Abstract
Tuberculosis (TB) is a contagious and airborne infectious disease caused by the pathogen Mycobacterium tuberculosis (Mtb). In spite of substantial research efforts, continuous multiple high-dose drug therapy regularly for 4–7 months can impede patient quality of life. The pathology of TB and biology of pulmonary airways permits for a variety of drug delivery strategies and natural route of infection denotes a more logical remedial approach for treatment of TB. Pulmonary delivery is non-invasive, allow easy transcytosis in alveolar region, avoids first-pass metabolism and extensive vascularization facilitates delivery of therapeutic agents to infection site. It also potentially reduces the frequency with dose requirement and linked side effects. Dry powder is a most preferred inhalation option due to their greater physiochemical stability over liquid or suspension based formulations. Dry powder inhalers (DPIs) are easy to handle and appropriate for high-dose formulations. Moreover, the progress of disciplines such as nanotechnology, particle engineering, material science and molecular biology permits further expansion of treatment capability and efficiency. Thus, this article will focus on the role of novel DPIs systems for treatment of TB. This article also contains a dedicated section discussing about technical limitations and clinical challenges with help of strengths, weaknesses, opportunities and threats (SWOT) analysis. Additionally, it will also offer some basic background information for drug repurposing, formulation development and drug delivery systems.
Introduction
Tuberculosis is a contagious and airborne infectious disease, caused by the pathogen Mycobacterium tuberculosis (Mtb). Mtb has been classed as the most hazardous microbial pathogen, killing more individuals than other microbial species [Citation1]. As the humankind began to form denser population hubs and heading to urbanization, Mtb distribute more easily and it became one of the primary causes of death by the beginning of this century [Citation2]. In 2016, the World Health Organization (WHO) reported a global mortality 10.40 million people, of which 6.20 million were men, 3.20 million were women and 1.0 million were children. Furthermore, TB accounted for 64% of the total, with India leading the count, followed by Nigeria, Pakistan and South Africa. Asia (61%) and Africa (26%) were recorded to have the highest incidence of TB estimating one-third of the TB cases globally. Moreover, WHO estimates that there were 0.60 million new cases with resistance to rifampicin the most effective first-line drug of which 0.49 million had multidrug-resistant TB (MDR-TB). Almost half of these cases were in India, China and the Russian Federation. Furthermore, bad resistant TB (XDR-TB) is a severe form of TB that responds to even fewer available medicines [Citation3]. Additionally, the emergence of not curable resistant infection for example, totally drug-resistant TB (TDR-TB), initially noted in Italy more than 10 years ago, rises healthcare concern and the need for new therapeutic tactics [Citation4]. The disease now ranks alongside human immunodeficiency virus (HIV), as the main cause of death from a single infectious disease [Citation3]. Considering the impact of TB on a worldwide scale, it is evident that this disease presents a significant public health challenge.
At present, available chemotherapy contains first-line drugs such as isoniazid, rifampicin, pyrazinamide and ethambutol; second-line drugs such as injectable drugs (streptomycin, amikacin, kanamycin, viomycin and capreomycin), fluoroquinolones (levofloxacin, ofloxacin, moxifloxacin and gatifloxacin) and other oral drugs (cycloserine, ethionamide, prothionamide, para-amino salicylic acid and terizidone) [Citation5]. WHO suggests that TB therapy needs large doses of antibiotics in combination to be taken orally continuously for at least 6 months. Usually, during the initial phase patients receive 3–4 first-line drugs daily for 2 months for a quick amelioration of clinical signs. In the maintenance period, 2–3 drugs are given regularly for 4–7 months to eradicate remaining Mtb cells and control the relapse rate [Citation6]. The second-line drugs are utilized when therapy with first-line drugs not succeed or in occurrence of MDR-TB cases. These molecules are more toxic and unavailable in many growing countries because of high cost. There are several new agents presently in development phase and in clinical trials and recently two new molecules (bedaquiline and delamanid) have been approved for the treatment of MDR-TB, when other alternatives are not available [Citation5]. But both these molecules have been conditionally approved due to severe side effects (hERG toxicity concerns and multiple ADME problems because of their high lipophilicity) have been noted [Citation2]. In addition to above-mentioned restrictions, administration routes possess few serious challenges. The oral route is the most suitable and least expensive; however, extended administration of high doses is required and sub-therapeutic levels of anti-TB drugs reach the site of action because of the hepatic first-pass metabolism, slower onset of action and harsh gastrointestinal environment. The oral route is also coupled with adverse effects as a result of high systemic exposure. Compared to oral route, pulmonary and parenteral routes have highest bioavailability and are not issue to first-pass metabolism [Citation5]. But, preventing repeated painful intramuscular injections mandatory for parenteral drugs is evenly important, especially for small children with little muscle mass and wasted adults. Furthermore, it also necessitates the presence of healthcare workers [Citation7]. In this context, direct lung delivery of anti-TB drugs using pulmonary route could be more advantageous [Citation5,Citation7].
Nebulizers, pressurized metered-dose inhalers (pMDIs), soft mist inhalers (SMIs) and DPIs are extensively scrutinized for the treatment of several lung diseases and various pathological conditions. Among them, DPIs are most preferred option due to their greater physiochemical stability over liquid or suspension based formulations. They do not require refrigeration and are easy to transport. Moreover, powder is dispersed into particles by the patient’s inspiratory flow and usually, the device is economical, hence suitable for TB treatment [Citation8]. Recent advances in nanotechnology, particle engineering and materials science open a door for improving the efficacy of inhalation treatment of different intrapulmonary and extrapulmonary diseases. Various particle engineering techniques are available to produced inhalable particles such as spray drying (SD), freeze drying (FD), ultrasound assisted antisolvent crystallization and high-volume production method, such as electrospinning. In addition to these techniques, various one step and eco-friendly dry powder coating techniques such as mechanofusion, turbo rapid variable, cyclomix and turbula mixer are also available. These techniques are well studied in field of DPIs for improvement of homogeneity, flowability, dispersibility and surface properties. The application of these understanding to the design of DPIs for successful delivery of anti-TB therapeutics purposely to cell and tissues affected by the disease allows for improved treatment outcomes and prevention of severe side effects upon cell and tissues together with those in the lungs and entire organ [Citation8,Citation9].
By knowing this scenario, this review summarizes modern approaches for the inhalation delivery of various anti-TB therapeutics. It illustrates advantages and limitations of inhalation drug delivery and analyses different types of drug delivery systems appropriate for inhalation with a special emphasis on nanotechnology and particle engineering based drug delivery systems. Additionally, review also offers the SWOT analysis for drug delivery systems for better treatment of TB. Finally, it also touches upon the future aspects of inhalation treatment of TB.
Drug repurposing and pulmonary tuberculosis
Drug repurposing is the progression by which well-established drug molecules are used for new applications, frequently involving new administration routes. Repurposing for pulmonary therapy refers to a state when a researcher uses a drug molecule from a previously approved product administered via non inhalation route (e.g. oral or injection) and reformulate it for delivery via pulmonary route (i.e. orally inhaled products). Moreover, delivery of drugs via pulmonary route has many advantages such as low enzymatic activity, easy transcytosis in alveolar region, high permeability, absence of the first-pass metabolism and extensive vascularization. Thus, repurposing of already approved molecules for administration via pulmonary route is more attractive. Good examples of repurposing include both (i) using the pulmonary route as a substitute to deliver systemic drugs without altering their intrinsic therapeutic action (e.g. inhaled insulin for diabetes) and (ii) finding new uses for old molecules (e.g. glycopyrronium and heparin). Glycopyrronium, initially utilized as for a gastrointestinal disorder but now offered in DPI form for treating chronic obstructive pulmonary disease (Seebri Breezhaler®; 44 microgram inhalation powder; Novartis Pharmaceuticals UK Ltd., Camberley). Whereas heparin conventionally utilized for various applications being repurposed for respiratory disorders [Citation10]. Practical challenge stems from the repurposing are listed in Box 1. Drug repurposing allows the option of more quick, less costly and less risky product development, even if the efficacy and safety of the drug when given by a new delivery route still needs to be determined [Citation7,Citation10]. Thus, repurposing of drugs is one of the feasible alternatives in the treatment of pulmonary TB. However, this article will not exclusively deal with the repurposing challenges and disputes in detail, but it will discuss and analyse the current pulmonary drug delivery scenario and situation in the treatment of pulmonary TB.
Box 1 Practical challenge stems from the drug repurposing.
Drug delivery and pulmonary tuberculosis
The pathology of TB and biology of the lungs allows for a variety of approaches for treatment which range from site-specific targeted delivery to non-specific high dose inhalation therapy, each of which has technological, scientific, clinical, regulatory and legal perspectives [Citation7]. The major factors responsible for growing research interests towards inhalation for treatment and prevention of TB are illustrated in Box 2. Inhalation therapy uses the alveolar-capillary interface to attain satisfactory systemic levels whereas minimizing the risk of systemic toxicity noticed with parentally delivered doses. Owing to anatomy of airways and applicable hydrodynamics, only 0.5–5.0 µm size range particles are more appropriate for inhalation therapies. It is generally accepted that particles less than 5 µm in diameter can invade the lungs and that as they approach 1 µm in diameter they have a better tendency to enter to the terminal alveoli or air spaces. Quickly dissolving microparticles are easily dispersed in airways lining fluid and from there will be carried to and through epithelium while bathing the local microenvironment in sufficiently high concentrations of drug. Whereas, slowly dissolving microparticles deposited in the upper airways are accumulated on mucus and washed by action of cilia of epithelial cells and transported to pharynx where they are ingested. Long housing time microparticles that are moved across mucociliary escalator deposit in bronchioles and alveoli where they are exposed to phagocytosis by alveolar macrophages and eventually disposition in molecular state following cell digestion or moved to lymphatics by macrophage migration with help of dendritic cells.
Box 2 Factors responsible for growing research interests towards inhalation for treatment of TB.
In diseased patients, not all airways are uniformly aerated which may restrict penetration of the aerosol microparticles to infected lung regions [Citation7]. Similar to other diseases, TB infected lungs also suffer from morphological variation as a result of growing inflammation, infiltration and oedema. Noteworthy narrowing of pulmonary airways habitually arises with an augmented arbitrary resistance to airflow that fallout in non-uniform particle distribution in infected airways which lowers the amount of drug at the site of action. Normal ventilation is distorted and it has been shown to influence drug delivery in a patient with bronchiectasis and cavitation. Additionally, severe TB infections can alter ventilation supply drastically because of the presence of local barriers and large granuloma lesions. On account of lung parenchyma demolition, elevated turbulence, abridged airflow and cavitation are likely to stimulate proximal deposition of inhaled microparticles [Citation4]. Furthermore, in severe cases of TB, lungs display huge numbers of granulomas and tubercles which leads to necrosis and caseation at their centre. Microparticles that deposits in airways would have to diffuse into these structures to access the pathogen in extracellular or intracellular surroundings [Citation7]. With this background, Novel drug delivery system for pulmonary tuberculosis section described variety of delivery systems that have been studied and analysed for inhaled TB therapy.
Novel drug delivery system for pulmonary tuberculosis
It is interesting to think of inhalation therapy as an innovative strategy for drug delivery, but this technique has been well documented in most of the primeval literatures and it has a sound history of more than 4000 years [Citation11]. From the therapeutic viewpoint, inhalation therapy provides some appealing benefits associated with the anatomical and physiological peculiarities of the pulmonary airways. It is very essential to note that in the pulmonary administration route factors such as solubility, dissolution kinetics, permeation rate through the membranes and bio-stability are less significant [Citation4]. In reality, the pulmonary environment is not more challenging for drugs compared to any other routes mainly the oral one. The low invasiveness of inhalation technique may further improve the patient compliance to the therapy. Furthermore, the growing technological and scientific advancement of formulation and inhaler devices (e.g. Respimat® Soft MistTM Inhaler, Advair Diskus®, Spiriva® HandiHaler®, AirDuo™ Respiclick®) has made inhalation therapy more “patient-friendly” and economically opportune [Citation4,Citation12].
Many different types of delivery systems have been developed and designed for pulmonary delivery of antimicrobials. The majority of these types of delivery systems include lipid vesicles (liposomes and proliposomes), nanoparticles (polymeric nanoparticles (PNs), polymeric micelles, solid lipid nanoparticles (SLNs) and dendrimers), microparticles (microspheres) and nanoparticles embed microparticles (. These inhalation delivery systems are briefly summarized in succeeding section.
Lipid vesicles
Liposomes
Liposomes were first described in 1965 and since then, they are significantly investigated and utilized as a drug carrier from last five decades. They are phospholipid vesicles made up of single or multiple concentric lipid bilayers enclosing aqueous mediums [Citation13]. Generally, a typical liposome (50–450 nm) made up of a single bilayer lipid membrane (unilamellar liposomes) or several bilayer lipid membranes (multilamellar liposomes). They were extensively studied as carrier for the inhalation delivery of synthetic drugs, phytoconstituents, protein and peptides for treatment of various intrapulmonary and extrapulmonary disease and pathological conditions. Additionally, they are most suitable for delivery of drugs to the lungs as they can be formulated from materials endogenous to the lung such as dipalmitoyl phosphatidylcholine (DPPC) and cholesterol. Several existing and novel methods have been investigated for aerosolization of liposomes such as DPIs. DPIs show a satisfactory deposition in the lungs for an extended period of time after inhalation and control release of therapeutic payload inside the cells. Overall, no major side effects were reported after the administration of negatively charged or neutral liposomes. But, cationic liposomes were found to be toxic to human cells and possibly can initiate genetic aberrations. Furthermore, side effects of cationic liposomes remarkably increased with an increase of positive charge of the particles. However, because cationic liposomes usually are utilized for the formation of almost neutral complexes with negatively charged molecules, such adaptation of cationic vehicles generally prevents side effects on the cells [Citation9].
Inhalable rifampicin-loaded liposomes were produced using cholesterol and soybean L-∞-phosphatidylcholine (SPC; 1:1 w/w) by a film hydration method followed by FD with lactose, mannitol and trehalose as a cryoprotectant. Rifampicin multilamellar liposomes showed mean particle size of 255 ± 13 nm and entrapment efficacy of 52 ± 3%. Among the cryoprotectant studied, mannitol was the most appropriate and it was crystalline after being freeze dried. A satisfactory aerodynamic performance was attained with a fine particle fraction (FPF) of 66.8% and an emitted dose (ED) of 100% measured via in-house dry powder inhaler (DPI) device at 60 l/min. Rifampicin in the liposomal DPI was also found to be more stable compared to rifampicin liposomal suspension and rifampicin aqueous solution over the period of one month [Citation14]. Changsan et al. developed rifampicin liposome using SPC by a film hydration method followed by FD with mannitol as a cryoprotectant. Smooth surface multilamellar nanovesicles showed FPF of 60% and a mass median aerodynamic diameter (MMAD) of 3.4 μm. Liposomes did not show any major toxicity sings to alveolar macrophages, bronchial epithelial cells and small airway epithelial. Furthermore, they did not activate alveolar macrophages to produce tumour necrosis factor-α, interleukin-1β and nitric oxide at a level that would lead to inflammatory actions. Rifampicin liposome showed 4-fold reduction in minimum inhibitory concentrations (MIC) against Mycobacterium bovis compared to rifampicin alone [Citation15]. Liposomes are also suitable to encapsulate two or more therapeutic agents in a single liposomal formulation due to their hydrophilic and hydrophobic characteristics [Citation16]. Another more effective drug delivery strategy to treat pathogens harboured in terminal alveoli or air spaces is surface functionalization of the liposomes with targeting ligands and vectors, satisfactorily high bioavailability can be achieved at the site of infection and thus, dose and adverse side effects of drug are minimized. This targeted multifunctional approach not only directs the delivery of drugs into infected organelles but also helps to improve the release kinetic and longevity of drug at terminal alveoli or air spaces [Citation17]. In a study by Bhardwaj et al., mannan attached multiple drug-loaded liposomes were prepared by using thin film hydration technique followed by FD. Combination of rifampicin, isoniazid and pyrazinamide (2:1:1 w/w) used for effective treatment of pulmonary TB. The liposomes showed good entrapment efficiency, particle size and flowability suitable of pulmonary delivery. Liposomes showed controlled release up to 48 h and release profile was best fitted to the Korsmeyer–Peppas equation. Furthermore, after pulmonary administration, mannan-coated liposomes showed satisfactory high lung uptake compared to the free drug at the end of 24 h [Citation18]. Research efforts in this section may lead to develop better delivery systems with superior targeting functionalities.
Proliposomes
Proliposomes were first introduced in 1986 as dry phospholipid formulations and are more stable than liposome dispersions. Afterward in 1991, the idea of proliposomes was expanded to include liquid phospholipid dispersions that can produce liposomes upon addition of aqueous medium. They are of mainly two types (i) particulate-based proliposomes and (ii) solvent-based proliposomes. Particulate-based proliposomes are explained as dry and free-flowing microparticles which can easily form liposomes when in contact with aqueous or biological mediums. Selection of an appropriate carrier is a key factor for formulation of particulate-based proliposomes. The carrier should be chosen on account of porosity and its capacity to accommodate phospholipids and drug moiety on its surface. Compared with conventional liposomal dispersions, proliposomes demonstrate more advantages in stability, solubility and drug release [Citation19]. By knowing this situation, different inhalable proliposomes formulations have been designed and studied to enhance the aerodynamic performance and physical stability of various anti-TB drugs. Various investigations on inhalable proliposomes formulations are summarized in .
Table 1. Proliposomes for the pulmonary delivery of anti-TB therapeutics.
Proliposomes has shown a remarkable therapeutic potential in treatment of TB. As we summarized in , proliposomes was easily formulated using SPC or HSPC with mannitol or lactose as carrier by SD technique. Spray dried proliposomes could improve physicochemical as well as aerodynamic properties of various anti-TB molecules. In comparison to all proliposomes, the HSPC and lactose with leucine (aerosol enhancer) based proliposome have offered a superior improvement in FPF (>90%) for rifapentine. Thus, the combination of anti-TB molecule with judiciously selected lipid moiety and carrier may be an ideal approach to formulate proliposomes with satisfactory aerodynamic performance.
Lipospheres
Lipospheres are self-enclosed small spherical particles composed of solid lipid core enclosed by a phospholipid layer that may entrap, embed or enrich its coat with the therapeutics. They are lipid-based water dispersible solid particles with particle size between 0.01 and 100 μm in diameter. Lipospheres offer some advantages such as easy reconstitution properties, high-drug loading capacity, controlled particle size and drug release kinetics. Due to their unique solid lipid matrix, they can improve bioavailability and physicochemical stability of variety of molecules such as anti-inflammatory agents, local anaesthetics, antibiotics and anticancer molecules. Recently, lipospheres entrapped vaccines are also developed to improve physicochemical properties [Citation24]. Various investigations on inhalable liposphere formulations are summarized in successive section.
Singh et al. developed rifampicin lipospheres using phospholipid (Lipoid S-75) as solid lipid core, sulfphobutyl ether-β-cyclodextrin and Vitamin C by SD technique. Spray dried amorphous lipospheres exhibited spherical shape with the size range of 1–5 μm. During in vitro deposition study, cyclodextrin lipospheres showed 1.21 and 1.27-fold improvement in percentage FPF compared to combined lipospheres and Vitamin C lipospheres, respectively, measured at a flow rate of 28.3 l/min. Furthermore, prepared cyclodextrin lipospheres showed lowest MIC values against Mtb H37Rv strain compared to other lipospheres formulation. Briefly, satisfactory improvement in anti-mycobacterial activity may be accredited to the cyclodextrin-cholesterol matrix structure [Citation25]. Same research group also developed lipospheres using rifampicin and phospholipid (Lipoid S-75) [1:1] by SD method. Rifampicin lipospheres showed particle size, bulk density and specific surface area (4.23 m2/kg) suitable for pulmonary delivery. During in-vitro deposition study, lipospheres showed FPF and MMAD of 77.61% and 2.72 µm, measured at a flow rate of 28.3 l/min. In in-vivo deposition study using nose-only inhalation chamber developed lipospheres showed 8 and 5.31-fold improvement in plasma drug concentration and mean residence time, respectively, compared pure drug. Additionally, lipospheres showed targeting factor >8 compared to pure drug [Citation26]. Thus, lipospheres formulation can be used as an alternative to existing drug delivery systems.
Nanoparticulate system
Nanoparticles are materials having a diameter of 1–100 nm in at least one dimension. The application of nanomaterials to formulation (nano-formulation) offers numerous physical and biological advantages such as improved solubility, better dissolution kinetics, control physiologic clearance rates, reduced systemic toxicity and improved clinical performance. Nanomaterials provide wide variety of delivery options such as PNs, polymeric micelles, dendrimers, SLNs, nanocapsules, nanospheres, nanoemulsion, carbon nanotubes and many more. At this time, they are widely utilized for drug delivery via various delivery routes, including inhalation. The composition, size, shape and surface properties of nanoparticles appreciably influence their dispersion, deposition and retention in the lungs. They can also control the premature mucociliary clearance of drugs. Many of the time, these nanoparticles might be exhaled during administration because of their extremely low mass. Few researchers solve this issue by formulating nanoparticles embed microparticles or microaggregates using SD, spray-freeze-drying or FD with polyols (mannitol, trehalose), force controlling agents (leucine, magnesium stearate) as matrix materials. Nanoparticles embedded microparticles are thoroughly discussed in Nanoparticles embedded microparticles section. Furthermore, surface functionalization of such nanoparticles allows easy targeting of specific cells such as alveolar macrophages [Citation27,Citation28]. Key investigations on inhaled nanoparticle formulations for anti-TB treatment are summarized in below section.
Polymeric nanoparticles
PNs are nanosized, solid, biodegradable colloidal systems with a spherical shape, in which therapeutic agent can be entrapped in core of particles, encapsulated in polymeric matrix (nanospheres) or enclosed by a polymeric layer (nanocapsules). This also makes it feasible for molecule to adsorb or attach to surface of particles. The mean particle diameter of PNs is around 50–500 nm. PNs may be considered the most suitable carriers for drug delivery because of the exceptional features that they present, including their biodegradability and biocompatibility, higher drug loading capacity, controlled drug release properties, good stability and an easy of surface functionalization. Preparation method for these particles is in harmony with their application and drug type. Biodegradable and biocompatible PNs are most preferred because they show compatibility with tissues and cells and can be composed of polymers such as poly(lactic-co-glycolic acid) (PLGA), poly-ε-caprolactone (PCL), poly(lactic acid) (PLA) and chitosan. Inhalable PNs formulations are advantageous for reason of enhancing solubility and dissolution kinetics of water-insoluble anti-TB drugs and reducing early mucociliary clearance of drugs [Citation9,Citation29]. Inhaled PNs might be exhaled during DPI administration because of their extremely low mass. This issue can be solved by formulating nanoparticles into inhalable nanoaggregates. The nanosized aggregates generated by SD showed satisfactory aerosolization performance for isoniazid and rifampin (nano-aggregates) and this was attributed to the porous structure and low density of the nanoaggregates. But, the toxicities of inhaled PNs deserve additional investigations. Key studies on PNs formulations of inhaled anti-TB drugs are listed in .
Table 2. Summary of inhaled nanoparticulate platforms for the treatment of tuberculosis.
Polymeric micelles
Polymeric micelles are the core-shell-type nanostructure formed through self-assembly of block copolymers or graft copolymers in suitable solvents. They are composed of two distinct functional moieties: inner core and outer shell. The inner core is accountable for drug loading, drug release and stability whereas outer shell regulates pharmacokinetic performance. Classic polymeric micelles have a spherical shape with the size is in the range of 20–180 nm. Compared with normal surfactant micelles, polymeric micelles exhibit superior kinetic and thermodynamic stabilities. Apart from its good stability, small size, high solubility, long circulation, targeting and simple production techniques, polymeric micelles system can modify the drug internalization route and subcellular localization; as a result, apply an unique mechanism of action from the entrapped therapeutic agents. Also, polymeric micelles can be formulated from more lipophilic materials to enhance the penetration of encapsulated drug into pathogen and its antibacterial activity against Mtb. Additionally, compared with those more recent nano delivery systems such as liposomes, proliposome, nanoparticles and dendrimers, they possess higher drug loading capacity and improved stability. Because of promising characters, they received fast-increasing scientific interest as a competent drug carrier in recent years [Citation39,Citation40].
In a study by Vadakkan et al., spray dried respirable rifampicin-loaded amphiphilic lipopolymer nano-micelles was formulated in order to control the rifampicin aerodynamic performance and macrophage uptake. The spray dried nano-micelles showed aggregated shape with particle size of <5 µm. A satisfactory high aerosolization efficiency was obtained with a FPF of 67.88% measured via a Rotahaler® device at 28.3 l/min. The developed spray dried nano-micelles showed a biphasic release pattern, initial burst release of ∼50% within 4 h followed by controlled release up to 24 h (87%) in phosphate buffer saline (pH 7.4). During MTT assay on THP-1 (human acute monocytic leukemia cells), almost no cytotoxicity and local inflammatory reaction were discovered. Cell uptake on THP-1 cell lines infecting with GFP tagged Mycobacterium smegmatis, spray dried nano-micelles showed maximum internalization of nano-micelles within 2 h. Briefly, the spray dried nano-micelles formulation was believed a capable delivery system for targeting organism residing within the phagosome of alveolar macrophage [Citation41]. From our understanding, the improved therapeutic activity of these nano-micelles was attributed to a more lipophilic arrangement that enhanced the penetration of the rifampicin into the M. smegmatis.
Solid lipid nanoparticles
SLNs are a new generation of colloidal drug delivery systems compose of surfactant-stabilized lipids that are solid at room and body temperatures. They have a hydrophobic solid matrix core with a phospholipid shell and a mean diameter of 40–1000 nm. They made up of 0.1–30% (w/w) lipid dispersed in an aqueous solution of 0.5–5% (w/w) surfactant as stabilizing agent. They combine the few advantages of emulsions, PNs and liposomes whereas minimizing some of their specific disadvantages. For the pulmonary delivery, SLN has numerous advantages. Better tolerability of SLN in the airways can be assured, if lipids are selected which are biodegradable ensuing in safety, often even endogenous degradation products. Furthermore, because of their size, SLN can be aerosolized into particles with aerodynamically apt properties, which facilitates satisfactory deep lung deposition of a therapeutic agent. In addition, nanosized particles stay to the mucosal surface of the airways for a longer time compared to microparticles. Particle adhesion as well as retention in the airways and controlled release properties of SLN can lead to improved and sustained pharmacological effects and thus result in a better patient compliance [Citation42]. In view of these unique properties, researchers have developed various SLNs based DPI formulations to enhance the therapeutic efficacy of anti-TB drugs ().
Dendrimers
The phrase dendrimer (from a Greek word Dendron-tree) typically represent a highly branched structure. Dendrimers are cyclically branched tree-like architecture. Such tree-like design represents a spherical shape to dendrimers after numerous layers of branching because of surface congestion. Every branching layer is called as a generation. Simply, dendrimers present distinct structural design with spherical shape, manifold surface functionalities and high degree of molecular uniformity. They can be used in various biomedical areas such as drug delivery, biomedical engineering and diagnostics. When compared to conventional polymers for drug delivery, dendrimers showed distinct advantages such as high drug-loading capacity at the interior space for entrapment or at surface terminal for conjugation, multivalency for conjugation to therapeutic agents, biopolymers, molecular sensors and targeting moieties and additionally precise size control with distinct numbers of peripheries. These unique geometrical features distinguish dendrimers from other traditional polymers. They can be used for variety of therapeutic applications for various diseases such as cancer, inflammatory and infectious diseases [Citation43]. Considering these unique geometrical features and therapeutic properties, few researchers have developed dendrimers based aerosol to enhance the therapeutic efficacy of anti-TB therapeutics. These research efforts are described in following segment.
Rajabnezhad et al. developed co-spray dried inhalable microspheres using different generations polyamidoamine dendrimers (G1, G2 and G3) and rifampicin as a model anti-TB molecule. The G3-rifampicin microspheres showed the smallest particle size (6.21 μm) and bulk density (0.024 g/ml) compared to other two generations. During in-vitro deposition study, G1-rifampicin microspheres achieved a highest FPF of 54%, measured using the Spinhaler® device at a flow rate of 60 l/min. Higher bulk density, less porous microstructure and sufficient electric charge are responsible for higher in-vitro G1 deposition efficacy. G3-rifampicin microspheres showed slow and controlled drug release up to 72 h at pH 7.4 compared to other two generations at same dose (5 mg). Furthermore, interestingly, after pulmonary insufflation, G3-rifampicin microspheres exhibited 30 and 4-fold improvement in mean residence time and plasma drug concentration compared to intravenous administration of drug alone. Simply, polycationic dendrimer-based microspheres can play important role in the pulmonary delivery of rifampicin like therapeutic agents [Citation44].
Nanoparticles embedded microparticles
Nanoparticle-based delivery systems show various therapeutic advantages as mentioned in above section. Drug-loaded lipid or polymer nanoparticles are normally formulated by solvent-based techniques and form colloidal suspensions which frequently suffer from physical and chemical instability resulting in loss of functionality. There are numerous techniques to stabilize such nanoparticle-based formulations including addition of surfactant to creating a steric barrier between the particles or ionic materials to offer electrostatic repulsion. However, for better and long-term stability often water needs to be removed to obtain a stable dry product. For this reason, various methods such as SD, spray freeze-drying and FD are commonly utilized to remove water from the nanoparticle-based formulations and to shape customized powder products i.e. nano-embedded microparticles (NEMs). NEMs act as an efficient carrier for stabilization and delivery to their proposed site of action. Sugars such as lactose, mannitol and trehalose are most commonly used to formulate the surrounding and stabilizing matrix. Additionally, these NEMs carriers are compatible with a wide range of therapeutic agents for different routes of administration [Citation45]. NEMs exhibit a capable approach to deliver nanoparticles to the pulmonary system with suitable aerodynamic properties they allow an application with DPI. After deposition, nanoparticles can be released to show their therapeutic advantages such as a controlled drug release and delivery of the drug across the mucus barrier. The selection of an apt excipient to embed the nanoparticles is essential for the satisfactory deposition and sustained release of nanoparticles [Citation28,Citation46]. By knowing this context researchers investigated various NEMs based DPIs for effective delivery of anti-TB drugs ().
Table 3. Respirable nanoparticles embedded microparticles along with the key findings.
Nano-aggregates
Nano-aggregates are simply described as a cluster of nanoparticles. Together with other particulate and vesicular delivery systems, nano-aggregates have recently appeared as a new supramolecular colloidal carrier with promising physicochemical and biological properties. They are shaped from a polymer-drug conjugated amphiphilic block copolymer. They are more suitable for entrapment of poorly water-soluble drugs by physical entrapment and covalent conjugation. Due to physical entrapment, a maximum amount of drug can be loaded in nano-aggregates, which helps to attain an adequately high drug concentration at the target site. Furthermore, they offer few inherent properties such as size in the nanometers and satisfactory in-vivo stability. They can also provide site-specific drug delivery using either an active or passive targeting mechanism. Active transport can be attained by conjugating a drug with specific ligands or vectors that bind particularly to receptors being overexpressed in the diseased cells [Citation50]. By knowing this scenario, inhalable nano-aggregates formulations have been designed and investigated to enhance the solubility, bioavailability and physical stability of anti-TB therapeutic agents.
In a study by Kaur et al., guar gum-based porous nanoaggregates were formulated by anti-solvent precipitation technique followed by SD with two frontline ant-TB molecules i.e. isoniazid (I-GGN) and rifampicin (R-GGN). Spherical and uniform nanoaggregates showed aerodynamic diameter of 3.53 µm (I-GGN) and 3.11 µm (R-GGN) with entrapment efficiency of 51.3% (I-GGN) and 50.4% (R-GGN). Both nanoaggregates showed biphasic release profile in a methanolic phosphate buffer saline (pH 7.4). However, I-GGN and R-GGN showed an initial burst release of 20 and 5% at end of 1 h, respectively. A relatively slow release of rifampicin could be credited to (i) hydrophobic interaction of guar gum and rifampicin and (ii) low drug solubility in the selected dissolution medium. In pharmacokinetic study, after pulmonary administration I-GGN and R-GGN showed 5.38 and 7.13-fold improvement in AUC(0–24), respectively, compared to individual drug alone. Furthermore, pulmonary administration of I-GGN and R-GGN is not only effective in quick attainment of high drug concentrations in alveolar macrophages but also sustain the concentration over a prolonged period of time compared to individual drug alone [Citation51]. Another research group Sung et al. formulated rifampicin loaded PVA-based porous nano-aggregates by solvent precipitation technique followed by SD. Thin-walled structured nano-aggregates showed volume median diameter of 4.2 μm with drug content and zeta potential of 13.5% and −33 mV, respectively. Porous nanoaggregates showed biphasic release profile in a phosphate buffer (pH 7.2). Spray dried powder with 40% (PNAP 40) and 80% (PNAP 80) of nanoaggregates showed FPF of 35.5 and 44.7%, respectively. In pharmacokinetic study, after intratracheal insufflation (2.5 mg/kg) PNAP 40 and PNAP 80 showed 4.23 and 3.11 fold improvement in bioavailability compared to oral suspension administration at same dose [Citation52]. This delivery system has the good potential to provide therapeutic advantages for diseases where both local and systemic treatment of the lungs may improve treatment, such as TB.
Numerous researchers have taken lots of efforts in designing and developing respirable nanoparticles for improved anti-TB therapy. Inhaled nanoparticulate consisting of anti-TB drugs have been successfully prepared by using various biocompatible and biodegradable lipid and polymers such as chitosan and PLGA. Between all excipient, PLGA is most commonly used polymer and have been utilized to entrap, embed or encapsulate the variety of anti-TB molecules. Among the prepared inhaled nanoparticulate, third generation polyamidoamine rifampicin dendrimers showed better mean residence time (30-fold) compared to pure drug alone after pulmonary insufflation while isoniazid NEMs prepared using antisolvent precipitation showed highest pulmonary bioavailability (>16 fold) compared to pure drug alone after pulmonary insufflation. Whereas, ethionamide PLGA nanoparticles prepared using solvent evaporation showed better FPF (>94%) at flow rate of 28.3 l/min. Still, few questions have to be answered to use full potential of inhaled nanoparticulate. The existing encapsulating excipients are limited to few lipids and biodegradable polymers e.g. poly(lactide-co-glycolide), which are agreed by the US FDA for parenteral delivery. However, no inhaled formulation containing polyester polymers has yet obtained marketing sanction, but drug master files for these materials have been submitted. Additionally, the use of nanoparticles is considered as harmful because of the indistinct effect that nano systems can exercise on lung tissues and their outcome if absorbed. All these aspects partially contribute to the slow progress of inhaled nanoparticles based antimicrobial development [Citation4]. However, the budding commitment of researcher and research organizations in developing inhaled nanoparticle-based antimicrobial and the technological advancement in manufacturing of DPIs could be the entry to the approval of new nanoparticle inhalation medicines for infectious disease like TB.
Microparticulate system
The phrase “microparticle” in drug delivery applications commonly refers to a particle with one or several micrometres in size. Microparticles are described as small particles, with size in the range of 1–1000 μm. They contain many materials like natural/synthetic polymers, polysaccharides, metals, ceramics and glass. Microparticles can be easily formulated using various biodegradable and biocompatible polymers such as chitosan, alginate, eudragits, PLGA, PLA, polystyrene and polyethylene polymers. Based upon the method of preparation, the drug is encapsulated, entrapped or simply dissolved to the microparticle template. Generally, they are porous and have good capacity for the absorption of a wide variety of hydrophobic and hydrophilic molecules. The recent progress in field of drug delivery in which the microparticles are decorated with various ligand and vectors assists targeted drug delivery. In clinical practices, microparticles significantly control drug dispersion, deposition and retention in the lungs apart from these facts microparticles also offer several benefits such as facilitation of easy handling, controlled dissolution profile, better stability profile and active targeting also [Citation9,Citation27]. By knowing this scenario, various investigations on microparticulate formulations of inhaled anti-TB drugs are listed in .
Table 4. Summary of inhaled microparticles formulations for the treatment of tuberculosis.
Microspheres
Microspheres are explained as small, microscopic hollow sphere, with size in the range of 1–1000 μm. Microparticle and microspheres both are submicron size particles but major difference among them is their shape. As stated above, microspheres are more spherical in shape. In addition to particle size, charge and density and particle shape also play an important role in drug delivery. Particle shape has a significant influence on the final performance of microparticle as a carrier of therapeutic moiety by affecting their fate not only in the in vitro but also in in-vivo studies. The particle shape and surface is important when powder flow is crucial to the performance of the product, for example, in the case of DPI dosage forms [Citation69]. Microspheres are perfect vehicles for several controlled delivery systems owing to their ability to encapsulate a variety of therapeutic agents, prolonged/sustained drug release characteristics, high bioavailability and good biocompatibility. Such systems also present a variety of benefits over conventional delivery systems, including protection of thermo-labile and fragile molecules, modifying of drug release kinetics and improved patient compliance [Citation9]. Microspheres based anti-TB DPI formulations are listed in .
Table 5. Microspheres for the pulmonary delivery of anti-TB therapeutics.
The existing literature regarding microparticulate drug delivery designing and development for effective TB therapy represents notable progress over the last few years. Satisfactory aerodynamic performance of microparticulate systems depends on four mutually dependent features: shape, size, surface and porosity. SD and FD are two most preferred methods to fabricate microparticulate formulations whereas PLGA and chitosan are two extremely common polymer in microparticulate systems. Microparticles produced by SD shown to have superior aerodynamic properties and fewer formulation challenges, it is a simple and straightforward method with the potential of a larger scale production. As reported in present literature, smooth surface spherical shape microparticles or microspheres showed satisfactory aerodynamic performance. Between the prepared inhaled microparticles, spray dried rapamycin PLGA microparticles displayed better-controlled release (10 d) and satisfactory inhibition of intracellular mycobacteria compared to pure drug alone while ofloxacin hyaluronan microspheres showed highest pulmonary bioavailability (10.9 fold) compared to iv administration at same dose (8 mg/kg). In a nutshell, prepared microparticles or microspheres offer a suitable delivery platform for inhalation, since they are prepared to form biodegradable ingredients and easily scalable techniques.
Combination therapy
MDR organisms habitually acquire resistance through a number of distinct resistance mechanisms. A single antimicrobial agent is not competent to deactivate or avoid every distinct resistance mechanism [Citation80]. Combination drug delivery strategies that unite antibiotics targeting different pathways are one of the suitable approaches for creating multidrug combinations to prevent antibiotic resistance. Combination of two or more antimicrobial agents represents a suitable approach to neutralize resistance developed by MDR organisms. Additionally, mixture of antimicrobial agents might offer synergistic bactericidal action at lower dose with better acceptability and safety. In spite of the administration route, numerous useful combinations that target different pathways are not strictly limited to antibiotics but extend to combinations with non-antibiotic supportive. Numerous non-antibiotic combinations have been investigated, including, combining antibiotics with mucolytic agents (e.g. dornase alfa) and combining antibiotics with force controlling agents (e.g. magnesium stearate and leucine). Thus, administrating antimicrobial combinations to control MDR has turn into regular policy with systemic agents and few researchers studied and apply it to inhaled antibiotics [Citation80,Citation81]. Till date numerous such combinations have been investigated, summary of reports of inhaled anti-TB combinations are listed in .
Table 6. Combined inhaled microparticles for the treatment of tuberculosis.
Inhaled protein, peptides and vaccine
Numerous therapeutic proteins, peptides and vaccine have been utilized in day-to-day clinical practice. More than 60 proteins and peptides based formulations are approved across the globe and continue to enter into various clinical phases [Citation88]. To name few, insulin to treat diabetes, adrenocorticotropic hormone (a polypeptide tropic hormone) used as diagnostic agent, human growth hormone (hGH) to supplement hormone deficiency, leuprorelin to treat prostate and breast cancer as well as calcitonin (linear polypeptide hormone) and parathyroid hormone (PTH) to treat osteoporosis. Because of the recent advances in molecular/cell biology and biotechnology with better understanding of the physiopathology of many diseases, cellular components are expected to become of growing importance. But a major problem of these components is the need to use parenteral route for administration. This is primarily because of the existence of various proteases enzymes and harsh gastrointestinal environment, leading to physico-chemical degradations and consequently loss of therapeutic activity. Pulmonary delivery of these therapeutic components could represent an option to parenteral delivery. Direct administration to the pulmonary airways for local treatment permits high doses of protein or peptides drugs to be delivered while preventive systemic adverse side effects. Pulmonary delivery can be utilized to provide systemic action of few proteins and peptides. Furthermore, some enzymes present at the thin epithelial membrane and the air-lung interface allow permits effective absorption of inhaled biomolecules. But, administration of proteins or peptides to the pulmonary airways represents few major challenges. An initial problem with any inhaled therapeutics is the need to supply the drug as solid or liquid microparticles (<5 µm) to reach the pulmonary airways. Another important problem, which is specific to inhaled proteins and peptides, is that they can experience physicochemical degradation during handing or tailoring microparticles such as, exposure to extreme pH, temperatures and diverse stresses. These physicochemical degradations can lead to loss of therapeutic activity. Another concern with these biomolecules is their large molecular weight, hydrophilicity of proteins and pulmonary biological fate [Citation89–91]. To surpass these all issues, various researchers tried and tested different strategies for pulmonary delivery of proteins and peptides. An overview of these strategies along with their key achievements is listed in .
Table 7. Respirable protein and peptides microparticles along with the key findings.
Device selection
Selecting the most appropriate inhaler device can be the gateway to successful TB treatment. Pathophysiology of every disease is distinct likewise outcome and manoeuver of every inhaler device are different [Citation94,Citation95]. Therefore, cautious consideration has to be given in selecting the right inhaler device for treatment of TB. Patient inspiratory flow rate, inhaler device resistance, patient compliance, device handling training, reliable and safe dosing are the main five key points we have to consider before selecting any inhaler device [Citation94,Citation95]. At present, various inhaler devices with unique geometry, design and technology are available in market for drug delivery to pulmonary airways [Citation96]. Different types of inhaler devices available in market are disposable inhalers devices (e.g. Cricket®, Twincer® and Occoris®), single-dose capsule-based inhaler devices (e.g. HandiHaler®, Breezhaler® and Aerolizer®), pre-metered multi-dose blisters based inhaler devices (e.g. Diskus® and Ellipta®) and reservoir based multi-dose inhaler devices (e.g. Respiclick®). In a nutshell, by understanding the patient capability and compliance we have to choose apt inhaler device for TB treatment.
Existing clinical status
For successful pulmonary TB therapy, drug should reach in deep lungs and inhaler devices are competent to delivery satisfactory dose to deep lungs. Furthermore, the various preclinical trials have shown optimistic results but there is a question allied to safety in human beings [Citation96]. Clinical trials are the key basis of evidence-based treatment and accordingly building the backbone of clinical practice [Citation97]. Various clinical trials related to “pulmonary tuberculosis” are registered and accessible on US FDA portal (ClinicalTrials.gov [https://clinicaltrials.gov/]). More precisely, presently, worldwide 217 clinical trials are registered for various molecules ranging from first line antibiotics to newly developed monoclonal antibodies for treatment of TB. Whereas in Europe (EU Clinical Trials Register [https://www.clinicaltrialsregister.eu/]), presently 53 clinical trials are registered under “pulmonary tuberculosis” title. But among them, only few studies are registered for inhalation based TB treatment. Still, a number of clinical trials have to perform for a wide range of antibiotics molecules.
Discussion
In spite of the truth that TB infection is a historic ailment, up to date >10 million peoples suffer; most of them are in the developing nations. It is a leading global public health affair, resulting in a heavy socio-economic and healthcare burden. TB killed more individuals than any other single transmissible agent, but financial support for research to develop better treatment, prevention and diagnosis techniques for TB failed to its lowest level in past few years [Citation98]. Thus, investigation in this area is still at the exploratory segment.
Needless to say but achieving sufficiently high drug concentration and targeting drug to alveolar region is still very challenging. Ahead of the complex host-pathogen relationship and lungs morphology, it should be kept in mind that patient’s age, breathing pattern, optimized formulation properties, device performance and features determine the real fate of aerosol and the final success of inhaled therapy. Simply, the successful delivery of any therapeutic molecules to the pulmonary airways using aerosol depends on four mutually dependent aspects: the formulation, the inhaler device, the metering system and the patient’s understanding/awareness. Till date plenty of articles have talked about novel formulation development strategies, pharmacokinetic outcomes and toxicological effects but merely touch issues related to device (. However, there is an equally fascinating story to be told regarding the inhaler devices, device designing, functioning, metering system and patient’s training.
Figure 2. Number of scientific publications on inhalable dry powder formulations for the treatment of TB; (A) Variety of novel dry powder formulation prepared till date and (B) Experimental efforts taken by researchers to evaluated novel formulations.
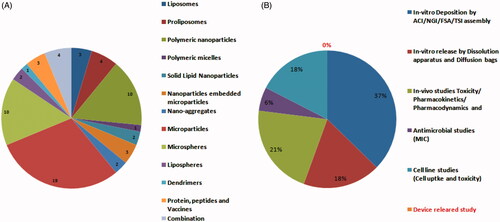
As we know, DPIs are passive inhaler devices, their end performance in terms of FPF and drug deposition pattern highly depends on the patient’s capacity to inhale an adequate volume of air at the preferred inspiratory flow rate. So as to guarantee optimal performances, development of the device and formulation should be determined and considered as a whole medicinal product. Device makeup and actuation technique should take into account patient’s age, overall health status and mainly patient’s lung capacity. Moreover, dose metering technique has great significance for DPIs since the dose needed for effective clinical performance of antimicrobial is too large to be inhaled in a single actuation. Thus, we need a strong teamwork between formulation scientists and device engineers to accomplish optimization of the complex device/formulation correlation. Computational and simulation tools like computational fluid dynamics (CFD) is now commonly utilized across all branches of science, engineering and technology and thus can be used effectively in analysing and predicting the mechanisms of dispersion and deagglomeration of DPIs [Citation8]. Even now, numerous questions have to be solved during development of anti-TB molecules for pulmonary delivery. Despite, notable progresses in imaging methods, measuring and correlating aerodynamic drug deposition with clinical efficacy is not easy. Apart from developing and designing novel delivery systems, we should carefully consider few factors such as nature of infection and drug prescribe effectiveness of therapy and treatment failure rate, judiciously assessment of cost and compliance. Thus, SWOT analysis for inhaled anti-TB molecules is described in .
Conclusion
As we identified, there is a clear need to continue the push to design, develop and systematically validate novel pulmonary formulations to treat TB infections. The real success of effective TB therapy based on the capability to attain high therapeutic concentration in the terminal alveoli or air spaces of lungs without offering drug systemically at a concentration that supports the development of drug-resistant microorganisms. The researchers from academic and pharmaceutical organization are now eagerly waiting for the successful translation of inhaled delivery systems to the marketplace as feasible option in terms of therapeutic perspective and cost-effectiveness for developing countries. Finally, the next development of pulmonary TB therapy, significantly remains in the following segments (i) improved understanding about Mtb and its life cycle to design better drugs and deliveries, (ii) good knowledge about device/formulation interface, (iii) selection and development of better animal infection models that mimic the pathology of the infected human lung, (iv) regulatory and CMC guidelines for better evaluation of novel pulmonary formulations and (v) up to date information about pulmonary pharmacokinetics and toxicity. In conclusion, we believe there is great strength in the present expertise, experience gained by them and diversity of researchers presently working in this area, which needs to be nurtured to persist the important battle against tuberculosis.
Disclosure statement
No potential conflict of interest was reported by the authors.
References
- Chetty S, Ramesh M, Singh-Pillay A, et al. Recent advancements in the development of anti-tuberculosis drugs. Bioorg Med Chem Lett. 2017;27:370–386.
- Hoagland D, Liu J, Lee R, et al. New agents for the treatment of drug-resistant Mycobacterium tuberculosis. Adv Drug Deliv Rev. 2016;102:55–72.
- WHO, Tuberculosis. Global tuberculosis report. Geneva, Switzerland: WHO; 2017.
- Giovagnoli S, Schoubben A, Ricci M. The long and winding road to inhaled TB therapy: not only the bug’s fault. Drug Dev Ind Pharm. 2017;43:347–363.
- Costa A, Pinheiro M, Magalhaes J, et al. The formulation of nanomedicines for treating tuberculosis. Adv Drug Deliv Rev. 2016;102:102–115.
- Parumasivam T, Chang RY, Abdelghany S, et al. Dry powder inhalable formulations for anti-tubercular therapy. Adv Drug Deliv Rev. 2016;102:83–101.
- Hickey A, Durham P, Dharmadhikari A, et al. Inhaled drug treatment for tuberculosis: past progress and future prospects. J Control Release. 2016;240:127–134.
- Mehta P. Imagine the superiority of dry powder inhalers from carrier engineering. J Drug Deliv. 2018;2018:1–19.
- Mehta P. Dry powder inhalers: a focus on advancements in novel drug delivery systems. J Drug Deliv. 2016;2016:1–17.
- Lyapustina S. Regulatory pitfalls and opportunities when repurposing for inhalation therapy. Adv Drug Deliv Rev.
- Sanders M. Inhalation therapy: an historical review. Prim Care Respir J. 2007;16:71–81.
- Zhou Q, Tang P, Leung S, et al. Emerging inhalation aerosol devices and strategies: where are we headed? Adv Drug Deliv Rev. 2014;75:3–17.
- Bardania H, Tarvirdipour S, Dorkoosh F. Liposome-targeted delivery for highly potent drugs. Artif Cells Nanomed Biotechnol. 2017;45:1478–1489.
- Changsan N, Chan H, Separovic F, et al. Physicochemical characterization and stability of rifampicin liposome dry powder formulations for inhalation. J Pharm Sci. 2009;98:628–639.
- Changsan N, Nilkaeo A, Pungrassami P, et al. Monitoring safety of liposomes containing rifampicin on respiratory cell lines and in vitro efficacy against Mycobacterium bovis in alveolar macrophages. J Drug Target. 2009;17:751–762.
- Akhter M, Rizwanullah M, Ahmad J, et al. Nanocarriers in advanced drug targeting: setting novel paradigm in cancer therapeutics. Artif Cells Nanomed Biotechnol. 2018;46:873–884.
- Lemmer Y, Kalombo L, Pietersen R, et al. Mycolic acids, a promising mycobacterial ligand for targeting of nano encapsulated drugs in tuberculosis. J Control Release. 2015;211:94–104.
- Bhardwaj A, Kumar L, Narang R, et al. Development and characterization of ligand-appended liposomes for multiple drug therapy for pulmonary tuberculosis. Artif Cells Nanomed Biotechnol. 2013;41:52–59.
- Khan I, Elhissi A, Shah M, et al. Liposome-based carrier systems and devices used for pulmonary drug delivery. Biomaterials and medical tribology research and development. Sawston, Cambridge: Woodhead Publishing Series in Biomaterials; 2013. p. 395–443.
- Rojanarat W, Changsan N, Tawithong E, et al. Isoniazid proliposome powders for inhalation-preparation, characterization and cell culture studies. Int J Mol Sci. 2011;12:4414–4434.
- Rojanarat W, Nakpheng T, Thawithong E, et al. Inhaled pyrazinamide proliposome for targeting alveolar macrophages. Drug Deliv. 2012;19:334–345.
- Rojanarat W, Nakpheng T, Thawithong E, et al. Levofloxacin-proliposomes: opportunities for use in lung tuberculosis. Pharmaceutics. 2012;4:385–412.
- Patil-Gadhe A, Pokharkar V. Single step spray drying method to develop proliposomes for inhalation: a systematic study based on quality by design approach. Pulm Pharmacol Ther. 2014;27:197–207.
- Dudala T, Yalavarthi P, Vadlamudi H, et al. A perspective overview on lipospheres as lipid carrier systems. Int J Pharm Investig. 2014;4:149–155.
- Singh C, Koduri L, Singh A, et al. Novel potential for optimization of antitubercular therapy: pulmonary delivery of rifampicin lipospheres. Asian J Pharm Sci. 2015;10:549–562.
- Singh C, Koduri L, Dhawale V, et al. Potential of aerosolized rifampicin lipospheres for modulation of pulmonary pharmacokinetics and bio-distribution. Int J Pharm. 2015;495:627–632.
- Kuzmov A, Minko T. Nanotechnology approaches for inhalation treatment of lung diseases. J Control Release. 2015;219:500–518.
- Zhou Q, Leung S, Tang P, et al. Inhaled formulations and pulmonary drug delivery systems for respiratory infections. Adv Drug Deliv Rev. 2015;85:83–99.
- Rawal T, Parmar R, Tyagi R, et al. Rifampicin loaded chitosan nanoparticle dry powder presents an improved therapeutic approach for alveolar tuberculosis. Colloids Surf B Biointerfaces. 2017;154:321–330.
- Noraizaan A, Wong T. Physicochemical effects of lactose microcarrier on inhalation performance of rifampicin in polymeric nanoparticles. Powder Technol. 2017;310:272–281.
- Garg T, Goyal A, Rath G, et al. Spray-dried particles as pulmonary delivery system of anti-tubercular drugs: design, optimization, in vitro and in vivo evaluation. Pharm Dev Technol. 2016;21:951–960.
- Pourshahab P, Gilani K, Moazeni E, et al. Preparation and characterization of spray dried inhalable powders containing chitosan nanoparticles for pulmonary delivery of isoniazid. J Microencapsul. 2011;28:605–613.
- Bhardwaj A, Mehta S, Yadav S, et al. Pulmonary delivery of anti-tubercular drugs using spray-dried lipid-polymer hybrid nanoparticles. Artif Cells Nanomed Biotechnol. 2016;44:1544–1555.
- Debnath S, Saisivam S, Omri A. PLGA ethionamide nanoparticles for pulmonary delivery: development and in vivo evaluation of dry powder inhaler. J Pharm Biomed Anal. 2017;145:854–859.
- Ahmad M, Ungphaiboon S, Srichana T. The development of dimple-shaped chitosan carrier for ethambutol dihydrochloride dry powder inhaler. Drug Dev Ind Pharm. 2015;41:791–800.
- Debnath S, Saisivam S, Debanth M, et al. Development and evaluation of Chitosan nanoparticles based dry powder inhalation formulations of Prothionamide. PLoS One. 2018;13:e0190976.
- Maretti E, Rustichelli C, Romagnoli M, et al. Solid Lipid Nanoparticle assemblies (SLNas) for an anti-TB inhalation treatment-A design of experiments approach to investigate the influence of pre-freezing conditions on the powder respirability. Int J Pharm. 2016;511:669–679.
- Maretti E, Costantino L, Rustichelli C, et al. Surface engineering of Solid Lipid Nanoparticle assemblies by methyl α-d-mannopyranoside for the active targeting to macrophages in anti-tuberculosis inhalation therapy. Int J Pharm. 2017;528:440–451.
- Afsharzadeh M, Hashemi M, Mokhtarzadeh A, et al. Recent advances in co-delivery systems based on polymeric nanoparticle for cancer treatment. Artif Cells Nanomed Biotechnol. 2018;46:1095–1110.
- Pawar A, Rajalakshmi S, Mehta P, et al. Strategies for formulation development of andrographolide. RSC Adv. 2016;6:69282–69300.
- Vadakkan M, Annapoorna K, Sivakumar K, et al. Dry powder cationic lipopolymeric nano micelle inhalation for targeted delivery of antitubercular drug to alveolar macrophage. Int J Nanomedicine. 2013;8:2871–2885.
- Weber S, Zimmer A, Pardeike J. Solid Lipid Nanoparticles (SLN) and Nanostructured Lipid Carriers (NLC) for pulmonary application: a review of the state of the art. Eur J Pharm Biopharm. 2014;86:7–22.
- Nagpal K, Mohan A, Thakur S, et al. Dendritic platforms for biomimicry and biotechnological applications. Artif Cells Nanomed Biotechnol. 2018;1–15.
- Rajabnezhad S, Casettari L, Lam J, et al. Pulmonary delivery of rifampicin microspheres using lower generation polyamidoamine dendrimers as a carrier. Powder Technol. 2016;291:366–374.
- Bohr A, Water J, Beck-Broichsitter M, et al. Nanoembedded microparticles for stabilization and delivery of drug-loaded nanoparticles. Curr Pharm Des. 2015;21:5829–5844.
- Torge A, Grützmacher P, Mücklich F, et al. The influence of mannitol on morphology and disintegration of spray-dried nano-embedded microparticles. Eur J Pharm Sci. 2017;104:171–179.
- Goyal A, Garg T, Rath G, et al. Development and characterization of nano embedded microparticles for pulmonary delivery of antitubercular drugs against experimental tuberculosis. Mol Pharm. 2015;12:3839–3850.
- Gaspar D, Gaspar M, Eleutério C, et al. Microencapsulated solid lipid nanoparticles as a hybrid platform for pulmonary antibiotic delivery. Mol Pharm. 2017;14:2977–2990.
- Ohashi K, Kabasawa T, Ozeki T, et al. One-step preparation of rifampicin/poly(lactic-co-glycolic acid) nanoparticle-containing mannitol microspheres using a four-fluid nozzle spray drier for inhalation therapy of tuberculosis. J Control Release. 2009;135:19–24.
- Sharma V, Jain A, Soni V. Nano-aggregates: emerging delivery tools for tumor therapy. Crit Rev Ther Drug Carrier Syst. 2013;30:535–563.
- Kaur R, Garg T, Malik B, et al. Development and characterization of spray-dried porous nanoaggregates for pulmonary delivery of anti-tubercular drugs. Drug Deliv. 2016;23:882–887.
- Sung J, Padilla D, Garcia-Contreras L, et al. Formulation and pharmacokinetics of self-assembled rifampicin nanoparticle systems for pulmonary delivery. Pharm Res. 2009;26:1847–1855.
- Durham P, Zhang Y, German N, et al. Spray dried aerosol particles of pyrazinoic acid salts for tuberculosis therapy. Mol Pharm. 2015;12:2574–2581.
- Eedara B, Tucker I, Das S. Phospholipid-based pyrazinamide spray-dried inhalable powders for treating tuberculosis. Int J Pharm. 2016;506:174–183.
- Pham D, Grégoire N, Couet W, et al. Pulmonary delivery of pyrazinamide-loaded large porous particles. Eur J Pharm Biopharm. 2015;94:241–250.
- Kaewjan K, Srichana T. Nano spray-dried pyrazinamide-L-leucine dry powders, physical properties and feasibility used as dry powder aerosols. Pharm Dev Technol. 2016;21:68–75.
- Vadakkan M, Kumar G. Cryo-crystallization under a partial anti-solvent environment as a facile technology for dry powder inhalation development. RSC Adv. 2015;5:73020–73027.
- Parikh R, Dalwadi S. Preparation and characterization of controlled release poly-ε-caprolactone microparticles of isoniazid for drug delivery through pulmonary route. Powder Technol. 2014;264:158–165.
- Maretti E, Rossi T, Bondi M, et al. Inhaled Solid Lipid Microparticles to target alveolar macrophages for tuberculosis. Int J Pharm. 2014;462:74–82.
- Garcia Contreras L, Sung J, Ibrahim M, et al. Pharmacokinetics of inhaled rifampicin porous particles for tuberculosis treatment: insight into rifampicin absorption from the lungs of guinea pigs. Mol Pharm. 2015;12:2642–2650.
- Takeuchi I, Taniguchi Y, Tamura Y, et al. Effects of L-leucine on PLGA microparticles for pulmonary administration prepared using spray drying: fine particle fraction and phagocytotic ratio of alveolar macrophages. Colloids Surf A Physicochem Eng Asp. 2018;537:411–417.
- Brunaugh A, Jan S, Ferrati S, et al. Excipient-free pulmonary delivery and macrophage targeting of clofazimine via air jet micronization. Mol Pharm. 2017;14:4019–4031.
- Verma R, Germishuizen W, Motheo M, et al. Inhaled microparticles containing clofazimine are efficacious in treatment of experimental tuberculosis in mice. Antimicrob Agents Chemother. 2013;57:1050–1052.
- Garcia-Contreras L, Padilla-Carlin D, Sung J, et al. Pharmacokinetics of ethionamide delivered in spray-dried microparticles to the lungs of guinea pigs. J Pharm Sci. 2017;106:331–337.
- Parumasivam T, Ashhurst A, Nagalingam G, et al. Inhalation of respirable crystalline rifapentine particles induces pulmonary inflammation. Mol Pharm. 2017;14:328–335.
- Durham P, Young E, Braunstein M, et al. A dry powder combination of pyrazinoic acid and its n-propyl ester for aerosol administration to animals. Int J Pharm. 2016;514:384–391.
- Ibrahim M, Hatipoglu M, Garcia-Contreras L. SHetA2 dry powder aerosols for tuberculosis: formulation design and optimization using quality by design. Mol Pharm. 2018;15:300–313.
- Schoubben A, Giovagnoli S, Tiralti M, et al. Capreomycin inhalable powders prepared with an innovative spray-drying technique. Int J Pharm. 2014;469:132–139.
- Kulkarni V, Shaw C. Microscopy techniques. Essential chemistry for formulators of semisolid and liquid dosages. 1st ed. Amsterdam, Netherlands: Elsevier; 2016. p. 183–192.
- Pai R, Jain R, Bannalikar A, et al. Development and evaluation of chitosan microparticles based dry powder inhalation formulations of rifampicin and rifabutin. J Aerosol Med Pulm Drug Deliv. 2016;29:179–195.
- Onoshita T, Shimizu Y, Yamaya N, et al. The behavior of PLGA microspheres containing rifampicin in alveolar macrophages. Colloids Surf B Biointerfaces. 2010;76:151–157.
- Doan T, Couet W, Olivier J. Formulation and in vitro characterization of inhalable rifampicin-loaded PLGA microspheres for sustained lung delivery. Int J Pharm. 2011;414:112–117.
- Liu Z, Li X, Xiu B, et al. A novel and simple preparative method for uniform-sized PLGA microspheres: preliminary application in antitubercular drug delivery. Colloids Surf B Biointerfaces. 2016;145:679–687.
- Sethuraman V, Hickey A. Powder properties and their influence on dry powder inhaler delivery of an antitubercular drug. AAPS PharmSciTech. 2002;3:E28.
- Liu C, Kong C, Wu G, et al. Uniform and amorphous rifampicin microspheres obtained by freezing induced LLPS during lyophilization. Int J Pharm. 2015;495:500–507.
- Park J, Jin H, Kim D, et al. Chitosan microspheres as an alveolar macrophage delivery system of ofloxacin via pulmonary inhalation. Int J Pharm. 2013;441:562–569.
- Hwang S, Kim D, Chung S, et al. Delivery of ofloxacin to the lung and alveolar macrophages via hyaluronan microspheres for the treatment of tuberculosis. J Control Release. 2008;129:100–106.
- Parumasivam T, Leung S, Quan D, et al. Rifapentine-loaded PLGA microparticles for tuberculosis inhaled therapy: Preparation and in vitro aerosol characterization. Eur J Pharm Sci. 2016;88:1–11.
- Gupta A, Pant G, Mitra K, et al. Inhalable particles containing rapamycin for induction of autophagy in macrophages infected with Mycobacterium tuberculosis. Mol Pharm. 2014;11:1201–1207.
- Brooks B, Brooks A. Therapeutic strategies to combat antibiotic resistance. Adv Drug Deliv Rev. 2014;78:14–27.
- Weers J. Inhaled antimicrobial therapy - barriers to effective treatment. Adv Drug Deliv Rev. 2015;85:24–43.
- Kadota K, Senda A, Tagishi H, et al. Evaluation of highly branched cyclic dextrin in inhalable particles of combined antibiotics for the pulmonary delivery of anti-tuberculosis drugs. Int J Pharm. 2017;517:8–18.
- Chan J, Chan H, Prestidge C, et al. A novel dry powder inhalable formulation incorporating three first-line anti-tubercular antibiotics. Eur J Pharm Biopharm. 2013;83:285–292.
- Kumar Verma R, Mukker J, Singh R, et al. Partial biodistribution and pharmacokinetics of isoniazid and rifabutin following pulmonary delivery of inhalable microparticles to rhesus macaques. Mol Pharm. 2012;9:1011–1016.
- Momin MAM, Tucker I, Doyle C, et al. Manipulation of spray-drying conditions to develop dry powder particles with surfaces enriched in hydrophobic material to achieve high aerosolization of a hygroscopic drug. Int J Pharm. 2018;543:318–327.
- Momin MAM, Tucker I, Doyle C, et al. Co-spray drying of hygroscopic kanamycin with the hydrophobic drug rifampicin to improve the aerosolization of kanamycin powder for treating respiratory infections. Int J Pharm. 2018;541:26–36.
- Rodrigues S, Alves A, Cavaco J, et al. Dual antibiotherapy of tuberculosis mediated by inhalable locust bean gum microparticles. Int J Pharm. 2017;529:433–441.
- Lau J, Dunn M. Therapeutic peptides: historical perspectives, current development trends, and future directions. Bioorg Med Chem. 2018;26:2700–2707.
- Depreter F, Pilcer G, Amighi K. Inhaled proteins: challenges and perspectives. Int J Pharm. 2013;447:251–280.
- Chaulagain B, Jain A, Tiwari A, et al. Passive delivery of protein drugs through transdermal route. Artif Cells Nanomed Biotechnol. 2018;1–16.
- Mehta P, Pawar V. Electrospun nanofiber scaffolds: technology and applications. Applications of nanocomposite materials in drug delivery. Sawston, Cambridge: Woodhead Publishing Series in Biomaterials; 2018. p. 509–573.
- Kwok P, Grabarek A, Chow M, et al. Inhalable spray-dried formulation of D-LAK antimicrobial peptides targeting tuberculosis. Int J Pharm. 2015;491:367–374.
- Tyne A, Chan J, Shanahan E, et al. TLR2-targeted secreted proteins from Mycobacterium tuberculosis are protective as powdered pulmonary vaccines. Vaccine. 2013;31:4322–4329.
- Muralidharan P, Hayes D, Jr, Mansour H. Dry powder inhalers in COPD, lung inflammation and pulmonary infections. Expert Opin Drug Deliv. 2015;12:947–962.
- Mehta PP. Multi-dose dry powder inhaler: advance technology for drug delivery to airways. India Drugs. 2018. Available from: https://www.indiandrugsonline.org/accepted-article-details?id=MTE0MDM=
- Rogueda P, Traini D. The future of inhalers: how can we improve drug delivery in asthma and COPD? Expert Rev Respir Med. 2016;10:1041–1044.
- Mehta P, Pawar A, Mahadik K, et al. Emerging novel drug delivery strategies for bioactive flavonol fisetin in biomedicine. Biomed Pharmacother. 2018;106:1282–1291.
- Frick M. Funding for tuberculosis research-an urgent crisis of political will, human rights, and global solidarity. Int J Infect Dis. 2017;56:21–24.