Abstract
An ideal in vitro drug screening model is important for the drug development. In addition to monoculture systems, 3 dimensional (3D) coculture systems are extensively used to simulate the in vivo tumor microenvironment as cell-cell and cell-extracellular matrix interactions within the tumor tissues can be mimicked. In this study, in vitro 3D suspension coculture multicellular spheroids with core/shell cell distribution were developed on chitosan-coated surfaces. Based on the characteristic of chitosan inhibiting cell adhesion, SW620 (colon cancer cell line), 3A6 (mesenchymal stem-like cell line) and Hs68 (foreskin fibroblast line) cells could aggregate to form 3D coculture spheroids with intimate cell contacts. When cells were cocultured on chitosan, 3A6 and Hs68 cells always located in the core of spheroids and were completely enveloped by SW620 cells due to their N-cadherin protein expression following the differential adhesion hypothesis. The core cells could be the feeder layers to stimulate the shell SW620 cells to enhance their mitochondria activity. Moreover, 3D coculture core/shell multicellular spheroids could enhance the resistance of SW620 cells against the cytotoxicity effect of chemotherapy drugs. To sum up, based on the specificity of the core/shell coculture multicellular spheroids, a novel in vitro tumor model was proposed in this study.
Introduction
Cancer is a great threat to human health. To develop new anti-cancer drugs, the cytotoxicity and effectiveness of drugs are usually determined by using low-cost in vitro drug screening platforms before animal and clinical studies. An ideal in vitro drug screening method can reduce the cost of animal experiments and boost the development of new drugs [Citation1]. Traditionally, cancer cells are cultured on the plates to form two-dimension (2D) monolayered morphology for the cytotoxicity tests. However, cancer cells form three-dimension (3D) tumor in vivo. Therefore, compared with 2D system, 3D culture systems are better candidates to build in vitro cancer models [Citation2–4]. Some techniques have been reported to create 3D culture environments for the formation of cancer cell aggregates in vitro, such as by using non-adherent culture plates [Citation5], gyratory shaker [Citation6], 3D scaffolds [Citation2,Citation7] and decellularized matrix [Citation8].
Chitosan is prepared from the deacetylation of chitin [Citation9–11]. Chitosan with characteristics of biocompatible and biodegradable properties has been used in biomedical field including wound healing [Citation12,Citation13] and the treatment of age-related diseases [Citation14–16]. It is known that different cancer cells show different characteristics on different biomaterials [Citation17,Citation18], and we have shown that chitosan could enhance the CD44 expression, one of the cancer stem cell markers, of cancer cells in our previous study [Citation19]. This result indicates that chitosan would enhance the drug resistance of cancer population. Dissimilar to traditional culture system in which cultured cells exhibit monolayered morphology [Citation20], chitosan developed a suspension culture system to form cellular aggregates spontaneously [Citation21,Citation22]. Therefore, chitosan was used to suspend cancer cells to form 3D multicellular spheroids without additional substrate modification, driving force or 3D scaffold design in this study.
As cancer cells can interact with other cells to regulate their function and peripheral microenvironment [Citation20,Citation23,Citation24], it is inappropriate to predict cancer cell behaviors in vivo by just considering the response of cancer cells themselves in vitro. Especially, it has been proposed that mesenchymal stem cells (MSCs) could promote the growth and angiogenesis of tumors [Citation25], and fibroblasts could also promote the proliferation and decrease the drug sensitivity of cancer cells [Citation26–28]. Compared with monoculture, coculture of cancer cells with MSCs or fibroblasts on specific biomaterial surface can provide more important and interesting data for studies [Citation29–33]. To this end, we tried to coculture human colon cancer cell line (SW620) with human mesenchymal stem-like cell line (3A6) or human foreskin fibroblast line (Hs68) to form 3D coculture multicellular tumor spheroids on chitosan. To simplify the system, the 3A6 and Hs68 cell lines were used to simulate cancer-associated MSCs and fibroblasts. This 3D suspension coculture system could studied the cell interaction within aggregates, and the induction of cell responses by external stimulates [Citation34].
The 3D suspension coculture system has been proposed, and it has been proved that it is better than 2D culture and 3D monoculture systems [Citation35,Citation36]. However, most systems could create 3D mixed multicellular spheroids only [Citation37,Citation38], and the exposed and dispersed stem cells or fibroblasts might lead to underestimate the anti-drug resistance of cells or overestimate the cytotoxicity of drugs. Recently, hanging-drop [Citation39], ultra-low attachment 96-well plates [Citation40], RGD peptide platform with the liquid overlay technique [Citation41] and polyelectrolyte microcapsules [Citation41] were proposed to create core/shell coculture multicellular spheroids, but these techniques are more time, labor and money consuming. From the conceptual basis of the feeder layers, we create core/shell coculture multicellular spheroids by biomaterials characteristics, which stem cells or fibroblasts aggregated in the core of the spheroids for supporting and cancer cells covered over the shell of the spheroids for being supported, by the differential adhesion hypothesis (DAH) in this study. In other words, cells with different amount of cadherin expression were used to create 3D core/shell coculture multicellular spheroids. Then, whether the 3D core/shell heterogeneous coculture multicellular spheroids were an effective screening model of anti-cancer drugs was following investigated.
Materials and methods
Preparation of biomaterial substrates
Chitosan (Sigma-Aldrich C3646) with more than 75% deacetylation was dissolved in acetic acid to prepare 1% solution. The prepared polymer solution was coated on tissue culture polystyrene (TCPS) plates (Corning), dried at 60 °C, washed with the sterilized water and exposed to ultraviolet light for 30 min for cell culture.
Cell culture
Cells were maintained in Dulbecco's Modified Eagle Medium (Gibco) which contained 10% fetal bovine serum (Biological industries). The 3A6 cell line was a gift from Professor Hung, Shih-Chieh (China Medical University, Taiwan), and 3A6 cells have been verified that they have the characteristics of MSCs [Citation42].
The transwell (Corning 3413) was used to evaluate the effects of cells to SW620 treated with doxorubicin (Sigma-Aldrich). The membrane pore size of the transwell insert was 0.4 um. SW620 treated with 1 µM doxorubicin were monocultured in the top chambers of the transwell inserts (10,000 cells/cm2), and healthy 3A6, Hs68 and SW620 cells were monocultured in the bottom chambers of the other new 24-well TCPS plate (10,000 cells/cm2). After 3 d, the inserts were moved to the 24-well plate which has been cultured by healthy cells. These cells were cocultured for another 3 d, and then we only examined the mitochondria activities of doxorubicin-treated SW620 cells. For chitosan treatment, cells were collected from TCPS and seeded on chitosan-coated surfaces at a density of about 15,000 cells/cm2 totally. The morphology of multicellular spheroids was visualized under a microscope (LEICA DMI6000). For tracking the cells, the dyes (Invitrogen Cell Tracker™ CMFDA and CMRA) were used to stain the cells according to the manufacture’s protocol. Briefly, cells were incubated in a serum-free medium with 1 μM CMFDA or CMRA dye at a 37 °C incubator for 30 min. Then, the medium was removed by centrifugation, and the cells were washed by fresh medium three times. The stained multicellular spheroids were visualized by an inverted fluorescent microscope (LECIA DMI6000) and a confocal fluorescent microscope (LECIA SP5). For ethylene diamine tetraacetic acid (EDTA; Sigma-Aldrich) treatment, the 4 mM EDTA was added into the medium when cells were cocultured on chitosan. The diameters of multicellular spheroids were analyzed by ImageJ software. For doxorubicin treatment, cells were cultured for 3 d, treated with different concentrations of doxorubicin for another 3 d and examined the mitochondria activities.
The plasmids with N-cadherin short hairpin RNA (shRNA) sequence were purchased from RNAi Core Lab of Taiwan Academia Sinica. The hairpin sequence is GTGCAACAGTATACGTTAATA, which has been ligated into pLKO_TRC005 vectors. The plasmids were transformed into E. coli stbl3. The ViraPower Lentiviral Expression System (Invitrogen) was used to recombine lentiviral vectors. 3A6, SW620 and Hs68 cells were cultured until 60–70% confluence, polybrene (1 mg/ml) and lentiviral vectors were added into the cells, and then the cells were transfected for 24 h. After refreshing the medium, cells were cultured for post-transfection for 3 d. Medium with puromycin (1 μg/ml) was used to screen the transfected cells for 2 w. The cells transfected with no hairpin sequences were mock groups, and the cells without transfection were wild-type groups.
Mitochondria activity assay
The 3–(4,5-dimethylthiazol-2-yl)2,5-diphenyl-tetrazolium bromide (MTT; Sigma-Aldrich) reduction assay was used to evaluate the mitochondria activity. Cells were cultured, and 5 mg/ml MTT was added at the time points. After incubation for 4 h, the medium were removed and the MTT formazans were dissolved by dimethyl sulfoxide. The 570 nm absorbance was detected by the enzyme-linked immunosorbent assay reader (Molecular Devices Spectra Max M2e).
Immune-fluorescence staining
Cells were fixed in 4% formaldehyde solution and permeabilized in 0.1% triton X-100 solution. After blocking in 1% bovine serum albumin (BSA; Sigma-Aldrich) solution, cells were probed with the primary antibody against E-cadherin (BD Pharmingen 610182; 1:50), N-cadherin (BD Pharmingen 610921; 1:100) and P-cadherin (Abcam ab19350; 1:100). Goat anti-mouse IgG H&L DyLight 488 secondary antibody (Abcam ab96871) and 4',6-diamidino-2-phenylindole (DAPI; Sigma-Aldrich) were used to visualize the primary antibodies and the nucleuses of cells. Cells were photographed by the inverted fluorescent microscope.
Western blotting
Cells were lysed with lysis buffer (Roche). Proteins from lysed cells were separated by sodium dodecyl sulfate polyacrylamide gel electrophoresis and then transferred onto nitrocellulose membranes. After blocking in 5% BSA solution, membranes were probed with human primary antibodies E-cadherin (1:5000), N-cadherin (1:2000), P-cadherin (1:1000) and GAPDH (Abcam ab22555; 1:10000), incubated in HRP-conjugated secondary antibodies (Goat anti-rabbit IgG (Abcam ab97051) and Goat anti-mouse IgG (Abcam ab97023)), and visualized by enhanced chemiluminescence (Millipore).
Statistical analysis
All measurements were shown as the mean ± standard deviation from at least 3 independent experiments. Statistical significance was determined using one-way analysis of variance (ANOVA) followed by Duncan’s test. p < .05 and .01 was considered significantly.
Results
The effect of healthy cells to doxorubicin-treated cancer cells
To know whether the cells which existed in tumor microenvironment mediated the drug resistant ability of cancer cells, the mesenchymal stem cells and fibroblasts were cocultured with cancer cells by transwells. To simplify the system, human colon cancer cell line (SW620), human mesenchymal stem-like cell line (3A6), and human foreskin fibroblast line (Hs68) were used for examination in this study. On the transwell experiment, SW620 cells cultured in the top chambers of the transwell inserts have been treated with 1 µM doxorubicin, and the untreated SW620, 3A6, and Hs68 cells were seeded in the bottom chambers of the transwell for 3-day coculture. shows 3A6 and Hs68 cells in the bottom chambers of the transwell could significantly enhance the viability of doxorubicin-treated SW620 cells, compared with SW620 cells or no cells in the bottom chambers for 3-day coculture (p < .05 and p < .01).
Figure 1. The doxorubicin-treated SW620 cells were cocultured with healthy cells by transwell assay. SW620 cells treated with doxorubicin (SW620 (+) were cultured in the top chambers of the transwell for 3 d and then cocultured with other healthy cells (–) cultured in the bottom chambers of the transwell for another 3 d. Then, the SW620 (+) cells in the top chambers were examined by MTT. ** p < .01, * p < .05, n = 4.
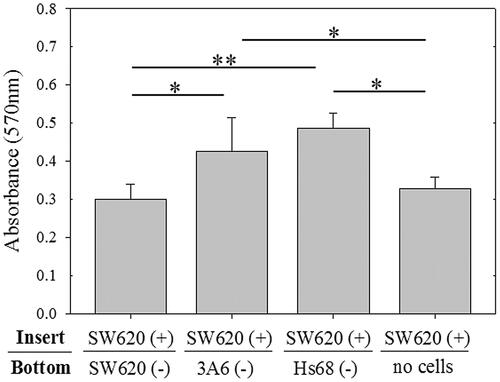
The cadherin protein expression of cells
Different members of the cadherin family have been reported in different cells and locations [Citation43]. Major cadherins such as N-cadherin, E-cadherin and P-cadherin of cells were measured in this study and shown in . Obviously, 3A6 and Hs68 cells expressed the N-cadherin protein, and SW620 cells expressed the E-cadherin and P-cadherin proteins (). Furthermore, the western blot assay confirmed the results (, p < .01).
Cell distribution of multicellular spheroids on chitosan
SW620, 3A6 and Hs68 cells were seeded on chitosan to create suspension coculture system. show they could form suspended spheroids with a core/shell structure when 3A6 and Hs68 cells were cocultured with SW620 cells on chitosan for 3 d. Detailed examination of multicellular spheroids by confocal microscopy shows 3A6 and Hs68 cells could be completely enveloped by the SW620 cells ().
Figure 3. Cell distribution of coculture multicellular spheroids on chitosan for 3 d. (A) Optical images of cell spheroids. Scale bar = 200 um. (B) Inverted microscopic images of cell spheroids. Scale bar = 200 um. (C) Confocal microscopic images of cell spheroids. Scale bar = 50 um.
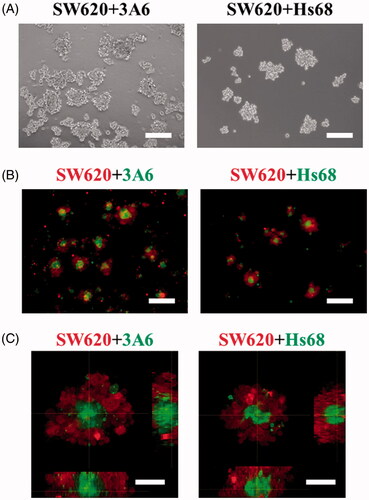
Given that, cells cocultured on chitosan obviously differed in the cell distribution, this study further investigated whether their distribution is seeding order- or cell ratio-dependent. Firstly, one cell type was seeded on chitosan to aggregate for 1 d and then the other cell type was seeded to coculture for another 2 d. We assumed the aggregates formed at the first day should be surrounded by the later seeded cells. However, show that SW620 cells were always in the outer layer to cover the 3A6 or Hs68 cells even SW620 cells aggregate earlier than 3A6 and Hs68 cells. These results suggest that the cell distribution of core/shell structure was not seeding-order dependent.
Figure 4. Cell distribution of multicellular spheroids with different seeding orders and ratios on chitosan. Confocal microscopic images of cell spheroids. (A) The SW620 cells were seeded to aggregate for 1 d, and then the 3A6 or Hs68 cells were seeded to coculture for another 2 d. (B) The 3A6 or Hs68 cells were seeded to aggregate for 1 d, and then the SW620 cells were seeded to coculture for another 2 d. (C) The ratio of cocultured cells was changed from 1:1–1:0.5 and 0.5:1, and the cells were cocultured for 3 d. Scale bar = 50 um.
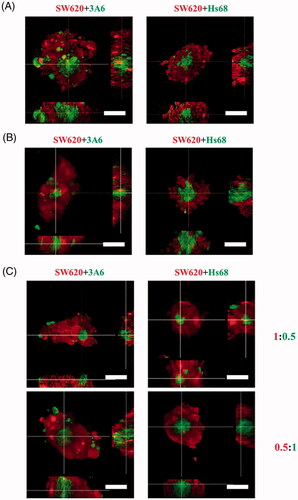
Secondly, the ratio of cocultured cells was changed from 1:1 to 1:0.5 and 0.5:1, which might regulate the probability of cell collision to influence the cell distribution of multicellular spheroids. shows that no matter what the cell ratios were, the coculture cells could show the same core/shell distribution.
The effect of calcium-dependent adhesion molecules on cell distribution
It is known that cadherin plays an important role in calcium-dependent cell adhesion to bind cells together [Citation44]. The EDTA, a calcium chelator, was used to evaluate whether the cell aggregation of multicellular spheroids on chitosan was calcium-dependent. show the size of spheroids decreased significantly in the presence of 4 mM of EDTA (p < .01). The average diameter of SW620 + 3A6 spheroids was 140.6 ± 22.9 μm for the control group without EDTA and 47.8 ± 16.1 μm for the 4 μm EDTA group, and that of SW620 + Hs68 spheroids was 137.7 ± 22.9 μm for the control group and 43.5 ± 13.2 μm for the 4 μm EDTA group. This assured the process of cell spheroids forming on chitosan was calcium-dependent and associated with cadherins.
Figure 5. EDTA treatment of multicellular spheroids and cell distribution of N-cadherin knockdown cells. (A) Optical image of cell spheroids treated with 4 mM EDTA. Scale bar = 200 um. (B) The average diameters of multicellular spheroids treated with (EDTA groups) or without (control groups) 4 mM EDTA on chitosan for 3 d. At least 35 spheroids were calculated for each case, and the data of control groups was analyzed from Figure 3 A. **p < .01. (C) Western blot results of N-cadherin. WT = wild type. NshRNA = N-cadherin shRNA transfected. (D) Confocal microscopic images of cell spheroids. These cells were directly cocultured on chitosan for 3 d. Scale bar = 50 um.
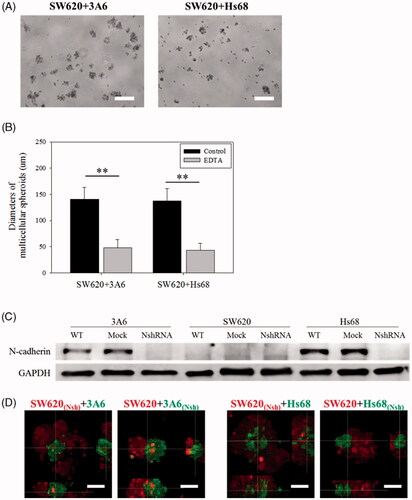
As Hs68 and 3A6 cells always occurred in the core when cocultured with SW620 cells (), we proposed that Hs68 and 3A6 cells possessed stronger interaction to aggregate faster than SW620 cells did. Based on the DAH, we hypothesized that the N-cadherin would be a more major protein than E-cadherin and P-cadherin in this system. To test our hypothesis, the N-cadherin protein of cells was knocked down by the transfection of the N-cadherin shRNA. shows the N-cadherin expression of transfected Hs68 and 3A6 cells was knocked down successfully. Subsequently, transfected cells were cocultured with non-transfected cells on chitosan for 3 d to investigate the effect of N-cadherin on cell distribution. shows SW620 cells transfected with N-cadherin shRNA still completely enveloped 3A6 and Hs68 cells without transfection. These results indicated no effect on the cell distribution when the N-cadherin of expression of the SW620 cells was knocked down. In contrast, when the core 3A6 and Hs68 cells were transfected with N-cadherin shRNA, these cells could not form the core region to be enveloped by non-transfected SW620 cells again. Cocultured cells would be separated to aggregate by themselves, forming attached separate homogeneous spheroids.
The mitochondria activity of core/shell multicellular spheroids
shows the mitochondria activity of SW620, 3A6 and Hs68 cells monocultured on TCPS increased with time, and SW620 cells exhibited the highest mitochondria activity. However, when these cells monocultured on chitosan, only SW620 cells could increase the mitochondria activity with time, and 3A6 and Hs68 cells only maintained very low mitochondria activities during the culture period. This may be the cell viabilities of 3A6 and Hs68 cells was highly related to cell adhesion degree [Citation45], and these two cells could attach onto TCPS but suspend on chitosan. Interestingly, when 3A6 or Hs68 cells cocultured with SW620 cells, the mitochondria activities of whole cells were significantly higher than SW620 cells monocultured on chitosan, even the ratio of 3A6 or Hs68 cells was decreased to 25% of SW620 cells (, p < .01). These results indicated that 3A6 and Hs68 cells located inside the SW620 cell spheroids could support the SW620 cell proliferation with the enhanced mitochondria activities, which might decrease the cytotoxic effect of chemotherapy drugs on SW620 cells.
Figure 6. Mitochondria activity of monocultured, cocultured cells and doxorubicin treated cells. (A) Cells were monocultured on TCPS and chitosan. (B) Cells were cocultured on chitosan. All data were compared with data of SW620 monoculture on chitosan group for statistical analysis. (C) Cells were cultured and treated with doxorubicin for 3 d. SW620_T: SW620 cells were cultured on TCPS; SW620_C: SW620 cells were cultured on chitosan. (D) Cells were cocultured and treated with doxorubicin on chitosan for 3 d. Nsh = N-cadherin shRNA transfected cells. ** p < 0.01, * p < .05, n = 4.
The cytotoxic effect of doxorubicin on core/shell multicellular spheroids
shows the survival curves of SW620 cells monocultured and cocultured with 3A6 or Hs68 cells on TCPS and chitosan after treatment with increasing concentrations of doxorubicin for 3 d. The IC50 (the drug concentration causing 50% inhibition of the mitochondria activity of cells) values were greater than 3 μM for SW620 cells cocultured with 3A6 and Hs68 cells on chitosan, but the values were less than 1.5 μM for other cases. At such concentration, the survival rate of core/shell SW620 spheroids was still greater than 80%, indicating the multicellular spheroid structure might have better survival rates.
shows the difference of the cell survival rate was significant between two different suspended cell structures, two-independent homogeneous spheroids () and core/shell spheroids (p < .01 for 2 μM and p < .05 for 4 μM doxorubicin treatment). Therefore, we proposed that the lower sensitivity of core/shell spheroids to doxorubicin was reasonably attributed to the presence of the 3A6 or Hs68 cells in the core of SW620 cell spheroids.
Discussion
For a living organism, different kinds of cells are responsible for different functions and cooperate with each other to maintain the overall tissue function. Thus, it is impractical to predict cell behaviors in vivo by just considering the response of single type of cells in vitro. To this end, coculture techniques are extensively developed for specific studies recently. In addition to normal tissues, tumor is also considered to be a kind of tissue in a living organism and is composed of many kinds of cells such as cancer cells, cancer stem cells, mesenchymal stem cells, inflammatory cells, cancer-associated fibroblasts and endothelial cells [Citation46,Citation47]. Moreover, mesenchymal stem cells could promote the growth and angiogenesis of tumors [Citation25], and cancer-associated fibroblasts could enhance the proliferation and decrease the drug sensitivity of cancer cells [Citation26–28]. also shows that healthy 3A6 and Hs68 cocultured on the bottom chambers of the transwell could really enhance the viabilities of the doxorubicin-treated SW620 cells in the inserts. These data mean that mesenchymal stem cells and fibroblasts can mediate the nearby cancer cells, and the cancer cells can be stimulated by their microenvironment. Therefore, to figure out the interactions between the cell–cell and the cell–extracellular matrix (ECM) within the tumor tissue, coculture techniques play important roles on cancer-related studies.
Traditionally, cocultured cells were seeded on TCPS wells to form 2D distribution with limited cell contact or were separated by a transwell without contact at all. However, cancer cells form 3D tumor in vivo. Therefore, 3D culture systems are better to build in vitro cancer models for mimicking real tumor tissue than 2D systems [Citation2–4]. In this study, suspension coculture of three human cell lines on chitosan was developed. Based on the characteristic of chitosan, SW620, 3A6 and Hs68 cells with different properties aggregated to form 3D coculture multicellular spheroids with intimate cell contacts ().
As shown, the SW620 mitochondria activities of the healthy 3A6 and Hs68 cultured in the bottom chamber of transwell were higher than that of no cells or healthy SW620 cultured in the bottom chamber. It means, healthy 3A6 and Hs68 could support doxorubicin-treated SW620 for enhancing proliferation. On the other hand, when cells aggregate to form multicellular spheroids, larger spheroids will create a zone of proliferating cells at the outside, a zone of quiescent cells at the middle and a zone of necrotic cells at the core [Citation1,Citation4]. Therefore, based on the conceptual basis of the feeder layers, we developed core/shell coculture multicellular spheroids, which stem cells or fibroblasts aggregated in the core for supporting and cancer cells covered over the shell for being supported. The advantages of these core/shell coculture multicellular spheroids are that the stem cells or fibroblasts would not be harmed by anti-cancer drugs to underestimate the drug resistance of cancer cells, they could support the proliferation of the cancer cells, and they could aggregate at the zone of necrotic cells of multicellular spheroids for avoiding the death of cancer cells.
Previous investigators reported that cadherin mediated the cell–cell interaction to regulate the mutual spreading of heterotypic cells [Citation6,Citation48–50], called DAH. DAH indicated that cells possessed stronger interaction to aggregate faster than other cells did, and the interaction sourced from the cadherin amount of the cells. Therefore, the cadherin expression of these cells was examined next. It was found that the main difference of calcium-dependent adhesion molecules in this study was that 3A6 and Hs68 cells expressed the N-cadherin protein and SW620 cells expressed the E-cadherin and P-cadherin proteins ().
After making sure the cadherin expression of cells, SW620, 3A6 and Hs68 cells were cocultured on chitosan, and they aggregated to form 3D coculture multicellular spheroids (). As anticipation, suspended spheroids on chitosan exhibited core/shell structure with specific arrangement. When cells were cocultured on chitosan for 3 d, 3A6 and Hs68 cells could be completely enveloped by SW620 cells (). In general, to prepare specific cell arrangement, researchers needed to seed different kinds of cells sequentially [Citation51] or used special devices such as micro-patterns, microfluidic channels and so on [Citation38]. Compared with these techniques, chitosan really provided a simple and automatic method to prepare a core/shell spheroid model without much labor intensive operation.
Considering the collision theory, the cell distribution of spheroids in the medium is determined by the cell collision rate and the significant adhesion among cells. The former can be controlled by the cell ratio and the latter is dependent on the intercellular binding ability. In this study, the change in cell ratio, without manipulating the interaction between cells, would not modify the spheroid structure (). More importantly, the cell distribution of core/shell structure was not seeding order dependent (). These results indicate the formation of cell aggregates was not controlled by cell–cell collision as they could not adhere efficiently during cell collision. The cell distribution of core/shell spheroids was determined by the cell–cell interaction.
Based on , we found that Hs68 and 3A6 cells were always occurred in the core when cocultured with SW620 cells. Thus, we proposed that N-cadherin of Hs68 and 3A6 cells might play a more critical role in the formation of core structure in our system. To test our hypothesis, cocultured cells were firstly treated with EDTA to clarify whether the formation of cell spheroids were calcium-dependent (). Then, when the core 3A6 and Hs68 cells were transfected with N-cadherin shRNA, they could not form the core region to be enveloped by non-transfected SW620 cells again. Conversely, no effect was observed on the spheroid reorganization when the N-cadherin of expression of the shell SW620 cells was knocked down (). Thus, the capability of core cells to aggregate and then the shell cells to envelope the aggregate could be regulated by the core cell interaction through the N-cadherin expression.
Cell sorting by cadherins has been proved in embryonic development [Citation52–54]. However, chitosan does not exist during the embryonic development. The role of cadherin in the cell distribution is similar for in vivo and in vitro, but the effect of N-cadherin on the formation of core/shell spheroids cultured on the chitosan-coated substrate is specific in vitro. Why did not E-cadherin or P-cadherin proteins decide the cell distribution of multicellular spheroids when cells cocultured on chitosan? Katsamba et al. have proposed that equivalent N-cadherin possesses higher affinity of homodimers than E-cadherin, and the heterophilic binding affinity of N/E-cadherin is stronger than the homophilic binding affinity of E-cadherin [Citation55]. Cells with higher N-cadherin expression would aggregate firstly, and N-cadherin expressing cells and E-cadherin expressing cells prefer to form instinct or core/shell multicellular spheroids rather than two separate homogeneous multicellular spheroids. Moreover, mixture of equivalent E-cadherin and P-cadherin expressing cells has been proved to create mixed heterogeneous multicellular spheroids as there is little or no difference between these two kinds of cadherins [Citation49]. Therefore, the P-cadherin of SW620 would not affect the cell distribution of core/shell coculture multicellular spheroids in this study.
An in vitro tumor model with 3D cultured cells was proposed by the specificity of adhesion inhibiting of chitosan in this study. In traditional models, the culture of cancer cells on 2D environment was evaluated. There are significant differences between these two kinds of models. For example, every 2D cancer cell is directly exposed to the drug attack from the medium. However, only surface cancer cells of the 3D spheroids are in contact with the medium directly. Drug needs to diffuse through the surface cell layer to interact with the cells in the interior of the spheroids. As shown in , the cell survival rates of SW620 spheroids on chitosan, regardless of coculture or monoculture, were higher than those of monolayered SW620 cells cultured on TCPS. Therefore, the multilayered structure of spheroids would form diffusion resistance to reduce the cytotoxicity of drugs [Citation17].
On the other hand, nutritional supplement needs to be considered for a tumor model. In vivo, angiogenesis is an approach for tumor growth and prevents the necrosis of inner cells [Citation56]. Similar to the design of feeder layer [Citation33], we proposed that the core cells, 3A6 and Hs68, could play the role in supporting the cell viability of the shell SW620 cells in the core/shell coculture multicellular spheroids. As shown in 3A6 or Hs68 cells could enhance the mitochondria activity of SW620 cell when they were located within the SW620 cell spheroids. When the ratio of 3A6 or Hs68 cells was decreased to 25% of SW620 cells, the mitochondria activities of the whole core/shell spheroids were still significantly higher than homogeneous SW620 cell spheroids. However, homogeneous 3A6 or Hs68 cell spheroids on chitosan exhibited very low mitochondria activity during the culture period. Therefore, it is possible that the increased mitochondria activity of core/shell cell spheroids might be attributed by the 3A6 or Hs68 cells to stimulate the SW620 cells but not attributed by 3A6 or Hs68 cells existing.
Finally, we propose that the core/shell structure can increase the resistance against the cytotoxic effect of chemotherapy drugs as on the one hand, core cells can support the mitochondria activity of the shells, and on the other hand core cells can be protected by shell cells under the attack of chemotherapy drugs. As shown in , IC50 values of core/shell multicellular spheroids were obviously greater than that of pure SW620 cell spheroids. further shows the cell survival rate of multicellular spheroids was significantly reduced when the core/cell structure was changed to two attached separate homogeneous cell spheroids. These results assure the lower sensitivity of core/shell spheroids to doxorubicin could be reasonably attributed to the presence of the 3A6 or Hs68 cells in the core of SW620 cell spheroids. Therefore, based on the role of core cells, the coculture core/shell multicellular spheroids on chitosan are potential to be an effective cancer model in vitro.
Conclusion
In summary, chitosan could suspend SW620, 3A6 and Hs68 cells to form 3D core/shell coculture multicellular spheroids. The cell distribution within the spheroids was highly related with their N-cadherin expression. Based on their better cell survival rates when the anti-cancer drug was treated, we proposed the core/shell multicellular spheroids might be an ideal in vitro cancer model for screening drugs or other applications.
Acknowledgments
The authors thank the staff of the Third and Eighth Core Lab, National Taiwan University Hospital and the staff of the imaging core at the First Core Labs, National Taiwan University College of Medicine for technical assistance. This research did not receive any specific grant from funding agencies in the public, commercial or not-for-profit sectors.
Disclosure statement
The authors have declared that no competing interests exist.
References
- Hirschhaeuser F, Menne H, Dittfeld C, et al. Multicellular tumor spheroids: an underestimated tool is catching up again. J Biotechnol. 2010;148:3–15.
- de la Puente P, Muz B, Gilson RC, et al. 3D tissue-engineered bone marrow as a novel model to study pathophysiology and drug resistance in multiple myeloma. Biomaterials. 2015;73:70–84.
- Huang YJ, Hsu SH. Acquisition of epithelial-mesenchymal transition and cancer stem-like phenotypes within chitosan-hyaluronan membrane-derived 3D tumor spheroids. Biomaterials. 2014;35:10070–10079.
- Mehta G, Hsiao AY, Ingram M, et al. Opportunities and challenges for use of tumor spheroids as models to test drug delivery and efficacy. J Control Release. 2012;164:192–204.
- Eetezadi S, De Souza R, Vythilingam M, et al. Effects of doxorubicin delivery systems and mild hyperthermia on tissue penetration in 3d cell culture models of ovarian cancer residual disease. Mol Pharmaceutics. 2015;12:3973–3985.
- Foty RA, Steinberg MS. The differential adhesion hypothesis: a direct evaluation. Dev Biol. 2005;278:255–263.
- Wang K, Kievit FM, Florczyk SJ, et al. 3D porous chitosan-alginate scaffolds as an in vitro model for evaluating nanoparticle-mediated tumor targeting and gene delivery to prostate cancer. Biomacromolecules. 2015;16:3362–3372.
- Xiong G, Flynn TJ, Chen J, et al. Development of an ex vivo breast cancer lung colonization model utilizing a decellularized lung matrix. Integrative Biology: Quantitative Biosciences from Nano to Macro. 2015;7:1518–1525.
- Kas HS. Chitosan: properties, preparations and application to microparticulate systems. Journal of Microencapsulation. 1997;14:689–711.
- Kato Y, Onishi H, Machida Y. Application of chitin and chitosan derivatives in the pharmaceutical field. Cpb. 2003;4:303–309.
- Shi C, Zhu Y, Ran X, et al. Therapeutic potential of chitosan and its derivatives in regenerative medicine. J Surg Res. 2006;133:185–192.
- Archana D, Singh BK, Dutta J, et al. In vivo evaluation of chitosan-PVP-titanium dioxide nanocomposite as wound dressing material. Carbohydrate Polymers. 2013;95:530–539.
- Peng CC, Yang MH, Chiu WT, et al. Composite nano-titanium oxide-chitosan artificial skin exhibits strong wound-healing effect-an approach with anti-inflammatory and bactericidal kinetics. Macromol Biosci. 2008;8:316–327.
- Kerch G. The potential of chitosan and its derivatives in prevention and treatment of age-related diseases. Marine Drugs. 2015;13:2158–2182.
- Tsai CW, Kao YT, Chiang IN, et al. Chitosan treatment delays the induction of senescence in human foreskin fibroblast strains. PloS One. 2015;10:e0140747.
- Tsai CW, Chiang IN, Wang JH, et al. Chitosan delaying human fibroblast senescence through downregulation of TGF-beta signaling pathway. Artificial Cells, Nanomedicine, and Biotechnology. 2017;1–12.
- Phung YT, Barbone D, Broaddus VC, et al. Rapid generation of in vitro multicellular spheroids for the study of monoclonal antibody therapy. J Cancer. 2011;2:507–514.
- Zhang W, Choi DS, Nguyen YH, et al. Studying cancer stem cell dynamics on PDMS surfaces for microfluidics device design. Sci Rep. 2013;3:2332.
- Tsai CW, Young TH. CD44 expression trends of mesenchymal stem-derived cell, cancer cell and fibroblast spheroids on chitosan-coated surfaces. Pure Appl Chem. 2016;88:843–852.
- May JE, Morse HR, Xu J, et al. Development of a novel, physiologically relevant cytotoxicity model: application to the study of chemotherapeutic damage to mesenchymal stromal cells. Toxicology and Applied Pharmacology. 2012;263:374–389.
- Cheng NC, Wang S, Young TH. The influence of spheroid formation of human adipose-derived stem cells on chitosan films on stemness and differentiation capabilities. Biomaterials. 2012;33:1748–1758.
- Chen YH, Wang IJ, Young TH. Formation of keratocyte spheroids on chitosan-coated surface can maintain keratocyte phenotypes. Tissue Engineering Part A. 2009;15:2001–2013.
- Salvatore V, Focaroli S, Teti G, et al. Changes in the gene expression of co-cultured human fibroblast cells and osteosarcoma cells: the role of microenvironment. Oncotarget. 2015;6:28988–28998.
- Ji X, Ji J, Shan F, et al. Cancer-associated fibroblasts from NSCLC promote the radioresistance in lung cancer cell lines. International Journal of Clinical and Experimental Medicine. 2015;8:7002–7008.
- Zhang T, Lee YW, Rui YF, et al. Bone marrow-derived mesenchymal stem cells promote growth and angiogenesis of breast and prostate tumors. Stem Cell Res Ther. 2013;4:70.
- Harati K, Daigeler A, Hirsch T, et al. Tumor-associated fibroblasts promote the proliferation and decrease the doxorubicin sensitivity of liposarcoma cells. Int J Mol Med. 2016;37:1535–1541.
- Shiga K, Hara M, Nagasaki T, et al. Cancer-associated fibroblasts: their characteristics and their roles in tumor growth. Cancers (Basel). 2015;7:2443–2458.
- Li XY, Hu SQ, Xiao L. The cancer-associated fibroblasts and drug resistance. Eur Rev Med Pharmacol Sci. 2015;19:2112–2119.
- Lan SF, Starly B. Alginate based 3D hydrogels as an in vitro co-culture model platform for the toxicity screening of new chemical entities. Toxicology and Applied Pharmacology. 2011;256:62–72.
- Chandrasekaran S, Geng Y, DeLouise LA, et al. Effect of homotypic and heterotypic interaction in 3D on the E-selectin mediated adhesive properties of breast cancer cell lines. Biomaterials. 2012;33:9037–9048.
- Phan-Lai V, Florczyk SJ, Kievit FM, et al. Three-dimensional scaffolds to evaluate tumor associated fibroblast-mediated suppression of breast tumor specific T cells. Biomacromolecules. 2013;14:1330.
- Shang Y, Tamai M, Ishii R, et al. Hybrid sponge comprised of galactosylated chitosan and hyaluronic acid mediates the co-culture of hepatocytes and endothelial cells. J Biosci Bioeng. 2014;117:99–106.
- Celli JP. Stromal interactions as regulators of tumor growth and therapeutic response: a potential target for photodynamic therapy? Isr J Chem. 2012;52:757–766.
- Goers L, Freemont P, Polizzi KM. Co-culture systems and technologies: taking synthetic biology to the next level. Journal of the Royal Society, Interface/the Royal Society. 2014;11:20140065.
- Majety M, Pradel LP, Gies M, et al. Fibroblasts influence survival and therapeutic response in a 3d co-culture model. PloS One. 2015;10:e0127948 Jundoi: ARTN e0127948
- Knight E, Przyborski S. Advances in 3D cell culture technologies enabling tissue-like structures to be created in vitro. J Anat. 2015;227:746–756.
- Jaganathan H, Gage J, Leonard F, et al. Three-dimensional in vitro co-culture model of breast tumor using magnetic levitation. Sci Rep. 2015;4:000342723900001.6468
- Hsiao AY, Torisawa YS, Tung YC, et al. Microfluidic system for formation of PC-3 prostate cancer co-culture spheroids. Biomaterials. 2009;30:3020–3027.
- Hsiao AY, Tung YC, Qu X, et al. 384 hanging drop arrays give excellent Z-factors and allow versatile formation of co-culture spheroids. Biotechnol Bioeng. 2012;109:1293–1304.
- Ivanov DP, Parker TL, Walker DA, et al. In vitro co-culture model of medulloblastoma and human neural stem cells for drug delivery assessment. Journal of Biotechnology. 2015;205:3–13.
- Akasov R, Gileva A, Zaytseva-Zotova D, et al. 3D in vitro co-culture models based on normal cells and tumor spheroids formed by cyclic RGD-peptide induced cell self-assembly. Biotechnol Lett. 2017;39:45–53.
- Tsai CC, Chen CL, Liu HC, et al. Overexpression of hTERT increases stem-like properties and decreases spontaneous differentiation in human mesenchymal stem cell lines. J Biomed Sci. 2010;17:64.
- Takeichi M. The cadherins: cell-cell adhesion molecules controlling animal morphogenesis. Development. 1988;102:639–655.
- Niessen CM, Leckband D, Yap AS. Tissue organization by cadherin adhesion molecules: dynamic molecular and cellular mechanisms of morphogenetic regulation. Physiol Rev. 2011;91:691–731.
- Ruoslahti E, Reed JC. Anchorage dependence, integrins, and apoptosis. Cell. 1994;77:477–478.
- Huang ZJ, Wu TT, Liu AY, et al. Differentiation and transdifferentiation potentials of cancer stem cells. Oncotarget. 2015; Nov 246:39550–39563.
- Krishnamurthy S, Nor JE. Head and Neck Cancer Stem Cells. J Dent Res. 2012;91:334–340.
- Foty RA, Steinberg MS. Cadherin-mediated cell-cell adhesion and tissue segregation in relation to malignancy. Int J Dev Biol. 2004;48:397–409.
- Duguay D, Foty RA, Steinberg MS. Cadherin-mediated cell adhesion and tissue segregation: qualitative and quantitative determinants. Dev Biol. 2003;253:309–323.
- Beysens DA, Forgacs G, Glazier JA. Cell sorting is analogous to phase ordering in fluids. Proceedings of the national academy of sciences of the United States of America; 2000 Aug 15; 9467–71.
- Tseng H, Balaoing LR, Grigoryan B, et al. A three-dimensional co-culture model of the aortic valve using magnetic levitation. Acta Biomaterialia. 2014;10:173–182.
- Derycke LD, Bracke ME. N-cadherin in the spotlight of cell-cell adhesion, differentiation, embryogenesis, invasion and signalling. Int J Dev Biol. 2004;48:463–476.
- Stepniak E, Radice GL, Vasioukhin V. Adhesive and signaling functions of cadherins and catenins in vertebrate development. Cold Spring Harb Perspect Biol. 2009;1:a002949.
- Halbleib JM, Nelson WJ. Cadherins in development: cell adhesion, sorting, and tissue morphogenesis. Genes Dev. 2006;20:3199–3214.
- Katsamba P, Carroll K, Ahlsena G, et al. Linking molecular affinity and cellular specificity in cadherin-mediated adhesion. Proceedings of the National Academy of Sciences of the United States of America; 2009 Jul 11594–11599.
- Iiizumi M, Liu W, Pai SK, et al. Drug development against metastasis-related genes and their pathways: a rationale for cancer therapy. Biochim Biophys Acta. 2008;1786:87–104.