning calorimetry, DSC (Q20, TA instrument, USA). 6 mg of each sample was loaded and heated up at the rate of 10 °C/min in inert conditions of nitrogen as carrier gas, with a flow rate of 25 ml/min.
Entrapment efficiency
Known amounts of SMV-loaded nanocarriers were dispersed in 10 ml of absolute methanol and left with shaking in an incubator for 5 days to release all SMV from the nanocarriers. Afterwards, samples were filtered using a syringe filter, and the corresponding concentration of SMV in methanol was measured by means of UV-Vis spectrophotometry (Evolution UV 600, Thermo Scientific, USA) at λmax = 247 nm. The entrapment efficiency (EE%) of SMV in PLGA NPs was then calculated from EquationEquation (1)(1)
(1) . [Citation29]
(1)
(1)
In-vitro release profile of SMV from the developed nanocarriers
The release profile of SMV from the selected NPs, with and without lecithin coating, was studied using two compartments diffusion cells separated by a cellulose membrane of molecular weight cut-off of 12,000. Appropriate amount of each formulation equivalent to 2 mg SMV were suspended in the donor compartment in 1 ml PBS at pH 7.4, while the receiver compartment contained a mixture of PBS and methanol (ratio 9:1) and left with shaking at 37 °C for 30 days. Measurement of the concentration of the released drug at the receiver compartment was performed on a daily basis using UV-Vis spectrophotometry at λmax of 247 nm. The concentration of the released drug was measured using the following EquationEquation (2)(2)
(2) :
(2)
(2)
where Cn is the expected nth sample concentration, Cn means is the measured concentration, A is the volume of withdrawn aliquot, V is the volume of the dissolution medium, n-1 is the total volume of all the previously withdrawn samples before the currently measured sample, and Cs is the total concentration of all previously measured samples before the currently measured sample. All measurements were run in triplicates. Different release kinetic models were tested to find the best fitting model for the release data using the excel add-in software package (DDSolver) [Citation30] and interpreted according to Costa et al. [Citation31]
Cytotoxicity evaluation
Cell viability study
Cell viability was tested using the 3–(4,5,-dimethylthiazole-2-yl)-2,5-di-phenyl tetrazolium bromide (MTT) assay as reported previously [Citation32]. Void PLGA NPs, PLGA-SMV conjugate NPs, free SMV, and F (1:4) NPs were examined on human prostate cancer cell line (PC-3). Cells were cultured using DMEM supplemented with 10% FBS, 10 μg/ml of insulin and 1% penicillin–streptomycin. In a 96-well plate, an amount of 100 μL of a cell suspension (cells density, 1.2 – 1.8 × 10,000 cells/well) and 100 μL of logarithmic dilution of each sample (0.01–1000 µg/mL) were plated per each well. Plate was incubated for 24 h at 37 °C. For each well, 100 μL of MTT was mixed and incubated for 4 h. Then, 1 ml of MTT solubilization solution was added to dissolve formazan crystals. After 45 s, the purple color was observed. Optical density at 595 nm was measured spectrophotometrically to detect viable cells using the following EquationEquation (3)(3)
(3) :
(3)
(3)
Cell cycle study
Cell cycle of PC-3 cells was evaluated at the IC50 concentration of SMV and F (1:4) NPs using BD FACSCaliburTM (Beckton Dickinson) flow cytometer. Four phases including pre-G1 (apoptosis), G0/G1, S and G2/M phases were determined by measuring the cell uptake of propidium iodide (PI) by flow cytometer. [Citation33] After trypsinization of the adherent cells, cells were suspended in 10% FCS and centrifuged twice for 5 min at 1000 rpm to obtain pellet. The pellets were then suspended in 1 ml PBS. Cells suspension was then added to 2.5 ml of absolute ethanol to prevent clustering of cells during the fixation then incubated on ice for 15 m (or overnight at –20 °C). After centrifugation for 5 min at 1000 rpm, PC-3 cells were added to a 6-well plate with a density (1 × 106 cells/mL) and incubated for 12 h. Then, the cells were washed and re-suspended in 500 µL PI-solution and incubated for 40 min at 37 °C with 1 mg/mL RNase and 0.05% Tritin X-100. After incubation, 3 ml of PBS was added to the suspension and centrifuged at 100 rpm for 5 min then the remained pellet was suspended in 500 µl PBS. Samples were applied to the cells for 24 h and DNA content analysis was measured using BD FACSCaliburTM flow cytometer.
Annexin V/propidium iodide apoptosis assay
Apoptosis and necrosis mechanisms were evaluated using annexin V/propidium iodide assay on PC-3 as reported previously [Citation34]. Briefly, the IC50 concentration of SMV and F (1:4) NPs were incubated with PC-3 cells in 6-well plates for 24 h. All study conditions were like cell cycle analysis study. After incubation, cells were harvested and washed with PBS. Annexin-V FITC apoptosis detection kit was used according to manufacturer’s instruction. The samples were measured by BD FACSCaliburTM flow cytometer.
ELISA immunoassay for caspase3 (apoptotic enzyme)
HepG2 cells were cultured in RPMI 1640 supplemented with 10% FBS and were incubated at 37 °C. HepG2 cells were treated with the IC50 concentration of SMV and F (1:4) NPs. After 24 h of treatment, cells were trypsinized and centrifuged. The pellet was lysed in cell extraction buffer at 4 °C for 45 min followed by centrifugation for 20 min. Lysates were then collected, and the levels of the apoptotic markers caspase-3 were measured using ELISA colorimetric kits according the manufacturer’s instructions as previously reported [Citation35]. The cell lysate was diluted and added to the wells of microtiter plates for the ELISA kits. A secondary biotin-linked antibody specific to the protein captured by the primary antibody was added to bind the captured protein. Then, streptavidin-HRP complex was added. The reaction was terminated by the addition of a stop solution, and a colored product was measured at 450 nm by a plate reader (Robonik P2000 ELISA Reader, USA).
Statistical analysis
All results were expressed as mean ± standard deviation. Significant difference tests were applied using student’s t-test and one-way analysis of variance (ANOVA) to all data obtained. All tests were estimated using the software GraphPad Prism Software Version 6.
Results and discussion
This study aims at introducing an efficient drug delivery system for the treatment of prostate cancer using SMV, as the acting drug, and SPIONS to guide the carrier system towards tumor. To achieve this goal, a nanocarrier system was fabricated as illustrated in . First, acid terminated PLGA was linked to SMV via ester bond formation (PLGA-COO-SMV), followed by NPs formation with physical entrapment of SPIONS and SMV. Finally, the selected formulation was coated by lecithin to enhance the drug release properties. The activity of the engineered formulations was evaluated against human prostate cancer cell line.
Figure 1. Chemical conjugation of SMV and PLGA-COOH: (a) Synthesis of SMV-PLGA conjugate followed by fabricating of lecithin-coated SMV/SPIONS-loaded PLGA-conj-SMV NPs, and (b) FTIR analysis of PLGA-COOH, SMV, and the chemical conjugate PLGA-COO-SMV. The absorption peak of OH (3544.57 cm−1) of SMV disappeared due to the conjugation with PLGA-COOH.
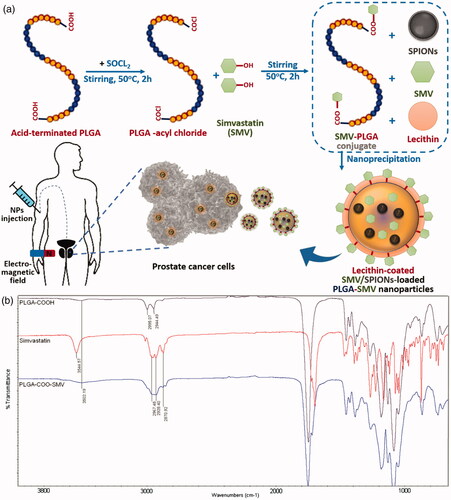
Chemical conjugation of SMV and PLGA-COOH
The chemical reaction of PLGA-COOH and SMV, as illustrated in , occurred between the carboxyl groups of PLGA and the hydroxyl groups of SMV forming ester groups. First, the acyl chloride (PLGA-CO-Cl) was formed, to provide the good leaving group “Cl”, by reaction of the PLGA-COOH with thionyl chloride. [Citation36] Afterwards, the acyl chloride reacted readily with SMV through its hydroxyl groups to form the corresponding ester in the form PLGA-COO-SMV. The formed ester was solubilized in DCM and re-precipitated with cold methanol three times for purification. This ester formation reaction demonstrated changes in the FTIR charts of both reactants when compared to the product. The main changes involve the disappearance of the absorption peak of phenolic O–H of SMV, reduction or disappearance of terminal O–H of polymer, and finally the appearance of almost all the other peaks of the two reactants in the chart of the product. FTIR results in showed the absorption stretching and bending vibrational peaks of SMV, PLGA-COOH, and PLGA-COO-SMV. For SMV, the chart demonstrated phenolic O–H stretching peak at 3544 cm−1, multiple alkyl C–H stretching at 2850–2950 cm−1, aromatic and alkenyl C–H stretching around 3000–3030 cm−1, aromatic C = C bending at 1700 cm−1, and ester C = O stretching at 1735 cm−1. On the other hand, PLGA-COOH showed some characteristic absorption peaks [Citation37], including two or three alkyl C–H stretching, which are less crowded than the corresponding area in SMV, a weak absorption peak at approximately 3500 cm−1, which is attributed to the terminal hydroxyl groups in the copolymers, and the C = O stretching at 1750 cm−1. Nevertheless, the FTIR of the product (PLGA-COO-SMV) showed disappearance of phenolic O-H of SMV as expected and reduction in O–H of the polymer and incorporation of all the other peaks of the two reactants in the product, especially the multiple C–H stretching of SMV, the C = C and C = O from SMV, and the unchanged peaks of the polymer itself, and hence proving the successful conjugation process.
Formulation and characterization of nanocarriers
Different formulations were prepared by changing drug to polymer ratio from 1:1 to 1:4 according to a modified nanoemulsion-solvent evaporation method [Citation27]. Also, plain nanoformulation was prepared as control. The ratio between polymer and SPIONS were kept constant at 10:1 (w:w), respectively.
Effect of drug-to-polymer ratio
The change in ratio between drug and polymer had a significant effect on the drug entrapment percentage (EE%) (), and a moderate effect on particle size and PDI (), while it did not alter the corresponding Z-potential (). The drug-to-polymer ratios of 1:1 and 1:2 showed the lowest EE% (50–70%) as assessed from EquationEquation (1)(1)
(1) by spectrophotometry, and the largest dynamic particle size (300–320 nm), and a slightly high PDI readings (0.31–0.44), with observable salting out of portion of the drug. Hence, the 1:1 and 1:2 ratios were totally excluded. Starting from the formula with the ratio 1:3, there were no salting out observations, acceptable EE% (76–80%) and PDI (0.24) and smaller particle size. However, a better entrapment and PDI were still needed. The last two ratios, 1:4 and 1:5 gave much better results in all measured parameters. In this work, formula with the ratio 1:4 was selected for further characterization. The Z-potential did not change in all the drug-to-polymer ratios, because the effective contribution in particle charge is the surface charge not the bulk. The particle surface charges were in the range from −12 to −14 mV, which depends mainly on the end groups of PLGA and PVA. Having such high surface charges provide stable nanosuspensions, as the coulombic repulsion forces between particles will overcome the Wander Waals attraction forces and hence prevent any possible agglomerations. [Citation38]
Figure 2. Characterizations of the engineered NPs: (a) Entrapment efficiency, EE%, (b) Particle size and PDI, and (c) zeta-potential of different formulations; (d) TEM images of the developed SPIONS (gray shadow represents SPIONS), the selected area is an electron diffraction (SAED) image (inset), (e) TEM images of SMV-loaded magnetic PLGA-COO-SMV NPs with lecithin coating layer (purple shadow represents the lecithin-coating and the dark red shadow represents SPIONS-loaded PLGA core), and (f) Vibrating sample magnetometer (VSM) results of SPIONS and the selected formulation, F (1:4) NPs. Data are represented as mean ± SD (*p < .05, **p < .01, ***p < .001, ****p < .0001 and n ≥ 3).
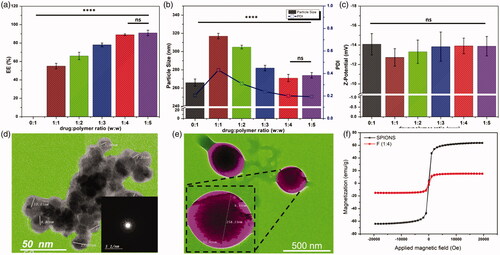
Synthesis and characterizations of SPIONS
The superparamagnetism is a type of magnetism that occurs in a specific size range (3–50 nm) depending on the type of materials used. For magnetite, the size of NPs must be below 25 nm [Citation39], so that they work as one single domain with higher susceptibility for magnetism than the corresponding paramagnetic materials. When it comes to drug delivery, another importance of superparamagnetic materials must be considered; i.e. when the external magnetic field is switched off, the magnetization turns back to zero instantly, with negligible remanence and coercivity, and hence agglomeration and possible capillary vessels embolization are evaded. [Citation39]
In this proposed method, ethanolamine was used for two main reasons; first, to elevate the pH of the medium to form the oxides. Second, to act as a good capping agent for fulfilling the very small size needed for this work and stabilizing the formed NPs. The size and morphology of the prepared particles were assessed using TEM imaging as in , which shows that the prepared NPs are spherical with sizes around 10 nm as an average. The selected area electron diffraction (SAED) image in inset, shows the crystalline nature of the prepare SPIONS. The magnetic properties were measured by VSM at room temperature and illustrated by the hysteresis loops in , which showed good magnetism with a specific saturation magnetization (Ms) at 63.5 emu/g. These good results can be attributed to their small sizes, which decrease the oxygen content and increase iron content, as discussed earlier by Thapa et al. [Citation40]
Lecithin coating of the selected formulation
The TEM images of the prepared lecithin-coated particles showed spherical NPs with a size around 258 nm, with SPIONS embedded inside them, as illustrated in . The successful lecithin coating was also clear, with a thickness of around 11 nm. The VSM results of the coated NPs are illustrated in . As discussed above, the selected formulation F (1:4) NPs with lecithin coating layer exhibited strong magnetic properties (Ms =15.4 emu/g), which are comparable or even stronger than the recently published work. [Citation41–44] However, this reduction in magnetism from 63.5 to 15.4 emu/g, might be attributed to the shielding effect of the non-magnetic coatings of the nanocarrier (PLGA-derivative), PVA and lecithin.
FTIR analysis of the lecithin-coated NPs
FTIR results () show the same peaks of PLGA-COOH and SMV as the ones discussed in (Chemical conjugation of SMV and PLGA-COOH) section, and so do the F (1:4) NPs, except that the absorption peaks of hydroxyl groups, at 3544 cm−1, of SMV are still found after the loading process which confirms that the encapsulation process happens physically by entrapping SMV inside PLGA-COOH matrix. On the other hand, in section (Chemical conjugation of SMV and PLGA-COOH), this absorption peak of hydroxyl group disappeared upon doing the chemical conjugation as the OH group was bound chemically to the polymer forming the corresponding ester PLGA-COO-SMV. Moreover, FTIR of the lecithin-coated formula shows peaks of all of ingredients; SMV, PLGA-COO-SMV and lecithin with its broad absorption peak [Citation45, Citation46] at 3200–3400 cm−1. Hence, the FTIR results confirm both the encapsulation and coating processes.
Thermograph from DSC results
The DSC measurements, were carried out to confirm the physical encapsulation of SMV inside PLGA 75:25 NPs and the success of lecithin-coating of the prepared NPs. The glass transition temperature (Tg) of pure PLGA polymer appeared as an endothermic peak at 50.7 °C. [Citation47] DSC thermogram of pure SMV showed a strong endothermic peak at 139 °C, corresponding to its melting temperature. [Citation48] Besides, SMV-loaded polymeric nanocarrier exhibited both of the polymer Tg, with a slight shift, at around 51.5 °C and the SMV melting peak at a slightly lower temperature of 136 °C due to a very fine change in its crystallinity, which confirms the successful entrapment of drug. Additionally, the lecithin-coated NPs showed a broad endothermic peak due to the overlap of the endothermic peaks from PLGA, SMV and lecithin. The DSC thermogram of the lecithin-coated PLGA NPs matches the thermographs of the recently published work [Citation28], which confirms the lecithin coating step.
In-vitro release profile
The release profile of a drug from a specific NPs is a result of the contribution of three main mechanisms; the burst drug release due to desorption of the drug from the surface of NPs, diffusion from the polymer matrix to the medium and erosion/biodegradation of the carrier NPs. [Citation49, Citation50] The contribution of each of these three routes vary depending on the encapsulation process as well as the polymer and drug nature, and it is significantly affected by the solubility. Moreover, the diffusion/erosion-caused release of an encapsulated drug is mostly slower than desorption of the surface-adsorbed drug. The results of the release profiles of SMV from lecithin-coated and uncoated NPs in show that the lecithin-coating enhanced the sustained release of SMV by minimizing the burst release, allowing more controlled release through diffusion with reducing PLGA NPs erosion, which matches the recently published studies. [Citation9, Citation28] For instance, the lecithin-coated formula released 20% on the first two days, while the uncoated formula released around 29% at the corresponding time. Starting from day 3 and until day 23, a well sustained release behavior was observed due to the diffusion mechanism. After day 26, the erosion/biodegradation of the coated polymeric NPs contributed weakly, due to the coating effect, and gave a slightly faster release when compared to the quick jump in release profile of the uncoated formula. By day 31, around 89% and 98% of the SMV were released from the coated and uncoated formulae, respectively. The slow release rate of the drug can be attributed to the hydrophobic nature of both the carrier (PLGA) and drug itself (SMV). Hence, the prepared lecithin-coated SMPS NPs was chosen for the prostate cancer cells treatment providing the required controlled sustain release.
The release profiles of SMV from coated and uncoated formulations were fitted to different kinetic models, including zero order, first order, Higuchi and Korsmeyer–Peppas. The best fitting model for both release profiles was Korsmeyer–Peppas model with highest r2 (0.99). Korsmeyer–Peppas is a mainly model describes drug release from a polymeric system where the diffusional factor representing the drug-release mechanism. The diffusional exponent value for uncoated formulation was 0.422 which represent Fickian model while for the coated formulation it was 0.527 which represents non-Fickian model. [Citation31]
Cytotoxicity evaluation
Cell viability study
Repurposing of SMV, antihyperlipidemic drug, as anticancer agent was examined in human prostate cancer cell line using MTT assay. The results of void PLGA NPs, PLGA-SMV conjugate NPs, selected formula F (1:4) NPs and free SMV were expressed in dose response (inhibitory) curve () and growth inhibitory concentration (IC50) values after 24 h (). SMV is prescribed as an antihyperlibidimic drug. Recently, several studies were focused on understanding its effects on proliferation and inflammation, where these two processes are involved in tumor growth. Kochuparambil et al investigated the Akt pathway in prostate cancer cells for SMV. [Citation51] Current study showed a significant decrease in the viability of the cells PC-3 () in a dose-dependent manner. The IC50 value of void PLGA NPs determined to be 2095 μg/mL while free SMV attained 1.15 μg/mL (). The conjugation of SMV to PLGA polymer significantly decreased (p < .05) the IC50 to 1028 μg/mL (). However, this concentration still high. On the other hand, encapsulation of SMV in PLGA-SMV NPs with lecithin coating significantly decreased the IC50 to only 0.22 μg/mL (p < .05) when compared to both free SMV and SMV-conjugated-PLGA (). This could be attributed to the SMV release behavior as the diffusion of physically loaded SMV will be faster than breaking the chemical bond of conjugated SMV. On the other hand, lecithin-coating will enhance both the release and cell uptake due to its lipid nature. This finding proved the efficacy of the engineered nanoformulations. Furthermore, it will be guided by external magnetic field towards tumor location because of SPIONs encapsulation.
Figure 4. Cell viability study: (a) Dose response (inhibitory) curve, and growth inhibitory concentration (IC50) values after 24 h of void PLGA NPs, PLGA-SMV conjugate NPs, selected formula F (1:4) NPs, and free SMV. Data are represented as mean ± SD (*p < .05, **p < .01, ***p < .001, ****p < .0001 and n ≥ 3).
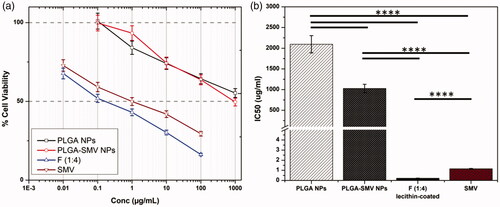
Cell cycle study
Cell cycle is mainly a cell reproduction through replication of DNA where nucleus division and cytoplasm partitioning occur to divide into two cells. This cycle consists of four main phases. Two gaps occur during the cycle. The first gap is G1 which arises between M phase (nuclear division) and S phase (DNA synthesis). The second gap is G2 between S phase and M phase. The main goal of these two gaps is repairing DNA damage and errors. [Citation35] The behavior of cell cycle in presence of SMV and the final formula was evaluated on PC-3 cells (). Remarkably, exposure of PC-3 cells to both SMV and the final formula significantly increased the percentage of cells at pre-G1 by 9-folds and 14-fold compared to control PC-3 cells, respectively (). Furthermore, a significant reduction in G0/G1 phases compared to control was noted. Moreover, both SMV and the final formulation significantly increased the percentage of cells at G2-M by 1.8-folds and 2-folds compared to control, respectively.
Figure 5. Mechanisms of cytotoxicity studies: (a–c) Cell cycle study, (d–f) Annexin V/Propidium iodide apoptosis assay (LL, viable; LR, early apoptotic; UR, late apoptotic; UL, necrotic), (g) Cell distribution in different cell cycle phases, (h) Cell distribution on early apoptotic, late apoptotic and necrotic, and (i) Protein level of caspase-3 at their IC50 concentrations of SMV, and final formula, lecithin-coated F (1:4) NPs. Data are represented as mean ± SD (*p < .05, **p < .01, ***p < .001, ****p < .0001 and n ≥ 3).
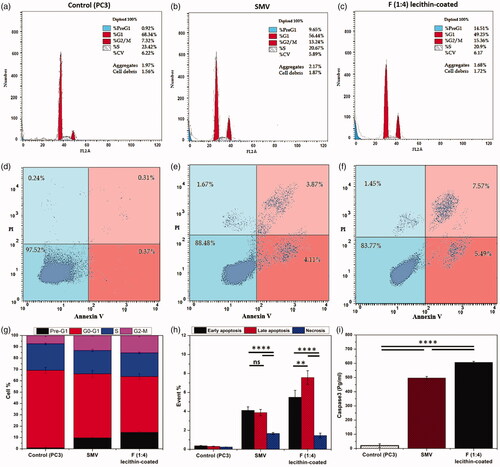
Annexin V/propidium iodide apoptosis assay
Based on the results obtained from the cell cycle study (), both SMV and the optimum nanoformulation, F (1:4) NPs had an impact on both pre-G1 phase (apoptosis) and G2-M phases. Therefore, annexin-V/Propidium iodide staining was performed to confirm the mechanisms on cell proliferation by apoptosis (). Annexin-V is conjugated to FITC to facilitate flow cytometric analysis which mainly used to distinguish between necrosis (low level of Annexin-V) and apoptosis (high level of Annexin-V). On the other hand, propidium iodide (PI) staining is used to distinguish between early (low level of PI) and late apoptosis (high level of PI). [Citation52] Flow cytometric analysis revealed that PC-3 cells treated with free SMV showed a significant increase (p < .05) in annexin-V positive apoptotic cells as compared to control. Moreover, staining the cells with PI showed no significant difference between early and late apoptotic cells. On the other hand, encapsulation and conjugation of SMV in nanocarrier showed a significant increase in annexin-V positive apoptotic cells with significant effect on late apoptosis. These results are in agreement with the cell cycle study which indicated an increase of cell population in pre-G1 (apoptosis).
Effect on caspase3 (apoptotic enzyme)
Caspase-3 is one of protease family enzymes which play essential roles in programmed cell death. It is mainly activated during the early stages of apoptosis where it is synthesized as an inactive pro-enzyme then undergoes activation during apoptosis cascade. Treatment of PC-3 cells with SMV and F (1:4) NPs significantly increased the level of active caspase-3 compared to control untreated cells (). The up-regulation of the Caspase 3 confirms results of both cell cycle study and annexin V/Propidium iodide apoptosis assay. All of these findings were in alignment with the published report on statins as potential anticancer agents. [Citation53] In this report several mechanisms were discussed specially the effect of statins on proapoptotic phase where activation of caspase-3 and retardation of G2-M phase were highlighted.
Conclusions
The developed PLGA-based hybrid nanocarrier significantly improved the SMV anticancer activity against human prostate cancer cell line through both apoptosis mechanism and retardation of G2-M phase of cell cycle. Incorporation of SPIONs into the nanocarrier could be beneficial in guiding the drug to tumor cells without side effect on other organs. Based on the obtained data, further in vivo and clinical studies like pharmacodynamics, pharmacokinetic and toxicological assessments are required to find the clinical possibility of the developed nanosystem.
Disclosure statement
No potential conflict of interest was reported by the authors.
References
- Lewis R. Prostate Cancer Screening. Physician Assistant Clinics. 2018;3:1–9.
- Stancu C, Sima A. Statins: mechanism of action and effects. J Cell Mol Med. 2001;5:378–387.
- Park YH, Seo SY, Lee E, et al. Simvastatin induces apoptosis in castrate resistant prostate cancer cells by deregulating nuclear factor-κB pathway. J Urol. 2013;189:1547–1552.
- Ahmadi Y, Ghorbanihaghjo A, Argani H. The balance between induction and inhibition of mevalonate pathway regulates cancer suppression by statins: A review of molecular mechanisms. Chemico-Biological Interactions. 2017;273:273–285.
- Lonardo A, Loria P. Potential for statins in the chemoprevention and management of hepatocellular carcinoma. J Gastroenterol Hepatol. 2012;27:1654–1664.
- Tsan Y-T, Lee C-H, Ho W-C, et al. Statins and the risk of hepatocellular carcinoma in patients with hepatitis C virus infection. JCO. 2013;31:1514–1521.
- Lee J, Lee SH, Hur KY, et al. Statins and the risk of gastric cancer in diabetes patients. BMC Cancer. 2012;12:596.
- Khurana V, Bejjanki HR, Caldito G, et al. Statins reduce the risk of lung cancer in humans: a large case-control study of US veterans. CHEST. 2007;131:1282–1288.
- Ahmaditabar P, Momtazi‐Borojeni AA, Rezayan AH, et al. Enhanced entrapment and improved in vitro controlled release of n‐acetyl cysteine in hybrid PLGA/lecithin nanoparticles prepared using a nanoprecipitation/self‐assembly method. J Cell Biochem 2017;118(12):4203–4209
- Platz EA, Leitzmann MF, Visvanathan K, et al. Statin drugs and risk of advanced prostate cancer. JNCI. 2006;98:1819–1825.
- Jun SW, Kim M-S, Kim J-S, et al. Preparation and characterization of simvastatin/hydroxypropyl-β-cyclodextrin inclusion complex using supercritical antisolvent (SAS) process. Eur J Pharma Biopharma. 2007;66:413–421.
- Greenwood J, Steinman L, Zamvil SS. Statin therapy in autoimmunity: from protein prenylation to immunomodulation. Nat Rev Immunol. 2006;6:358.
- Chang CC, Ho SC, Chiu HF, et al. Statins increase the risk of prostate cancer: a population-based case-control study. Prostate. 2011;71:1818–1824.
- Rosenson RS. Current overview of statin-induced myopathy. Am J Med. 2004;116:408–416.
- Taylor ML, Wells BJ, Smolak MJ. Statins and cancer: a meta-analysis of case-control studies. Eur J Cancer Prev. 2008;17:259–268.
- Pocobelli G, Newcomb PA, Trentham A., et al. Statin use and risk of breast cancer. Cancer. 2008;112:27–33.
- Baron JA. Statins and the colorectum: hope for chemoprevention? Cancer Prev Res. 2010;3:573–575.
- Alam M, Ahmed S, N, et al. Chemical engineering of a lipid nano-scaffold for the solubility enhancement of an antihyperlipidaemic drug, simvastatin; preparation, optimization, physicochemical characterization and pharmacodynamic study. Artif Cell Nanomed Biotechnol. 2017;1–12.
- Muzykantov VR. Targeted drug delivery to endothelial adhesion molecules. ISRN Vascul Med. 2013;2013:1.
- Kawashima Y. Nanoparticulate systems for improved drug delivery. Adv Drug Deliv Rev. 2001;47:1–2.
- Rajalakshmi S, Vyawahare N, Pawar A, et al. Current development in novel drug delivery systems of bioactive molecule plumbagin. Artif Cell Nanomed Biotechnol. 2018;1–10. doi:10.1080/21691401.2017.1417865
- Sivasami P, Hemalatha T. Augmentation of therapeutic potential of curcumin using nanotechnology: current perspectives. Artif Cell Nanomed Biotechnol. 2018;1–12. doi:10.1080/21691401.2018.1442345
- Naves L, Dhand C, Almeida L, et al. Poly (lactic-co-glycolic) acid drug delivery systems through transdermal pathway: an overview. Prog Biomater. 2017;6:1–11.
- Ahmad N, Alam MA, Ahmad R, et al. Preparation and characterization of surface-modified PLGA-polymeric nanoparticles used to target treatment of intestinal cancer. Artif Cell Nanomed Biotechnol. 2018;46:432–446.
- Gentile P, Chiono V, Carmagnola I, et al. An overview of poly (lactic-co-glycolic) acid (PLGA)-based biomaterials for bone tissue engineering. Ijms. 2014;15:3640–3659.
- Ahmadkhani L, Akbarzadeh A, Abbasian M. Development and characterization dual responsive magnetic nanocomposites for targeted drug delivery systems. Artif Nanomed Biotechnol 2018;46(5):1052–1063.
- El-Sherbiny IM, Smyth HDC. Controlled release pulmonary administration of curcumin using swellable biocompatible microparticles. Mol Pharma. 2012;9:269–280.
- Ali IH, Khalil IA, El-Sherbiny IM. Single-dose electrospun nanoparticles-in-nanofibers wound dressings with enhanced epithelialization, collagen deposition, and granulation properties. ACS Appl Mater Interfaces. 2016;8:14453–14469.
- Agnihotri SA, Jawalkar SS, Aminabhavi TM. Controlled release of cephalexin through gellan gum beads: Effect of formulation parameters on entrapment efficiency, size, and drug release. Eur J Pharm Biopharm. 2006;63:249–261.
- Zhang Y, Huo M, Zhou J, et al. DDSolver: an add-in program for modeling and comparison of drug dissolution profiles. Aaps J. 2010;12:263–271.
- Costa P, Lobo JMS. Modeling and comparison of dissolution profiles. Eur J Pharma Sci. 2001;13:123–133.
- Senthilraja P, Kathiresan K. In vitro cytotoxicity MTT assay in Vero. HepG2 and MCF-7 Cell Lines Study of Marine Yeast. J Appl Pharm Sci. 2015;5(3):080–084.
- Liu T-Y, Tan Z-J, Jiang L, et al. Curcumin induces apoptosis in gallbladder carcinoma cell line GBC-SD cells. Cancer Cell Int. 2013;13:64.
- Sedeky AS, Khalil IA, Hefnawy A, et al. Development of core-shell nanocarrier system for augmenting piperine cytotoxic activity against human brain cancer cell line. Eur J Pharm Sci. 2018;118:103–112.
- Eldehna WM, Ibrahim HS, Abdel-Aziz HA, et al. Design, synthesis and in vitro antitumor activity of novel N-substituted-4-phenyl/benzylphthalazin-1-ones. Eur J Med Chem. 2015;89:549–560.
- Morrison RTB, Robert Neilson, editor. Organic Chemistry/Edition 6. 6 ed: J. Chem. Educ. 1992;69 (11):A305. doi:10.1021/ed069pA305.2
- Xiao H, Wang L. Effects of X-shaped reduction-sensitive amphiphilic block copolymer on drug delivery. Int J Nanomed. 2015;10:5309.
- Betancourt T, Brown B, Brannon-Peppas L. Doxorubicin-loaded PLGA nanoparticles by nanoprecipitation: preparation, characterization and in vitro evaluation. Nanomed. 2007;2:219–232.
- Arruebo M, Fernández-Pacheco R, Ibarra MR, et al. Magnetic nanoparticles for drug delivery. Nano Today. 2007;2:22–32.
- Thapa D, Palkar V, Kurup M, et al. Properties of magnetite nanoparticles synthesized through a novel chemical route. Mater Lett. 2004;58:2692–2694.
- Cui Y, Zhang M, Zeng F, et al. Dual-targeting magnetic PLGA nanoparticles for co-delivery of paclitaxel and curcumin for brain tumor therapy. ACS Appl Mater Interfaces. 2016;8:32159–32169.
- Ebrahimi E, Khandaghi AA, Valipour F, et al. In vitro study and characterization of doxorubicin-loaded magnetic nanoparticles modified with biodegradable copolymers. Artif Cell Nanomed Biotechnol. 2016;44:550–558.
- Mosafer J, Teymouri M. Comparative study of superparamagnetic iron oxide/doxorubicin co-loaded poly (lactic-co-glycolic acid) nanospheres prepared by different emulsion solvent evaporation methods. Artif Cell Nanomed Biotechnol. 2018;46:1146–1155.
- Shabestari Khiabani S, Farshbaf M, Akbarzadeh A, et al. Magnetic nanoparticles: preparation methods, applications in cancer diagnosis and cancer therapy. Artif Cell Nanomed Biotechnol. 2017;45:6–17.
- Whittinghill J, Norton J, Proctor A. A Fourier transform infrared spectroscopy study of the effect of temperature on soy lecithin-stabilized emulsions. Vol. 76. 1999.
- Nzai J, Proctor A. Soy lecithin phospholipid determination by fourier transform infrared spectroscopy and the acid digest/arseno-molybdate method: a comparative study. J Am Oil Chem Soc. 1999;76:61–66.
- Dillen K, Vandervoort J, Van den Mooter G, et al. Evaluation of ciprofloxacin-loaded Eudragit® RS100 or RL100/PLGA nanoparticles. Int J Pharma. 2006;314:72–82.
- Swain RP, Pendela S, Panda S. Formulation and evaluation of gastro-bilayer floating tablets of simvastatin as immediate release layer and atenolol as sustained release layer. Ijps. 2016;78:458–468.
- Aguilar ZP. Chapter 5 - Targeted Drug Delivery. Nanomaterials for Medical Applications. USA: Elsevier; 2013. p. 181–234.
- Lu X-Y, Wu D-C, Li Z-J, et al. Chpater 7 - Polymer Nanoparticles. In: Villaverde A, editor. Progress in Molecular Biology and Translational Science. Vol. 104. USA: Academic Press; 2011. p. 299–323.
- Kochuparambil ST, Al-Husein B, Goc A, et al. Anticancer efficacy of simvastatin on prostate cancer cells and tumor xenografts is associated with inhibition of Akt and reduced prostate-specific antigen expression. J Pharmacol Exp Ther. 2011;336:496–505.
- Elbaz NM, Khalil IA, Abd-Rabou AA, et al. Chitosan-based nano-in-microparticle carriers for enhanced oral delivery and anticancer activity of propolis. Int J Biol Macromol. 2016;92:254–269.
- Altwairgi AK. Statins are potential anticancerous agents (review). Oncol Rep. 2015;33:1019–1039.